DOI:
10.1039/C4RA03291H
(Review Article)
RSC Adv., 2014,
4, 28265-28299
Review on modified N–TiO2 for green energy applications under UV/visible light: selected results and reaction mechanisms
Received
11th April 2014
, Accepted 27th May 2014
First published on 28th May 2014
Abstract
Titanium dioxide photocatalyst has witnessed considerable interest for use in water and air cleanup owing to its fascinating properties like non-toxicity, ease of preparation, favorable band edge positions, water insolubility, multifaceted electronic properties, surface acid–base properties, and super hydrophilicity. Although it possesses much functionality, large bandgap and massive charge carrier recombination limits its wide utility under natural solar light. These drawbacks were overcome through nitrogen doping in titania matrix (N–TiO2), which alters the surface-bulk structure for visible light absorption with high quantum efficiency. In this review, we highlight the recent progress of N–TiO2 towards pollutant degradation, hydrogen evolution and its use in organic synthesis under ambient conditions. The preparation of N–TiO2 via different methods (physical and chemical methods) with diverse morphologies, nature of chemical dopants, induced defects and fundamental reaction parameters governing efficient photoinduced reactions are explored in this review. Further improvements in the photoefficiency of N–TiO2 were achieved through co-doping with foreign ions, heterostructuring with other semiconductors, metal deposition and the tuning of N–TiO2 with reactive exposed facets. The resultant improvement from each of the modification is discussed in the light of charge carrier generation–separation–transfer–recombination dynamics together with pollutant adsorption and their reactions with reactive oxygenated species in the liquid or gaseous regime. This review attempts to give an overview of the research highlights concerned with N–TiO2. Although it is impossible to cover all of the research articles in the literature, several milestones in the pathway towards highly applicable N–TiO2 are explored. It is hoped that this review article will trigger further research in synthesizing N–TiO2 with multifunctional features to enhance its capacity for green energy applications.
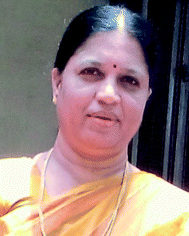 L. Gomathi Devi | Professor L. Gomathi Devi received her MSc degree (1981) from the University of Mysore and obtained her PhD in Chemistry from the Indian Institute of Science (1988), Bangalore, India. She is currently working as a senior professor in the Physical Chemistry section, Department of Chemistry, Bangalore University. Her research interest spans a broad spectrum of energy, environmental and material chemistry problems. She has authored more than 60 research articles in international peer reviewed journals and has authored several review articles and physical chemistry text books. |
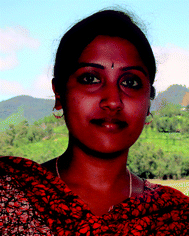 R. Kavitha | R. Kavitha has obtained her MSc degree in Physical Chemistry (2010) from the Department of Chemistry, Bangalore University. She is presently pursuing her PhD degree under the supervision of Professor L. Gomathi Devi. Her research work focuses on the preparation and characterization of nonmetal doped titania nanomaterials for energy and environmental applications. |
1 Introduction
Titania has shown great promise in several green applications, such as wastewater purification, catalyst in organic compound synthesis, gas sensors, photovoltaics and hydrogen generation, which can be ascribed to its favourable electronic band structure, biochemical compatibility, strong oxidizing power, non-toxicity and long-term stability against photochemical corrosion.1–10 However, TiO2 exhibits low photochemical quantum yield due to its relatively high recombination rate of photogenerated electron–hole pairs. In addition, the large bandgap of TiO2 absorbs only UV light, which accounts for merely 5% of solar photons, resulting in very low quantum yield in light to energy conversion. The abovementioned challenges serve as the impetus to engineer an environmentally benign and adsorptive-solar photocatalytic material by modifying the surface-electronic structure of TiO2 while retaining its advantageous catalytic properties.11–13 Numerous efforts, including crystal shape engineering, doping, co-doping with foreign ions, noble metal surface deposition and sensitization by inorganic complexes or organic dyes, hydrogen plasma reduction of TiO2, surface complexation, defect creation and multi-component heterostructuring, are frequently reported to display very high photocatalytic efficiency.14–30 Among the various approaches, nonmetal doping, is one of the promising techniques. In particular, nitrogen incorporation (N–TiO2) with different chemical entities into the titania lattice or on its surface is accepted to be beneficial for the improvement of photoefficiency of titania under UV/visible light.
The previously published review articles dealt with several fundamental aspects like synthesis, physical/chemical properties, role of nitrogen dopant, wavelength-dependent photoactivity and the synergism of electronically modified N–TiO2 with other materials that are aimed at enhancing TiO2 applications.11,13 Recently, our research group reported a comprehensive overview on nonmetal-doped TiO2, which focused on several factors like surface modification by noble metal deposition, co-doping with other foreign ions, organic dye sensitization and heterostructuring with other semiconductors, in addition to highlighting the correlations with photocatalytic reaction mechanisms.11 To date, reviews dedicated solely to N–TiO2 are rare in the literature; therefore, to fill this vacuum, we have discussed selected results of N–TiO2 that are mainly concerned with the preparation, structural modifications, morphology and synergistic effects of co-doping with other elements for the enhancement of photocatalytic activity to trigger research interest in this field.
2 Influence of preparative methods on the photocatalytic activity of N–TiO2
TiOHNx (x = 10 min is N2 plasma discharge time in min) obtained by the reduction–nitridation method via nonthermal plasma treatment was more favorable for methylene blue (MB) degradation under visible light compared to the samples prepared in other conditions like in the absence of N2 (TiOH) and H2 plasma treatment (TiONx).31 The above experimental condition promoted Ns doping in TiOHNx, which narrowed the bandgap efficiently when compared to simple nitridation treatment. Under visible light, electron is excited from Ns to the conduction band (CB), which then initiates superoxide radical production. The induced oxygen vacancies created by nitrogen dopants serve as trap sites for electrons at lower concentration, and they also facilitate recombination of electrons with the holes in Ns at high concentration.32,33 TiOHNx was found to be more stable in photocatalytic reactions compared to TiON10 for three consecutive runs, as the oxygen vacancies created by H2 plasma lead to the incorporation of nitrogen dopants deep into the TiO2 lattice (Table 1). When the lattice nitrogen on surface layer is oxidized by holes in the first run,34 a protective passivation layer is formed. This inhibits the further oxidation of nitrogen in TiO2 present in the deep layers, leading to stable lattice nitrogen content in subsequent cycles. In the case of TiON10, nitrogen is doped only into the surface layer, which can be easily oxidized by holes.31 The TiO2−xNx prepared by annealing TiO2 under NH3 flow decomposed gaseous 2-propanol at a faster rate under UV light compared to visible light. Note that UV light excites electrons from both valence band (VB) and dopant energy level, while visible light excites electrons only from the dopant level.35 The quantum yield for the degradation reaction decreased as the nitrogen content is increased in N–TiO2 (Fig. 1). During the process of annealing, oxygen sites were partially replaced with nitrogen atoms, which enhances the Ti3+ states, and oxygen vacancies below CB edge (0.75–1.18 eV) at higher dopant concentration, which promotes the recombination process.36 The N–TiO2 (N/Ti = 0.025 atomic ratio) prepared by sol–gel reverse micelle method with disodium ethylenediaminetetraacetate (Na2EDTA) as the nitrogen source showed remarkable activity for methyl orange (MO) degradation under visible light, which is attributed to the synergistic effects of nitrogen content, high crystallinity and large surface area of the sample.37 These above properties enable the catalyst to show higher extent of adsorption of oxygen/molecular water to react with photogenerated charge carriers to form oxygenated free radicals.37 The N–TiO2 calcined in atmosphere (200–250 °C, 0.5 h) displayed superior activity for MB decomposition under visible light compared to the sample sintered in N2 atmosphere (Fig. 2).38 In the former case, the number of active sites increases with the removal of organic residues on the surface, while in the latter case the number of such active sites will be lower as the organic residues cannot be oxidised. Note that the zeta potential of N–TiO2 was higher than TiO2, indicating that nitrogen doping and sintering induced more charges on the surface of these nanoparticles. Computational and ATIR results indicated that nitrogen doping occurs as a complexation between the central titanium metal ion and nitrogen atom.38 The preparation of N–TiO2 via the precipitation of [(TiO(C2O4)2)2−] in aqueous ammonia under alkaline conditions at low temperature, followed by calcination at 400 °C, exhibited better MO degradation under UV/visible light.39 This was attributed to the Bronsted acid sites created from covalently bonded dicarboxyl groups, which enhanced the capacity of N–TiO2 for MO adsorption (Fig. 3). The adsorption process in dark conditions revealed that N–TiO2 releases H+ ions when mixed with MO solution, reducing the solution pH and causes the catalyst surface to become more acidic.39 The N–TiO2 obtained by wet method using TiCl4 and NH4Cl as nitrogen source showed good performance for 4-nitrophenol degradation under UV/visible light compared to N–TiO2 catalyst prepared using TiOSO4 (NaOH) via wet and hydrothermal methods.40 The high photoactivity was due to the optimum anatase–rutile ratio,41–44 which allowed the vectorial transfer of charge carriers and retarded their recombination. Furthermore, bandgap narrowing,45,46 formation of oxygen vacancies and color centers also contributed to the activity in visible light.47 Contrarily, the presence of rutile phase was detrimental towards the degradation of MB and 4-cholrophenol (4-CP) using N–TiO2 calcined at > 500 °C, which was prepared by sol–gel method using 1,3-diaminopropane as nitrogen source.48 The VB of nitrogen-doped rutile TiO2 was lowered by 0.4 eV on the insertion of N 2p levels, which are lower in energy than pure rutile VB (0.05 eV). In this study, interstitial nitrogen served as mid bandgap states to render visible light response and also promoted anatase to rutile phase transition (ART).49 This suggests that titanium and nitrogen source, along with the preparative methods, play a vital role in altering the electronic properties of N–TiO2.
Table 1 Stability of lattice nitrogen in TiOHNx and TiON10 photocatalysts for its use in three repetitive cycles and the comparison of their activitiesa
Sample |
Fresh catalyst/at% |
First reuse/at% |
Second reuse/at% |
Third reuse/at% |
Reprinted with permission from ref. 31, Copyright (2011) Elsevier Publications. |
TiOHNx |
1.4 |
1.28 |
1.26 |
1.26 |
TiON10 |
0.32 |
0.24 |
0.20 |
0.18 |
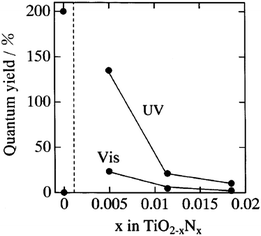 |
| Fig. 1 Quantum efficiency versus nitrogen dopant content (x) in TiO2 for the decomposition of gaseous 2-propanol under UV and visible light irradiation. Reprinted with permission from ref. 35, Copyrights (2003) American Chemical Society. | |
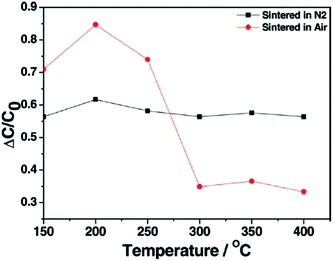 |
| Fig. 2 C/C0 versus temperature plot for N–TiO2 sintered at different temperatures (for 30 min) either in air or in N2 atmosphere. Photocatalytic activity was measured after 160 min of irradiation by a Xe arc lamp with wavelengths >400 nm. Reprinted with permission from ref. 38, Copyrights (2008) American Chemical Society. | |
 |
| Fig. 3 Mechanism of Bronsted acid site formations on the surface of N–TiO2 calcined at 400 °C (a schematic illustration). Reprinted with permission from ref. 39, Copyrights (2008) American Chemical Society. | |
The N–TiO2 thin films prepared by laser ablation method under N2 gas atmosphere of 200 Pa showed activity for MB degradation under UV/visible light. The reaction rate decreased for samples prepared at N2 pressure >200 Pa due to ART under low N2 pressure.50 XRD (X-ray diffraction) and XPS (X-ray photoelectron spectroscopy) provided information for a plausible mechanism for N–TiO2 by laser ablation. It was found that crystal structure transition from rutile to anatase occurs in the pressure range of 27 to 67 Pa. Moreover, it was observed that the nitrogen incorporation is easier near the critical transition point due to crystal instability. Furthermore, the molecular nitrogen becomes monoatomic and splits more easily under low N2 pressure compared to higher ones.51 In addition, N-TiO2 thin film turned yellow for the substrate at temperature >500 °C, suggesting that thermal energy originating from higher substrate temperatures is required for nitrogen doping, which otherwise does not occur in gas phase between the target and substrate.50 The nitrogen doping in the form of Ox–Ti–Ny and –(NO) obtained under O2/Ar plasma was active for isopropyl alcohol decomposition under UV/visible light, while nitrogen substitution in the form of –(NO2) under N2/O2/Ar plasma was less active compared to bare TiO2.52 The Ni–TiO2 (interstitially doped nitrogen) thin films synthesized by combinatorial atmospheric pressure chemical vapour deposition showed better MB and stearic acid degradation under UV and white light, respectively, than Ns–TiO2 due to instantaneous charge carrier recombination processes in Ns–TiO2.53 The N–TiO2 films fabricated by annealing TiO2 films in gaseous NH3 showed lower quantum yields for gaseous propanol decomposition and high decomposition rates for aqueous ethylamine ions (CH3CH2NH3+) as positively charged cationic ethylamine ions could adsorb efficiently on the negatively charged N–TiO2 surface.54 The zeta potential evaluation of N–TiO2 revealed that surface is negatively charged with an increase in the concentration of nitrogen dopant (Fig. 4). However, quantum yield values under visible light were much lower compared to studies under UV light, although the number of absorbed photons were identical under both excitation sources. This suggests that the holes produced by visible light were localized at isolated levels between VB and CB and were unable to diffuse to the upper edge of VB due to inefficient mixing of N 2p levels with O 2p orbital.54
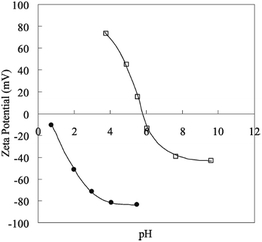 |
| Fig. 4 Zeta potential versus pH value for the thin films, open square: pure TiO2, closed circle: N–TiO2 (annealed in NH3 at 600 °C). Reprinted with permission from ref. 54, Copyrights (2004) Royal Society of Chemistry. | |
The N–TiO2 prepared by the heat treatment of nanotubic titanic acid (NTA) in ammonia solution and gaseous ammonia flow possessed better activity for organic pollutant degradation under visible light.55,56 This was attributed to the single electron trapped oxygen vacancy (SETOV), electron trapping sites, interstitial nitrogen doping (Ni) and also the formation
–NO–Ti hot centers as shown in the following equations.57
|
 | (1) |
|
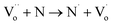 | (2) |
On illumination, electron transfer takes place from VB to CB, and then they are trapped by SETOV, which prevents instant recombination. Moreover, nitrogen atom in Ti–O–N in interstitial site transfers the electron cloud towards oxygen atom and gains high chemical valence by entrapping another electron from SETOV. This interstitial nitrogen atom with an excess electron is unstable and immediately detraps the electron to oxygen (Fig. 5).58 However, undoped TiO2 did not show visible light photocatalytic activity despite SETOV formation due to the absence of nitrogen dopant.56
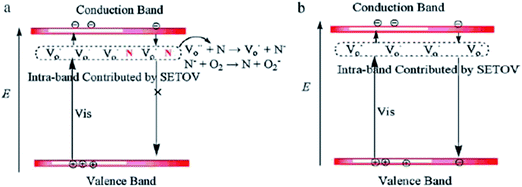 |
| Fig. 5 Schematic illustrations of the role of nitrogen dopant in preventing photogenerated charge carrier recombination (a) for N–TiO2 with enhanced visible light absorption and photocatalytic activity (b) for novel TiO2 with enhanced visible light absorption without photocatalytic activity. Reprinted with permission from ref. 59, Copyrights (2004) Elsevier Publications. | |
The N–TiO2 obtained by mixing TiO2 with 0.5 M guanidine carbonate as nitrogen source exhibited better activity for 2-propanol decomposition in visible light than the N–TiO2 samples prepared using urea and guanidine hydrochloride.59 The N–TiO2 synthesized at 160 °C by hydrothermal treatment showed superior MO degradation under visible light compared to N–TiO2 samples prepared at 120 °C by the same method.60 The visible light activity of N–TiO2 increases with the decrease in pH (3–10) in the preparative process as the nitrogen concentration strongly depends on pH of the reaction medium.60 The electron spin resonance spectra (ESR) revealed that anatase N–TiO2 with Nb˙ centers was active for formic acid degradation, while rutile N–TiO2 with paramagnetic Ti3+ centers had very low activity.61,62
3 N–TiO2 with hierarchical morphologies
To resolve the problem of low quantum efficiency, bulk diffusion and effective surface charge transfer, along with maximum light harvestation, are the important key factors. It is believed that complex hierarchical morphologies would offer new opportunities in integrating several fascinating properties like increased surface area, high porosity, low bulk density, enhanced photonic efficiency and dispersity in reaction solution together with structural and catalytic stability with a high extent of charge carrier separation.63,64 These properties originate from geometrical structure, good mechanical strength, multiple scattering, and better access of reactants to the catalyst together with high light harvesting efficiencies.65
The amorphous N–TiO2 nanotubes exhibited activity for rhodamine B (RhB) and MB degradation under visible light compared to crystalline N–TiO2 and DP25, indicating that high nitrogen content, high surface area and large pore volume played a crucial role in promoting the activity.66,67 The loss of nitrogen content,67 destruction of tubular structure, reduced specific surface area and oxygen vacancy formation became detrimental after calcining the catalyst >350 °C.66 The CO2 was reduced to HCOOH, HCHO and CH3OH effectively on N–TiO2 nanotube under visible light compared to unmodified TiO2 nanotube.68 The energy state of substituted nitrogen (Ns) was found on VB edge, while the energy state of Ni appeared in the forbidden TiO2 bandgap, thus altering its electronic structure.69,70 In addition, increased surface area favored more CO2 adsorption on the catalyst surface.68 Note that the oxidation of CO failed with N–TiO2 nanotube, but promoted the reduction of resazurin to resofurin under visible light, which was a kinetically favored reaction.71 The high degree of recombination induced by N 2p state hampered the activity under UV light, as oxygen reduction is a four-electron reaction with tedious kinetics and high overpotential.71 The N–TiO2 nanotubes synthesized via solvothermal treatment method heated at 120 °C for 5 h using protonated titanate nanotube in an NH4Cl–ethanol–water solution, followed by calcination at 450 °C, showed a better performance for MB degradation under visible light compared to protonated titanate nanotube.72 The presence of chloride ion in the synthesis played a key role in transition from titanate to N-TiO2 at low temperature. This fact is revealed by the use of various nitrogen sources like NH3, (NH4)2CO3, NH2CONH2 and NH4NO3 where the conversion of titanate to N-TiO2 was inefficient.72 The N–TiO2 mesosponge fabricated by the transformation of TiO2 nanotube arrays in ethanolic NH3 exhibited high photocurrent densities and MO degradation under UV light, which was attributed to the excellent degree of crystallinity and the efficient utilization of UV light by N–TiO2 as a result of localized N 2p level above TiO2 VB, which narrowed the bandgap and efficiently promoted charge carrier separation.73 The stable and reusable N–TiO2 nanotube array films fabricated by electrochemical anodization, followed by wet immersion in aqueous NH3, and then calcination at 450 °C for 2 h decomposed MO efficiently under UV/visible light.74 The XRD patterns revealed that bare TiO2 was composed of anatase and rutile phases, while nitrogen doping via O–Ti–N surface state suppressed ART process owing to the strong crystal distortion force.75,76 In addition, the presence of porous nanotube structure and nitrogen dopant inhibited the migration and rearrangement of titanium and oxygen atoms to form rutile phase during the calcination process.77,78 The electrochemical impedance spectroscopy (EIS) spectra showed a smaller impedance arc radius for N–TiO2 under UV illumination, demonstrating that N–TiO2 had a greater charge carrier separation efficiency and fast charge transfer than pure TiO2 at solid–liquid interface.74
The N–TiO2 hollow sphere was more active for bisphenol A (BPA) degradation and mineralization under blue, green and yellow lights compared to undoped TiO2 hollow spheres and Hombikat UV100 (Table 2).79 The degradation rate monotonically increased with the increase in pH, and the rate was highest at pH ∼ 9.6 (pKa of BPA ∼1), which can be attributed to the increased hydroxyl radical concentration. The high surface area, high porosity and low bulk density enhanced the photonic efficiency and dispersity in reaction solution.79 In another study, activity of N–TiO2 hollow spheres synthesized by co-precipitation and template elimination method and spherical N–TiO2 showed similar performance for dichlorophenol (DCP) degradation, indicating that photocatalytic reaction does not occur on the inner surface of the hollow sphere but occurs only on the outer surface of the hollow sphere as sufficient light cannot reach the inner surface.80 The photoanode consisting of multilayer films of Ti0.91O2−xNx nanosheets showed an increase in photocurrent compared to undoped nanosheets under visible light irradiation, which was mainly attributed to the photoexcitation of oxygen vacancy related states induced by nitrogen doping.81 These nanosheets were prepared by solid state proton exchange of Cs0.68Ti1.83O4 with H0.68Ti1.83O4, which was followed by subsequent exfoliation process.82 The nitrogen doping was carried out using solid state reaction step with gaseous NH3. The interlayer galleries of layered Cs0.68Ti1.83O4 precursor provided excellent channels for nitrogen diffusion over layered titanate particles.83 The N–TiO2 was obtained from these titanates via ion exchange process in HCl solution (1 mol dm−3), followed by a subsequent exfoliation process in tetrabutylammonium (TBAH) solution (Fig. 6).81 The mesoporous N–TiO2 prepared by exfoliation–reassembling strategy with ethylamine as the nitrogen source and a delaminating agent for layered titanate showed exceptional activity for MO and 4-aminobenzoic acid degradation under visible light compared with P25, pristine protonic titanate and N–TiO2 fabricated from template-free route.84 This high activity is ascribed to high porosity, enhanced visible light absorption, increased surface area and efficient charge carrier separation. The mesopore size of 5 nm was large enough for easy access for organic molecules to the catalyst surface. Due to structural similarity, both TiO6 octahedra and titanate nanosheets were modified by nitrogen species,85,86 which introduced localized states in both TiO2 and titanate nanosheets, enabling visible light absorption and bandgap narrowing.87,88 The charge carrier transfer was improved due to the presence of straddling gap in energy potentials between TiO2 and titanate nanosheets. The MO degradation was hardly suppressed with the addition of tertiary butanol and was completely inhibited in the presence of ethylenediaminetetraacetate (EDTA), suggesting the dominant role of holes and negligible contribution from the sensitization process. Transmission electron microscope (TEM) images and XRD patterns revealed that the random reassembly between titanate nanosheets and TiO2 gave rise to its porous structure.84 The enhancement in hydrogen evolution under visible light irradiation with 10 vol% methanol by fibrous hierarchical meso-macroporous N–TiO2 was attributed to its porous network with uniform nitrogen dispersion throughout the catalyst.89 The hierarchical and fibrous structure allows channelization of electrons for effective surface charge transfer process (Fig. 7). The presence of macroporous channel within the catalyst enhances the efficiency of photoabsorption and improves the mass transfer process within the macro-mesoporous frame. The macrochannel acts as a light transferring path, which introduces incident photon flux into the inner surface, and this light activated surface area increases as the process absorption, reflection and scattering takes place within the material.90 With an increase in calcination temperature to >400 °C, the destruction of mesoporosity and loss of nitrogen content leads to poor activity.89 The N–TiO2 with morphologies of nano-octohedra, nanoparticles and nanosphericals were successfully synthesized through the nitrification of TiO2 in the presence of NH3 (700 °C, 30 min) as depicted below:91
Table 2 Pseudo-first-order rate constant (k′) and regression coefficient (R2) values for various photocatalysts under different light sources for the photocatalytic degradation of BPAa,b
Sample |
Blue LED |
Green LED |
Yellow LED |
k′ (h−1) |
R2 |
k′ (h−1) |
R2 |
k′ (h−1) |
R2 |
Within the initial 3 h of reaction. Reprinted with permission from ref. 79, Copyrights (2010) Elsevier Publications. |
TiO2 powder (pH 6.0) |
0.238 |
0.996 |
0.034 |
0.931 |
0.034 |
0.932 |
TiO2 hollow sphere (pH 6.0) |
0.353 |
0.977 |
0.048 |
0.981 |
0.040 |
0.978 |
N–TiO2 hollow sphere (pH 6.0) |
1.131a |
0.989 |
0.148 |
0.976 |
0.076 |
0.969 |
N–TiO2 hollow sphere (pH 3.0) |
0.507a |
0.971 |
— |
— |
— |
— |
N–TiO2 hollow sphere (pH 10) |
1.342a |
0.971 |
— |
— |
— |
— |
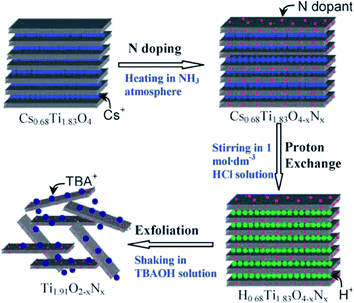 |
| Fig. 6 Schematic procedure for the preparation of nitrogen-doped Ti1.91O2−xNx nanosheets starting from Cs0.68Ti1.83O4. TBA+: tetrabutylammonium ion. Reprinted with permission from ref. 81, Copyrights (2009) Royal Society of Chemistry. | |
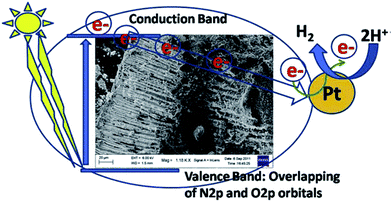 |
| Fig. 7 Illustration of the mechanism of hydrogen production from fibrous hierarchical meso-macroporous N–TiO2 under visible light irradiation. Reprinted with permission from ref. 89, Copyrights (2013) Elsevier Publications. | |
The N–TiO2 nano-octahedra exhibited the best activity for MO degradation compared to other N–TiO2 structures under UV/visible light irradiation, attributed to the impurity state above the VB by nitrogen dopant, which also improved the TiO2 conductivity and inhibited recombination rates. In addition, well exposed {101} facet on crystallized surface serves as possible reservoirs for electrons to facilitate the production of superoxide radicals.91 The N–TiO2 with rice grain-like structure prepared by the electrospinning method, followed by annealing at 450 °C for 1 h, showed superior MB degradation and hydrogen evolution compared to TiO2 rice grains, which was attributed to the nitrogen dopant with rice grain nanostructure.92 The rutile N–TiO2 with V-shaped nanorods synthesized by hydrothermal method using HCl and TiN showed an enhanced visible light absorption and a red shift in bandgap absorption.93 The separation of nitrogen ions from TiN provided a route for self-doping in the formation of nanorods with HCl. The chemical reactions involved in the transformation from TiN to TiO2 are represented as follows:94
|
Ti3+ + H2O → Ti(OH)2 + H+
| (6) |
|
Ti(OH)2 + O2 → Ti(IV)oxo species
| (7) |
|
Ti(IV)oxo species + N species → N–TiO2
| (8) |
The Ti(IV)oxo species is an intermediate between TiO2+ and TiO2, consisting of partly dehydrated polymeric Ti(IV) hydroxide.95,96 The Ti(OH)2 was derived from the precursor solution (Ti3+N3−) and O2 from autoclave or the reaction solution. There were two types of V-shaped nanorods with inner angles of 114.4° and 134.9° with the same coherent boundary on the {101} plane. Oriented attachment (OA) and Ostwald ripening (OR) processes lead to the formation of straight and V-shaped N–TiO2 nanorods (Fig. 8). The small V-shaped nanocrystal is formed via OA mechanism by attaching two similar grains, in which two {101} high energy planes join together and are eliminated, and it finally follows the OR coarsening process. The formation of V-shaped nanocrystal is essentially a facet-mediated aggregation and there is sequential elimination of high energy surfaces owing to the significant contribution of surface energy to the total free energy in a nanoscale system.93 The V-shaped structures are of particular interest because the sudden break down in lattice periodicity at the junction offers a good lateral confinement; thus, they can enhance the excitonic optical response.97 The dual structure of rutile N–TiO2 nanoflower film aggregates sitting on top of the anatase layer showed superior activity for RhB degradation under visible light compared to the activity of titania nanorod film and titania derived from the P25 slurry.98 The activity was ascribed to its unique morphology of top layer, which possessed a high specific surface area of 77.8 m2 g−1.98 These N–TiO2 nanoflower films were fabricated by oxidizing metallic Ti plates with H2O2-containing hexamethylene tetraamine (HMT) and concentrated nitric acid at 353 K for 72 h. The low-temperature growth of flower like rutile TiO2 on the top layer occurred only after 60 h when the reaction solution contained almost no hydrogen peroxide or Ti(IV) ions.98 In thermodynamics, two factors were believed to contribute to the low-temperature growth of rutile nanoflower, i.e., additives like HMT and nitric acid, and the porous hydrated titania layer formed in the first 60 h of the reaction. At this stage, a porous hydrated amorphous titania layer was deposited onto the titanium substrates, on which a top layer of rutile nanoflower precipitated due to a supersaturated Ti(IV) solution. With prolonged reaction time of up to 72 h, small rutile nanorods precipitated from the solution, which aligned to form bundles and eventually fused through an OA mechanism to achieve a larger nanorod. The large nanorods self-assembled to a flower-like morphology with the assistance of structure-directing agents like NH3 and NO3−, which were produced by the decomposition of HMT:99,100
|
(C6H2)6N4 + 6H2O + H+ → 6HCHO− + 4NH+4
| (9) |
 |
| Fig. 8 The mechanism of formation of straight and V-shaped N–TiO2 nanorods starting from TiN. Reprinted with permission from ref. 93, Copyrights (2012) Royal Chemical Society. | |
These ions also helped in the synchronal incorporation of nitrogen into the titania matrix; however, a red shift for the light response of the titania nanoflower film was not observed.98
4 Co-doping
There exist unfavorable factors such as oxygen vacancy and Ti3+ defects due to charge imbalance induced by nitrogen, which may serve as recombination centers. However, co-doping maintains the charge balance through charge compensation, creates new electronic levels, delays recombination of charge carriers and further shifts the absorption edge to visible region. The probability of charge carriers to reach catalyst surface before recombination is higher for co-doped TiO2 compared to single ion-doped and undoped counterparts. The activity of co-doped titania is largely determined by the chemical nature of the dopants and the bonds between them.101–104
4.1 Incorporation of nitrogen into TiO2 with other nonmetal dopants
Nitrogen and boron codoped TiO2 (N–B–TiO2) with 10 mol% dopant exhibited high activity for 4-CP degradation under UV/visible light compared to single-doped titania.105 XPS and XRD gave evidence for the coexistence of interstitial nitrogen, [NOB] species in bulk and NOx species, and B2O3 on TiO2 surface when the boron content is high. The interstitial B3+ ion was sp2-hybridized with lattice oxygen forming a planar structure while lattice oxygen atoms linked with one boron and two titanium atoms to form Ti–O–B–O–Ti structure,106–109 which shifted the bandgap absorption to the visible region. Although the [NOB]˙ species do not directly contribute to the visible light response, trapped CB electrons will generate the [NOB−] diamagnetic center, which inhibits the recombination reaction pathways.110 Under UV light, electrons are excited from both TiO2 VB and [NOB]˙ species to TiO2 CB, which in turn are transferred to B2O5 CB.111,112 The simultaneous hole transfer from VB to surface state energy level of NOx species leads to the direct oxidization of surface adsorbed 4-CP.113 On the other hand, the electron could transfer from dopant-induced electronic state to CB under visible light illumination. Moreover, the electrons residing at B2O3 CB are trapped by adsorbed oxygens to generate superoxide radicals.114 The N–B–TiO2 (red anatase TiO2) prepared by heating anatase TiO2 microspheres (with a predoped interstitial boron shell) between 580 and 620 °C in a gaseous NH3 atmosphere with a flux of 50 mL min−1 for 60 min exhibited high photoelectrochemical water splitting activity under visible light.115 The UV/visible absorption spectra of red anatase TiO2 shows an extended absorption edge up to 700 nm, covering the full visible light spectrum due to a bandgap gradient, which varies from 1.94 eV on the surface to 3.22 eV in the core by gradually elevating the VB maximum (Fig. 9). Raman studies showed an additional active mode in the range of 450 to 500 cm−1 for red TiO2, attributed to the substitutional nitrogen dopant in the TiO2 bulk, which breaks down the Raman selection rules to generate a new active mode by lowering the geometric symmetries of TiO2.116 This new doping approach by a predoped interstitial boron gradient improved the solubility of substitutional nitrogen in TiO2 bulk without introducing the Ti3+ impurity level. The interstitial boron dopant effectively weakened the surrounding Ti–O bonds to facilitate easier nitrogen substitution and increased the chemical stability of codoped TiO2. Moreover, the extra electrons from boron dopant compensated for the charge difference between the lattice O2− and substitutional N3− ion to maintain charge neutrality.116
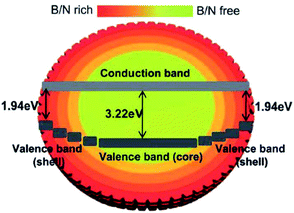 |
| Fig. 9 Schematic of the band structure of boron and nitrogen-doped red TiO2 depicting band gap gradient. Reprinted with permission from ref. 115, Copyrights (2012) Royal Chemical Society. | |
The nitrogen and carbon codoped TiO2 (N–C–TiO2) showed high activity for MB decomposition under visible light due to the synergistic effect between carbon and nitrogen co-doping.117 The degradation process followed two pathways: (i) reactive CB electrons reduce surface adsorbed oxygen to its radical anion, which transforms into H2O2 and hydroxyl radical; (ii) reactive holes oxidize MB to its radical cation through hydroxyl radical produced by the oxidation of water. During the course of interfacial electron transfer, adsorbed carbonaceous species gets excited and injects electrons into TiO2 CB, thereby increasing the kinetics of dioxygen reduction. The nitrogen dopant creates an intra bandgap state close to VB and also shifts the position of flat band potential to a higher level than undoped TiO2, thus increases the interfacial electron transfer kinetics. The ion–dipole interaction between TBA+ (carbon source) and water molecules hinders the TBA diffusion into the TiO2 bulk, indicating that carbon atoms are difficult to weave into TiO2. This leads to TBA segregation on the TiO2 surface inhibiting the crystal growth. Moreover, the adsorption experiments indicated that surface carbonaceous species degraded more number of MB molecules and transferred them to residual vacancies through surface diffusion, which is faster than free diffusion in solution, thus enhancing its degradation rate.117 The hydrothermal synthesis of N–C–TiO2 nanotubes exhibited 2.3 times higher sonocatalytic activity for RhB degradation, which was ascribed to various factors like co-doping, high surface area, lower bandgap energy and the creation of surface vacancies as a result of Ti3+ formation.118 XPS results indicated that interstitial nitrogen atoms were bonded to the oxygen atoms via Ti–O–N and Ti–N–O and substituted nitrogen atoms at oxygen sites via N–Ti–O bond, and the carbon dopant via Ti–C bond and as complex carbonate species on the catalyst surface. Sonoluminescence technique produced light flash with an average photon energy of 6 eV. Under these conditions, both surface adsorbed carbonaceous species and RhB serve as sonosentizers injecting electrons into TiO2 CB,119–121 resulting in the reduction of Ti4+ to Ti3+ state, which is localized at 0.75–1.18 eV below TiO2 CB. These reactive sites facilitate oxygen adsorption on the TiO2 surface.118–123 In addition, water molecules are also decomposed under acoustic cavitation to release active hydroxyl and hydrogen radical species, contributing to the overall performance.118
|
N–C–TiO2 + ))) → e− + h+, ))) = ultrasonic irradiation
| (10) |
|
2HOO˙ + H2O → H2O2 + OH˙
| (17) |
|
H2O + N–C–TiO2 + ))) → OH˙ + H+
| (19) |
The N–F–TiO2 under optimized conditions (catalyst dosage = 600 mg L−1, ratio of Cr(VI)/Benzoic acid (BA) = 5 and pH = 4) initiated a simultaneous redox reaction: the reduction of Cr(VI) ions and the oxidation of BA under visible light.124 In this binary system, coupled oxidation of BA consumes holes or hydroxyl radicals, while Cr(VI) ions serve as electron acceptors. The electrons in states below N–F–TiO2 CB have a weaker reduction ability, which can preferably reduce strong oxidizing agents like Cr(VI), and therefore enhance the target reduction reaction without competition from other ineffective reduction reactions.124 The mesoporous N–F–TiO2 performed better for acid orange (AO7) degradation under visible light due to large surface area and surface acidity, which promoted the increased adsorption of organic pollutants on catalyst surface.125 In addition, fluorine doping suppressed the anatase to rutile phase transformation process, enhanced the formation of oxygen vacancies and facilitated the generation of highly oxidative free hydroxyl radicals in solution.126,127 The N–F–TiO2 nanobelts prepared by a solvothermal method employing amorphous titania microspheres showed pronounced activity for MO degradation under UV/visible light, which is ascribed to the following factors:128 (i) enhanced visible light absorption and scattering;129–131 (ii) rapid transport of diffusion-free electron along the longest direction;132,133 (iii) high degree of crystallinity with minimum grain boundaries;129–131 (iv) smaller pore size and larger surface area. The fluorine doping leads to the formation of several new active sites and generates more number of hydroxyl radicals. The photocatalytic test measurement via the new testing method of scotch tape-covered N–F–TiO2 nanobelt further enhanced its activity as more photons can be captured by the nanobelts to simulate charge carrier generation compared to conventional methods. The BET results revealed the presence of large amounts of wormhole like mesopores which act as prisons and it is not easy for the photons to escape from these prisons, leading to the collision of photons with nanobelts for the exchange of energy.128
N–Si–TiO2 hollow microspheres obtained by facile aerosol flow method showed good performance for salicylic acid degradation under visible light due to the cooperative effects of nitrogen dopant and Ti–O–Si bond.134 The aerosol-assisted flow synthesis process was initiated by homogeneous aqueous solution containing soluble precursors when the droplets passing through tubular furnace carried by an air stream reduced the temperature of the droplets from the outer to interior layer.109 The solvent evaporated quickly and precursor ions assembled in the interior layer of droplets via a diffusion process (Fig. 10). Simultaneous generation of NH3 and HF from the decomposition of NH4F played a vital role in the formation of hollow structured particles. Further treatment with HF solution led to the formation of microspheres with macroscopic pores, which increased the specific surface area of the catalyst.134 The N–P–TiO2 (0.05 P to Ti ratio) showed a remarkable visible light activity for MB and RhB decomposition rather than N–TiO2 and N–S–TiO2.135 The nitrogen doping resulted in the formation of O–Ti–N linkage, while the dopant phosphorous existed either in the pentavalent oxidation state (P5+) or as a PO43− group coordinated to TiO2. The oxygen vacancies formed due to this coordination promoted electron and hole transfer to the surface, thereby reducing the charge recombination.136,56
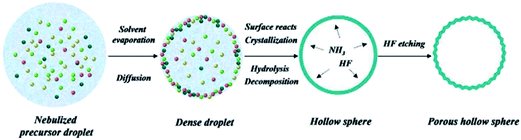 |
| Fig. 10 Illustration of various multistage process involved in the formation of N–Si–TiO2 porous hollow microsphere. Reprinted with permission from ref. 134, Copyrights (2012) Elsevier Publications. | |
The cooperative effects of visible light response and high charge carrier lifetime contributed to the excellent performance of mesoporous N–S–TiO2 (B) nanobelts (thiourea to Ti molar ratio [R] = 3) for potassium ethyl xanthate degradation under visible light.137 With a further increase in the R value to 4, the activity decreased as the dopants sites acted as recombination centers.137 The mixed phase (62% anatase + 38% rutile) N–S–TiO2 modified by thiourea exhibited superior activity for MB degradation under visible light.138 The Fourier transform infrared spectroscopy (FTIR) and XPS studies showed the formation of a Ti4+–thiourea complex, S6+ doping and the existence of nitrogen as lattice (N–Ti–N) and interstitial (Ti–N–O) species in the heterojunction. The isolated S 3p, N 2p and π* N–O states between VB and CB narrowed the bandgap, thereby rendering visible light response.139,140 The increased surface acidity of TiO2 due to the electron-withdrawing inductive effect of sulphate ions and effective charge transfer in biphasic anatase–rutile coupled system increased the activity.141,49 In another study, N–S–TiO2–montmorillonite was synthesized by impregnating doped titania sol into the interlayers of montmorillonite, followed by calcination at 350 °C, showed a better performance for acid red decomposition under UV light than undoped TiO2–montmorillonite, which is attributed to its large surface area, pore volume, crystallinity and the high number of hydroxyl radical species on the surface.142 Furthermore, electron-deficient nitrogen atom in O–Ti–N states trapped the CB electron and prevented the charge carrier recombination.143 The N–I–TiO2 having 1
:
5 Ti
:
N/I molar ratio showed simultaneous reduction of Cr(VI) and BA oxidation under visible light irradiation similar to N–F–TiO2 as reported earlier.144 The electron paramagnetic resonance (EPR) data suggests that Nb˙ species narrows the bandgap and facilitates the formation of Ti3+ surface ion, which plays a key role in capturing gaseous oxygen and reduction–oxidation process. The light absorption ability of co-doped titania was proportional to the concentration of Nb˙ species insertion, and it was found deeper in TiO2 lattice with lower Eg values. The doping of I5+ promotes the formation of photoinduced surface Ti3+ electrons, which react with adsorbed Cr(VI) and O2 to form radicals such as O2˙−, HO2˙−and OH˙. In addition, EPR experiments showed the stabilization of oxygen trapped holes TiO4+–O˙−. This was created by the reaction of anatase holes with lattice oxygen O2− and its concentration was inversely correlated with the oxidation rates.144 The hierarchical macro-/meso-porous N–TiO2, N–S–TiO2 and N–F–TiO2 prepared by hydrothermal method without calcination did not show any visible light activity for RhB degradation, since the non-metal ions existed as surface impurities before calcinations. However, this thermally calcined N–TiO2, N–S–TiO2 and N–F–TiO2 were active for RhB degradation under the same experimental conditions.145 Thermal calcination effectively increases nitrogen implantation into TiO2 lattice and causes a red shift in optical absorption. However, sulphur and fluoride ions resided mainly on the surface. The visible light activity was enhanced when the catalyst was stored for six months in desiccators or by stirring in water for 1 h, which facilitates the electrostatic adsorption of water molecules with surface hydroxyl groups on the catalyst surface. However, water-treated N–TiO2 and N–S–TiO2 were not more active than TiO2 calcined at 400 °C due to the presence of nitrogen and sulphur residues on the surface, which disturbed the bonding of water and subsequent water-mediated adsorption switching process. Interestingly, water-treated N–F–TiO2 showed a high activity despite the presence of nitrogen and fluoride species on the surface, which can induce the formation of electrostatic adsorption with RhB.146,147
4.2 Incorporation of nitrogen along with other metal dopants into titania lattice
The co-doping of nitrogen with other metals ions improves the photoactivity of titania, in which metal ions with changing valence serve as carrier reactive trapping sites and passivate the defect bands produced by monodoping.148–150 In general, metal ion dopants form energy level below CB edge and anionic dopants of nonmetals generate energy levels immediately above the TiO2 VB, effectively narrowing the bandgap, which enables the utilization of a large fraction of the solar spectrum.150
4.2.1 Incorporation of nitrogen along with 3d block metal ions into titania lattice. The co-doping of nitrogen and vanadium in TiO2 lattice (N–V–TiO2) showed activity for RhB degradation under visible light, and the activity is attributed to optimal dopant concentration with enhanced light absorption ability and efficient charge carrier separation.151 In addition, N–V–TiO2 with small crystallite size with a good degree of dispersion provided high surface to volume ratio, thereby increasing the contact area between active sites and reactants. Due to the strong V–N covalent bond, V 3d states lowers the N 2p energy levels, bringing them closer to VB, and thereby enhances the mixing of N 2p and O 2p states, which produces favorable conditions for trapping holes.152 On the contrary, the position of V 3d states are shifted to low energy regions, therefore diminishing any possible overlap between V 3d and Ti 3d due to the bonding interaction between the V 3d and N 2p orbitals. The presence of V5+ ions in the form of V2O5 (Eg = 2 eV) produces a space charge layer at the TiO2 interface due to the difference in electrochemical potential,153 which acts as driving force for CB electron to be injected instantaneously into the V5+ species.154 The codoping of Mn and nitrogen (Mn–N–TiO2) stabilized the anatase phase and also performed better RhB degradation under visible light,155 while doping with only Mn was reported to promote rutile formation even at moderate temperatures.156–162 In the case of Fe–N–TiO2, the synergy of a higher degree of crystallization and pronounced mesoporosity with narrow pore-size distribution resulted in phenol and MO degradation under visible light.163,164 The high degree of crystallization and small crystallite size facilitated rapid electron transfer from bulk to surface effectively inhibited carrier recombination,32 while ordered mesoporous channels facilitated the diffusion and adsorption of reactant molecules for efficient degradation process.164,165 The iron atoms distributed on TiO2 surface in the form of Fe2O3 suppressed the recombination and increased the quantum efficiency.166 The N–Zn–TiO2 (1 atom% Zn) displayed excellent activity for MB degradation under visible light due to the oxygen vacancies induced by zinc doping and the formation of N 2p states above VB edge.167 The co-doping of zinc into N–TiO2 shifted the bandgap absorption to the lower wavelength region due to the formation of oxygen vacancies and the Ti3+ state in TiO2.168,169 The PL spectra indicated that zinc doping suppressed the free exciton recombination emission due to electron trapping by oxygen vacancies and these trapped electrons further recombined with holes to increase the low energy PL intensity. However, nitrogen dopant can hold back the recombination of trapped electron in oxygen vacancy in TiO2 with holes by N 2p states above VB maximum. It has been stated that the surface oxygen vacancy interacts strongly with exoteric oxygen molecules to form O2 (efficient electron scavenger), which traps electrons to form O− crucial for oxidation process.169–171
4.2.2 Incorporation of nitrogen along with 4d block metal ions into titania lattice. The photoactivity of N–Y–TiO2 towards MB degradation in visible light was ascribed to charge trapping and photocatalytic active centers induced by yttrium doping.172 However, the high content of yttrium dopant served as recombination centers thus reducing the activity. The ionic radius of Y3+ (0.089 nm), which is higher than Ti4+ (0.068 nm), expanded the TiO2 lattice on Y3+ substitution.172 The N–Zr–TiO2 synthesized via modified sol–gel route using supercritical CO2 decomposed MB under visible light, which was ascribed to several factors:173 (i) high surface area with mesoporous structure favoring adsorption of reactant molecules; (ii) reduction in crystallite size, which provided higher interfacial area and access to active sites; and (iii) defects induced by nitrogen dopant captured more electrons and reduced the recombination.174–177 The nitrogen-doped Ti1−xZrxOx solid solutions obtained by hydrothermal method exhibited a much higher activity for acid red 88 and benzene degradation under visible light irradiation compared to N–TiO2, which was attributed to the synergistic effect of nitrogen and zirconium dual-modification.178 XPS and DRS studies revealed that nitrogen was mainly doped on the surface layer of catalyst and induced a surface state close to VB. On the other hand, lattice Ti4+ ions were substituted by Zr4+ ions, resulting in the elevation of CB thus facilitating electron transfer and separation of charge carriers.178 In N–TiO2 solid solutions, electrons are excited from surface state to CB and subsequently transferred to adsorbed surface species. In comparison to N–TiO2, the elevation of CB in N–Ti1−xZrxO2 could not utilize the visible light efficiently; however, it had the strong potential required to reduce oxygen leading to the formation of active oxygen radicals (Fig. 11).178 The N–Nb–TiO2 (25 mol% Nb) prepared by sol–gel process, followed by nitridation under flowing ammonia, exhibited a 6-fold increase in MB degradation under visible light compared to other Nb doped samples (<25 mol%).179 The energy of N 2p levels are close to the hydroxyl radical potential [E(H2O/˙OH) = 2.37 eV] such that the photoinduced holes can react with surface hydroxyl species to form hydroxyl radicals.180–182 However, the O 2p levels are low enough in energy for the photoinduced holes to oxidize either H2O or surface OH− to ˙OH. The XPS results revealed that mole percentage of substituted nitrogen in the TiO2 was a linear function of the mole percentage of niobium. The incorporation of Nb5+ in the sol–gel reaction led to the charge compensation as depicted below:183 |
 | (20) |
|
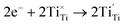 | (21) |
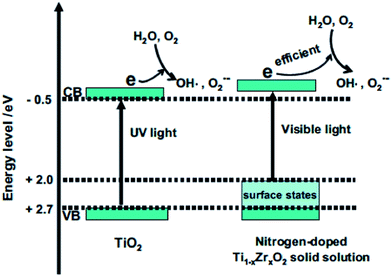 |
| Fig. 11 Schematic illustration of band structure of TiO2 and nitrogen-doped Ti1−xZrxO2 solid solution as photocatalysts for the production of various free radicals. Reprinted with permission from ref. 178, Copyrights (2013) Elsevier Publications. | |
The free electrons formed facilitated the reduction of Ti4+ to Ti3+ ions, which acted as recombination centers. At low mole percentages (1–5%), a stronger Ti3+ EPR signal and lesser substitutional nitrogen were found, while the opposite trend was observed at high mole percentages of niobium (10–30%).179 The N–Nb–TiO2 nanotube arrays showed an enhanced photoelectrochemical activity for water splitting under UV/visible light.184 The XPS results suggested that nitrogen content increased because of niobium predoping, which reduced the formation energy of co-doped systems compared to monodoped TiO2.185,186 The ratio between β-N and niobium was 0.46, suggesting that the positive charge induced by niobium was partially compensated by nitrogen at the surface. In addition, Nb reduced charge recombination by the presence of continuum like the hybridized states from N 2p to Nb 4d orbital above the VB instead of the localized states as observed in N–TiO2.185
The narrow bandgap of N–Mo–TiO2 was effective in RhB degradation under visible light.187 The electronic structure and optical properties calculated using polarized density functional theory revealed the continuum states of hybridized N 2p and O 2p states at the VB edge and Ti 3d and Mo 4d states at the CB lower energy edge. Moreover, localized states vanished completely from the forbidden bandgap, which prolonged the lifetimes of charge carriers.188,189 The N–Mo–TiO2 synthesized through the hydrolysis precipitation method combined with sonication post-treatment was positive for phenol degradation under visible light, which was attributed to small crystallite size, intense visible light absorption and narrow bandgap.190 On co-doping with Mo6+ and N−, the electrons migrated from N 2p level to CB and Mo6+ dopant level leading to the generation of more charge carrier density for participation in photocatalytic reactions. XPS results revealed that anionic N− substitutes oxygen atoms and coexist as β-N and γ-N, while Mo6+ substitutes Ti4+ lattice sites.190 The N–Cd–TiO2 was better for MO degradation under visible light, which was attributed to the modification of TiO2 structure as doping leads to crystal structure distortion, crystallinity reduction and an increased specific surface area that promoted the effective adsorption of organics on the catalyst surface.191 Crystal surface defects can inhibit electron–hole recombination on catalyst surface. Cadmium was present as CdCO3 due to a gradual expulsion from Ti–O framework to titania surface, and it hindered the crystal growth during thermal treatment. The first-principles calculations indicated that the energy gap of N–Cd–TiO2 became narrow and local internal fields induced by co-doping enabled photoexcited electron–hole pair separation more efficiently.191
4.2.3 Incorporation of nitrogen along with poor metal ions into titania lattice. The photoactivity of various catalysts for 4-CP degradation under UV/visible light was as follows: N–Sn–TiO2 > N–TiO2 > Sn–TiO2 > TiO2, attributed to the enhanced visible light absorption and the inhibition of charge carrier recombination.192 XRD studies revealed that there was no unit cell expansion for N–Sn–TiO2 despite the large ionic radius of nitrogen compared to oxygen, suggesting that nitrogen was distributed on titania surface rather than doping into the bulk. The Sn4+ substitutes Ti4+ lattice sites, which forms an energy level below the CB edge, while nitrogen on the surface generates energy states that are situated above the VB edge. During photocatalysis, electrons are excited from VB to Sn4+ energy level and from surface states energy level to CB. Moreover, CB electrons get trapped at Sn4+ energy level located at 0.4 eV below the CB.193 Since the energy level of O2/O2− is lower than Sn4+ and CB level, the excited electron are captured directly by surface adsorbed O2 molecules to form reactive oxygen species. In contrast, the holes in the VB and surface states oxidize hydroxyl groups to hydroxyl radical or can directly oxidize 4-CP molecules.192 Similarly, N–Bi–TiO2 was active for acid orange (AO7) degradation under UV/visible light.194 On visible light irradiation, electrons were excited from VB or nitrogen impurity states to titania CB, which were later captured by Bi4+/Bi3+ state. The density of oxygen vacancies induced by nitrogen dopant was decreased upon bismuth doping, which thus passivates the recombination centers and increases the photocatalytic activity.195 The N–In–TiO2 (In = 7%) exhibited high activity for 4-CP degradation under visible light irradiation than N–TiO2 and TiO2.196 XPS and diffused reflectance spectroscopy (DRS) results revealed unique chemical species such as N–O and O–In–Clx (x = 1 or 2) on the surface whose surface state energy levels were located close to VB and CB, respectively. The electrons were excited from both energy levels of N–O species to TiO2 CB and from VB to O–In–Clx (x = 1 or 2) on the surface, leading to an enhanced visible light absorption and charge carrier separation. Moreover, CB electrons may have fallen into O–In–Clx energy levels and the VB holes migrated to energy levels of N–O species. The 4-CP molecules adsorbed in the surface active sites were immediately oxidized by holes, resulting in complete decomposition.196
4.2.4 Incorporation of nitrogen along with 5d metal ions into titania lattice. Mesoporous N–W–TiO2 (W = 1.5%) showed a high tendency for RhB degradation under visible light due to the synergism in nitrogen and tungsten co-doping.197 Upon illumination, electrons were promoted from VB to tungsten impurity level, which was then captured by adsorbed oxygen giving rise to superoxide radicals, while holes reacted with surface adsorbed water to give hydroxyl radicals. Finally, these active oxygen species attacked dye radical cations and decomposed them.197 The N–W–TiO2 synthesized by microemulsion method, followed by a NH3 treatment (400 °C), showed selective oxidation of toluene and styrene under sunlight and the activity was attributed to the lack of tungsten-rich patches/zones on the surface, a decrease in bandgap and also the favorable surface properties for hydroxyl radical formation.198 The chemical entity of nitrogen species in N–W–TiO2 existed as (CN)n− species in bulk and as (NHx)n+ on surface. The increase in calcination temperature >450 °C resulted in a detrimental effect since tungsten was excluded from TiO2 lattice, resulting in a large heterogeneity of the nitrogen species.199 The superior activity of N–W–TiO2 (1% W) with twist-like helical structure for phenol degradation under UV/visible was ascribed to following reasons:200 (i) high specific surface area, which allows for the efficient diffusion of electrons, vacancies and adsorbed molecules to catalyst surface, which leads to photoreactions;201 (ii) stable mesoporous architecture and 3D connected pore system improved the molecular transport of reactants and products; (iii) W6+ trapped the CB electron to prevent formation of recombination centers; (iv) existence of Ti3+ and tungsten dopant created a new band below CB that served as reactive electron-trapping sites; and (v) highly crystalline anatase phase facilitated the transfer of vacancies from bulk to surface. The twist-like helical structure was formed due to the use of acetic acid in the synthesis, where the bidentate acetate ligand replaced chloride ions and bonded to metal ions in TiCl4 precursor. This modified the polymeric structure at the molecular level to promote the emergence of 3D type polymeric structure, which were later transferred to the helical structure with regular ventages by the decomposition of acetate ligands in the gel. Acetic acid inhibits the hydrolysis condensation process, which prolongs the gelation time and prevents the formation of irregular TiO2 particles.202 The N–W–TiO2 (3 wt% W) nanoparticles fabricated by combining sol–gel and mechanical alloying method showed activity for MB degradation, while increasing the tungsten content to 10 wt% favored sulfosalicylic acid degradation under visible light.203 The ‘F’ center induced by oxygen vacancies and donor–acceptor level induced by co-dopants served as carrier traps that contributed to high photocatalytic activity. XRD results showed the presence of anatase, rutile, srilankite and brookite phases, where srilankite phase was formed only in the ball milling process. During mechanical activation, the powders were repeatedly welded, fractured and re-welded. Note that mechanical alloying increases the contact area between reactant powder particles as a result of reduced particle size and allows fresh surfaces to come into contact repeatedly. As a consequence, phase transformations that normally require high temperature proceeds favorably at low temperatures. A large number of defects, such as vacancies and dislocations, could be induced into TiO2, which can significantly accelerate the atom diffusion process to induce nitrogen incorporation. |
2(NH2)2CO → NH3 + NH2CONHCONH2
| (22) |
|
3(NH2)2CO → 3NH3 + C3H3N3O3
| (23) |
The NH3 adsorbed on surface and interface released active [N] and [H] under high pressure, which diffuse faster through defects created by mechanical alloying. [H] reacts with lattice oxygen to produce new oxygen vacancy, while active [N] occupies the oxygen vacancy position or serves as an interstitial atom via O–Ti–N bond.203 The N–W–TiO2 showed exceptional potential for partial oxidation of styrene under visible light due to the combined effect of co-doping. The theoretical calculations revealed that titanium cation vacant sites (VTi) and surface wolframyl (W
O) species compensated for the extra charge of the W6+ and N anions in N–W–TiO2.204 The incorporation of nitrogen at substitutional positions with the concomitant presence of W
O species introduced localized states within the bandgap. The nitrogen impurity incorporates preferentially into the first coordination sphere of tungsten cation by ∼0.43 eV with respect to the equivalent positions of the first coordination sphere of the titanium cation. The stability diagrams for nitrogen incorporation in an undoped and tungsten-doped anatase {101} surface show that the implantation energy of substitutional nitrogen decreases with increasing tungsten content. On the contrary, interstitial nitrogen is destabilized by the presence of tungsten impurity (Fig. 12). The nitrogen doping allowed the absorption of visible light as electrons in VB were excited into these mid gap states and later to CB. The placement of W
O π levels above VB partially overlapped with N 2p levels, which facilitated the electrons to excite from this mid gap state. As a result, a weaker W–O and highly specific reactive surface oxygen radical was formed. The W
O entity was regenerated at the surface by the presence of oxidative atmosphere, which was a thermodynamically favorable process with ΔE = 1.3 eV.204 The visible light activity of N–W–TiO2 electrode sensitized with Fe–chlorophyllin (Fe3+–Por) towards MO and phenol degradation was found to be dependent on the concentration of sensitizer.205 The activity was maximum at 2 mM Fe3+–Por and decreased at higher concentrations since the electrons were inclined to be consumed on TiO2 surface. Adsorption of Fe3+–Por at high concentrations hinder the surface activity and light absorption capacity of TiO2, resulting in a lower photoelectrocatalytic (PEC) activity (Fig. 13).206 In addition, 3d orbital of tungsten lowered the CB level and 2p orbital of nitrogen shifted the VB upwards.
|
TiO2 + hv → TiO2 (eCB−h+)
| (24) |
|
Fe3+–Por + eCB− → Fe2+–Por
| (25) |
|
TiO2–Por + hv → TiO2 (e−)–Por+
| (26) |
|
TiO2 (e−)–Por+ + O2 → [TiO2–Por+] + O−2˙
| (27) |
|
O−2˙ + organic → degradation products
| (28) |
|
Ti–OH˙− + organic → degradation products
| (30) |
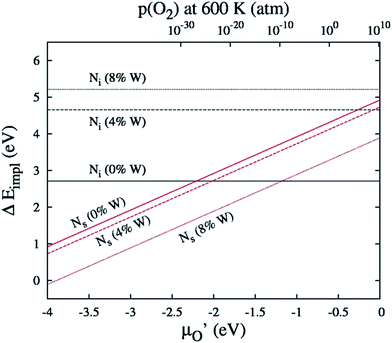 |
| Fig. 12 Implantation energies (in eV) as a function of the oxygen chemical potential μ′O (bottom axis) or O2 partial pressure (top axis) at a fixed temperature (T = 600 °C) for interstitial and substitutional nitrogen dopant in W-doped and undoped (101) anatase surface models. Reprinted with permission from ref. 204, Copyrights (2012) American Chemical Society. | |
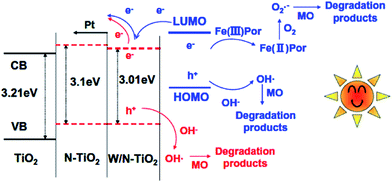 |
| Fig. 13 The illustration of the band structure of TiO2, N–TiO2 and N–W–TiO2–Fe–Chl excited under visible illumination showing photosensitization by Fe3+–porphyrin. Reprinted with permission from ref. 205, Copyrights (2012) Elsevier Publications. | |
4.2.5 Incorporation of nitrogen along with f block metal ions into titania lattice. The advantages of the lanthanides (Ln) as doping agents for TiO2 can be outlined as follows: (i) the complexing ability of Ln provides an effective mode for the adsorption of organic pollutants on TiO2 surface; (ii) effective trapping of CB electrons by Ln when they are confined to the TiO2 surface; and (iii) the electronic structure of lanthanide ions, which could lead to different optical properties and the formation of labile oxygen vacancies with relatively high mobility.207–209 The lanthanide ions with special 4f electron configurations are known for their ability to form complexes with various Lewis bases like acids, amines, aldehydes, alcohols, and thiols. This interaction can increase the extent of adsorption of organic pollutants on the catalyst surface and is beneficial for improving the photocatalytic activity.210The N–Ce–TiO2 was exceptional for MO and reactive red dye X-3B degradation under visible light due to the combined effects of nitrogen and cerium dopant in the titania lattice.211,212 The nitrogen doping narrowed the bandgap, whereas cerium with varied valence and special 4f level served as an electron trap.213–215
|
Ce3+ + O2 → O−2˙ + Ce4+
| (32) |
The existence of Ce4+/Ce3+ pairs created a charge imbalance, which resulted in the adsorption of a greater number of hydroxide ions on the surface and produced large number of hydroxyl radicals.216 In addition, cerium doping also increased TiO2 surface area by decreasing their crystallite size. The XRD and thermogravimetric-differential scanning calorimetry (TG-DSC) analysis showed that cerium dopant inhibited the phase transformation to rutile as Ce4+ ion with a larger ionic radius cannot substitute Ti4+ ion; therefore, it surrounded the anatase crystalline forming Ti–O–Ce bonds at CeO2–TiO2 interface.217 At this interface, Ti4+ was expected to substitute for Ce4+ in CeO2 lattice to form octahedral Ti sites. This interaction between tetrahedral Ti and octahedral Ti inhibited the anatase to rutile phase transformation.218,219 Similar results were also found for N–Pr–TiO2 towards BPA decomposition under visible light.220,221 In contrast, N–Nd–TiO2 showed poor photocatalytic activity for malachite green degradation compared to undoped and individually doped TiO2.222
The Sm–N–TiO2 with 1.5 mol% samarium calcined at 400 °C exhibited high activity for salicylic acid decomposition under visible light owing to its appropriate crystallite size, high surface area, and good adsorptive capacity due to the formation of Lewis acid–base complexes between Sm–N–TiO2 and salicylic acid (Fig. 14).223 In addition, the recombination process was effectively inhibited by the samarium dopant. The optimal dopant concentration of samarium was found to be 1.5 mol% as the charge carriers are efficiently separated only when the thickness of space charge region is equal to light penetration depth.224,225 The dopant inside the matrix can suppress or enhance the recombination depending on its concentration and the position of its energy level. The charge carrier trapping at low dopant concentration may not be obvious but at high concentration they may serve as recombination centers. Thus, optimal dopant concentration is necessary to prolong the lifetime of charge carriers. Beyond the optimum dopant concentration, the rate of recombination starts to dominate the reaction in accordance with the following equation:226,227
|
KRRα exp(−2R/a0)
| (35) |
where
KRR is the rate of recombination,
R is the distance separating the electron and hole pairs, and
a0 is the hydrogenic radius of the wave function for the charge carrier.
228,229 As a consequence, the rate of recombination increases exponentially with the dopant concentration. This is because the average distance between the trap sites decreases with increasing number of dopant atoms. On the other hand, the thickness of space charge layer is influenced by the dopant concentration according to the following equation:
|
 | (36) |
where
W is the thickness of space charge layer,
ε and
ε0 are the static dielectric constants of the semiconductor and vacuum respectively,
Vs is the surface potential,
Nd is the number of dopant donor atoms and
e is the electronic charge. The equation above clearly shows that
W decreases as the dopant concentration increases. In addition, penetration depth (
l) of the light into the solid is given by
l = 1/
a, where
a is the light absorption coefficient at a given wavelength. When the value of
W approximates that of
l, all the photons absorbed by the solid catalyst generates electron–hole pairs that are efficiently separated. Consequently, the existence of optimum value of
Nd, for which a space charge region whose potential is not less than 0.2 eV and whose thickness is more or less equal to light penetration depth, can be understood by the equations above.
224,225 The charge carriers generated beyond the space charge region are not under the influence of electric/potential field and hence recombine rapidly. At high dopant concentrations, the dopant level itself can act as recombination sites for the charge carriers evidently by decreasing the photocatalytic activity. The N–Eu–TiO
2 particles with spheroidal shape synthesized through modified sol–gel method exhibited high photocatalytic activity for the degradation of brilliant red X-3B under visible light illumination, ascribed to the high crystallinity and bandgap narrowing.
230 The Eu 4f level is shown to trap the CB electron to activate superoxide radical formation.
231,232 The N–Eu mesoporous TiO
2 microspheres with yolk–shell structure (TiO
2@void@TiO
2) obtained by facile one-pot hydrothermal method at low temperature (180 °C, 8 h) showed better activity for RhB and MO degradation under visible light illumination due to the synergistic effects induced by unique yolk–shell structure with high specific surface area and large pore volume.
233 The scanning electron microscopy (SEM) and TEM techniques revealed that the formation of yolk–shell structure followed the OR mechanism.
234–237 XRD and XPS studies suggested that Eu
3+ ions were distributed over titania surface since it is difficult to incorporate them into TiO
2 matrix at low temperature (180 °C) due to its larger radius (0.112 nm) compared to Ti
4+ ion (0.064 nm). The abundant mesoporous structure was formed by CO
2 gas bubbles released during the hydrothermal decomposition of urea. Upon excitation, electrons located in localized nitrogen states were stimulated to CB and they were trapped quickly by Eu 4f level, followed by a reaction with molecular oxygen.
238,239 The photogenerated holes formed in localized nitrogen states reacted with hydroxyl groups and H
2O molecule to produce hydroxyl radicals, which degraded the pollutant molecules.
240 |
TiO2 (e−) + Eu3+ → TiO2 + Eu2+
| (37) |
|
Eu2+ + O2 → Eu3+ + O2˙−
| (38) |
|
Eu2+ + O2 + H+ → Eu3+ + H2O2
| (39) |
|
H2O2 + O2˙− → OH˙ + OH− + O2
| (40) |
|
TiO2 (h+) + H2O (or H2O2 or O2˙−) →→ Products
| (41) |
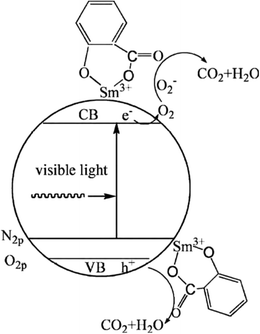 |
| Fig. 14 Formation of Lewis acid–base complexes between Sm–N–TiO2 and salicylic acid and its photocatalytic process under visible light irradiation. Reprinted with permission from ref. 223, Copyrights (2012) Elsevier Publications. | |
N–Gd–TiO2 prepared by hydrothermal method showed high photocatalytic activity for RhB degradation under visible light illumination.241 The activity was attributed to the inhibition of particle growth, which resulted in larger surface areas and surface active sites.242 In addition, Gd3+ ion on the surface acts as sensitizer and absorbs light energy and transfers it to TiO2, thereby enhancing its activity.243
4.3 Photocatalytic activity of tridoped TiO2
The tridoped C–N–S–TiO2 hollow microspheres prepared by fluoride-induced self-transformation using L-cysteine as carbon, nitrogen and sulphur source showed superior activity for brilliant red X-3B degradation under visible light illumination.244 This enhancement in the activity was due to the following reasons: (i) the ability of photocatalyst to harvest photons from visible light; (ii) the enrichment of dye adsorption on catalyst surface; and (iii) the effective charge carrier separation by two phases of the same semiconductor-undoped and doped TiO2.245–247 In another study, C–N–S–tridoped TiO2 nanorods prepared by hydrothermal method with 1
:
25 molar ratio of L-cysteine to TiCl4 showed a high removal efficiency for gaseous NO under simulated solar light irradiation.248–251 The XRD study revealed an enhancement in the anatase fraction with an increase in the amount of L-cysteine at the expense of rutile. During the synthesis of undoped TiO2, large amount of H+ ions produced by TiCl4 hydrolysis increased the solution acidity, leading to the production of rutile phase. In the presence of L-cysteine, –NH2, –COOH and –SH groups coordinated with the Ti atoms to bind L-cysteine to neighbouring protonated octahedrons sharing one edge. When another TiO6 octahedra complex attacks the two octahedra sharing one edge with L-cysteine, only a spinal chain of octahedrons forms because of the steric hindrance given by L-cysteine linked tightly to the terminal Ti ions which inhibit rutile formation.248 The C–N–S–TiO2 (molar percent of cysteine to TiO2 [R] is 4.2) was active for phenol degradation under simulated sun light illumination, which was attributed to the large number of surface hydroxyl groups and efficient charge carrier separation.252 XRD results showed only the anatase phase for C–N–S–TiO2 and triphasic for undoped TiO2 (85% anatase, 10% rutile and 5% brookite) (Table 3). The XPS studies revealed that S6+ substituted the Ti4+ ion, and nitrogen occurred at both substitutional (N–O–Ti) and interstitial (O–Ti–N) position and carbon existed as a mixed layer of carbonate on TiO2 surface. The C–N–S–TiO2 (R = 4.2) showed a stronger surface photovoltage signal (SPS) at 345 nm because of an electronic transition from O2− anti bonding orbital to the lowest empty Ti4+ orbital;253 the stronger the SPS signal, the higher the charge carrier separation as SPS signal arises from electron–hole pair generation followed by separation under in-built electric field (also called space-charge layer). In positive electric field, SPS signal increases markedly due to the same direction of added outer and in-built electric field and the signal becomes broad, which is ascribed to the trap-to-band transitions from surface states, while SPS response decreases for negative electric field, which is characteristic of n-type semiconductors.254 The highly transparent N–C–F–TiO2 films prepared by a simple layer-by-layer dip-coating method using TiO2 sol and NH4F methanol solution as precursors without additional pore inducing agent showed an enhanced activity for stearic acid degradation under visible light.255 The high performance originated from the combined effects of tridoping and high surface area of the photocatalyst.255 The doped carbon on the surface acts as sensitizers, which could be excited to inject electrons into TiO2 CB.256 The doped nitrogen atoms improve the visible light absorption, while doped fluorine atoms facilitated the formation of oxygen vacancies, which are important active species for initiating a photocatalytic reaction.257,258 Initially, a layer of TiO2 was coated onto the glass substrate, followed by a second layer of NH4F methanol solution, and a third coating of TiO2 sol. The first TiO2 coating prevents fluoride ions from reacting with the glass substrate. Subsequent coatings of NH4F solution and TiO2 sol prevent immediate precipitation of the reactants. The ensuing heat treatment at 500 °C results in a reaction between NH4F and TiO2 sol to form N–C–F–TiO2. The gaseous byproducts (NH3 and HF) induce high surface roughness in TiO2 coating and generates nonirradiated superhydrophilic surface. This superhydrophilicity is mainly due to the high roughness and highly accessible pores in N–C–F–TiO2 films that reduce diffusion resistance within the film structure and subsequently allows a better penetration of water through the void.259 The contact angle measurements of the films were 2.3°–3.1° in the absence of illumination, and they increased slowly in the dark (<1.8° in 30 days), which could be applied for fabricating self-cleaning surfaces.255 The contact angles were tested without light illumination and kept in the dark for 1–30 days.
Table 3 Phase composition, grain size, surface area and micropore volume values of P25–TiO2, undoped TiO2 and C–N–S–TiO2 (R = 4.2) photocatalysta
Sample |
Phase composition (%) |
Grain size (nm) |
Surface area (m2 g−1) |
Micropore volume (cm3 g−1) |
Anatase |
Rutile |
Brookite |
Reprinted with permission from ref. 252, Copyrights (2012) Elsevier Publications. |
P25–TiO2 |
82 |
18 |
0 |
23 |
58.2 |
— |
Undoped TiO2 |
85 |
10 |
5 |
6.94 |
111 |
0.140 |
C–N–S–TiO2 (R = 4.2) |
100 |
0 |
0 |
5.23 |
194 |
0.230 |
The N–S–Fe–TiO2 synthesized through simple one step sol–gel reactions in the presence of ammonium ferrous sulphate showed visible light activity for phenol degradation.260 The dopants Fe3+, S4+ and S6+ ions were substituted at Ti4+ lattice site and nitrogen coexisted as both substitutional (O–Ti–N) and interstitial (Ti–O–N) positions. The mechanism of photocatalytic process is shown in the following equations.260
|
F–N–S–TiO2 + hv → hVB+ + eCB−
| (42) |
|
Fe2+ + O2(ads) → Fe3+ + O2−
| (44) |
|
Fe2+ + O2(ads) → Fe3+ + O2−
| (45) |
|
O2− + 2H+ + eCB− → OH˙ + OH−
| (46) |
|
OH˙ + C2H5OH → … → CO2 + H2O
| (48) |
The high SPS response of Fe–N–S–TiO2 was due to the improvement in crystallinity, which made semiconductor electronic band structure perfect, which lead to a decrease in surface defects and promoted the charge separation and transfer process.260
The ellipsoidal N–F–W–TiO2 particles around 20 nm in length and 10 nm in width showed 98% RhB degradation and 94% carbon removal under visible light.261 The doping of fluorine induces the formation of Ti3+ to a higher extent and the dopant W6+ ion forms energy level below CB that serves as electron trapping sites to reduce recombination. On the other hand, nitrogen doping created an acceptor level, which facilitates visible light absorption to produce more charge carriers.262,263
|
W6+ + e− (TiO2)CB → W5+
| (49) |
|
W5+ + h+ (TiO2)VB → W6+
| (50) |
In another study, MO degradation by N–S–Gd–TiO2 (Gd = 0.6 at wt%) was observed under visible light and the activity is ascribed to the following reasons:264 (i) an increase in charge carrier lifetime by Gd3+ ions using 4f level for trapping the electrons and the results were substantiated by fluorescence lifetime studies, and (ii) all dopants introduced new electronic states in bandgap, which enhanced visible light absorption.265–267 The PL studies revealed that PL signals were largely raised from the defect level and that the peak maximum around 430 nm was due to an increased electron density at the oxygen site as a result of nitrogen and sulphur substitution in TiO2 lattice, both of which are less electronegative than oxygen.264
The N–F–Ta–TiO2 with 1% molar ratio of Ta to Ti using NH3 and TaF5 as precursors showed a degree of efficiency for RhB and phenol degradation under visible light due to synergistic effects such as increased crystallite surface area, increased hydroxyl radical generation and high visible light absorption.268 EPR and XPS studies revealed that N–Ta interaction induces charge compensation to form fully occupied continuum like the N 2p–Ta 5d hybridized states in VB edge, which promotes visible light absorption and improves charge carrier separation (Fig. 15). The fluorine dopant facilitated nitrogen incorporation, which promoted the formation of N 2p–Ta 5d hybridized states. The coexistence of tantalum and nitrogen narrows the bandgap by a charge transfer from the positively charged tantalum to the negatively charged nitrogen, leading to the formation of stable N–Ta chemical bond. The Nn (nitrogen atom next to the tantalum atom) and Nf (nitrogen atom without an adjacent tantalum atom) existed in the tridoped TiO2 and the holes produced in N 2p band from Nn had high oxidation power than N 2p band of N–TiO2.269 The N–C–Ce–TiO2 mesoporous membrane synthesized via a weakly alkaline sol–gel route using P123 template and calcined at 450 °C showed potentials for MO degradation under visible light.270 The cerium ion facilitated successful doping of nitrogen and carbon atoms, which lowered the bandgap to 2.14 eV and improved the visible light activity. The cerium doping strengthened the Ti–O bond and inhibited the transformation to rutile and rapid growth of the crystals, thereby preventing the collapse of assembled pores during the calcination process. The filtration experiments of the composite membrane presented a low cut-off molecular weight of 3300 Da and pure water flux of 4.05 Lm−2 h−1 per bar. This suggests that such photocatalytic membranes have great implications for environmental applications like wastewater treatment because of their ability to decompose dissolved organic contaminants while simultaneously removing pollutants.270
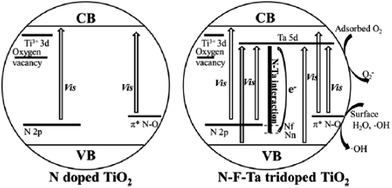 |
| Fig. 15 Comparison and modification of band structure for N–TiO2 and tridoped N–F–Ta–TiO2. Reprinted with permission from ref. 268, Copyrights (2012) Elsevier Publications. | |
The N–Yb–P–TiO2 with optimal 5 wt% phosphorous dopant exhibited the highest photoactivity for MB degradation under UV/visible light due to the cooperative effects of tridoping.271 The doped phosphorous inhibited crystal growth and recombination of charge carriers by serving as electron trap centers and enhanced the photon absorption efficiency.272 In addition, substituted P5+ ion induced a charge imbalance, which was compensated by additional surface hydroxyl groups and by the decrease of oxygen vacancies. The Yb3+ ions acted as electron scavengers, and then released them to surface adsorbed O2 to form superoxide radicals. Moreover, tridoping increased the number of surface hydroxyl groups and decreased the point of zero charge of TiO2 from 6.58 to 3.29, contributing to the higher degree of adsorption and dispersion of MB molecules on the surface. The increased mesopore sizes were beneficial to mass transfer because they allow the rapid diffusion of reactants and products. During photocatalytic reactions, the apparent rate constant indicated that ytterbium and phosphorous doping enhanced the activity, while N–TiO2 lowered its activity compared to undoped TiO2 (Table 4). On the contrary the activity of N–P–TiO2 was higher than N–TiO2 but lower than P–TiO2 indicating the absence in synergetic effect for N–P co-doped catalyst.271 The N–P–Mo–TiO2 showed efficient activity for MB and sulfosalicylic acid degradation under visible light than Mo–TiO2 and P–N–codoped TiO2, which was attributed to an efficient reduction in bandgap.273 The phosphorous and nitrogen dopants introduced acceptor levels above VB while the Mo donor levels were found below the CB edge. XRD results showed MoO3 phase formation at higher Mo content (0.72), which was beneficial for improving the photoactivity of N–P–Mo–TiO2.273
Table 4 First order kinetic apparent rate constants and relative coefficients for the degradation of MB over various photocatalysts under visible and UV light irradiationa
Photocatalyst |
Kapp under visible light irradiation (min−1) |
R2 |
Kapp under UV irradiation (min−1) |
R2 |
Reprinted with permission from ref. 271, Copyrights (2013) Elsevier Publications. |
TiO2 |
9.2 × 1 0−3 |
0.995 |
1.06 × 10−3 |
0.986 |
Yb–TiO2 |
1.09 × 10−2 |
0.990 |
1.44 × 10−2 |
0.992 |
N–TiO2 |
7.35 × 10−3 |
0.996 |
5.42 × 10−4 |
0.992 |
P–TiO2 |
2.95 × 10−2 |
0.992 |
2.90 × 10−3 |
0.999 |
N–Yb–TiO2 |
1.92 × 10−2 |
0.996 |
1.44 × 10−3 |
0.999 |
N–P–TiO2 |
2.52 × 10−2 |
0.991 |
2.22 × 10−3 |
0.999 |
N–P–Yb–TiO2 (1 wt% PO43−) |
1.56 × 10−2 |
0.990 |
1.59 × 10−3 |
0.999 |
N–P–Yb–TiO2 (5 wt% PO43−) |
4.66 × 10−2 |
0.996 |
4.15 × 10−3 |
0.990 |
N–P–Yb–TiO2 (10 wt% PO43−) |
1.81 × 10−2 |
0.982 |
2.58 × 10−3 |
0.992 |
5 N–TiO2 coupled with other semiconductors
The coupling of two semiconductors with suitable band edge potentials lead to the construction of heterojunction interface between the semiconductors with electric-field-assisted charge carrier transport from one semiconductor to the other via interfaces.274,275 The geometry of particles, surface texture, and particle size play a significant role in interparticle electron transfer.276,277 Appropriate placement of an individual semiconductor and an optimal thickness of the covering semiconductor are crucial for efficient charge separation. In addition, abundant charge carriers will be available at the surface/interface of the two semiconductors for redox reactions and will also extend the energy range of photoexcitation.278,279
The N–TiO2 obtained by direct amination of DP25 and triethanol amine as nitrogen source showed visible light activity for MO degradation.280 XPS and wavelength dispersive spectroscopy spectra (WDS) spectra proved that nitrogen atoms substituted titanium (y = 27) and oxygen (x = 0.36) lattice sites with 21% nitrogen dopant concentration in the Ti–O–N–O linkage (nitrogen substituted for titanium) and Ti–N–Ti–O/Ti–N–Ti–N linkage (nitrogen substituted for oxygen). In addition, Ti–O–N–N linkages also originated from nitrogen substitution for both titanium and oxygen.281 Thus, the formation of new heterostructure (TiO2)m/(Ti1−y−mO2−x−2mNx+y) having both wide and narrow bandgaps improved the visible light absorption and charge carrier separation as a result of vectorial transfer of electron and hole from narrow band (TiO2)m–(Ti1−y−mO2−x−2mNx+y) to wide bandgap TiO2.280 The 1D N–TiO2 nanorods with anatase–brookite heterostructure (82.4% anatase and 17.6% brookite) showed high activity for MO and 4-CP degradation compared to TiO2 nanoparticle without 1D structure under UV/visible light.282,283 This architecture was designed by one-pot solvothermal method (200–220 °C, 48 h) using hydrazine hydrate and TiO2 colloids as starting materials. During the alkaline thermal process, titania nanoparticles are dissolved in solution and recrystallized to give TiO2 embryos. When the N2H4·H2O/TiO2 colloids concentration ratio was 16.8, N–TiO2 had a smaller nanorod content, since the growth of TiO2 embryos was retarded by the increase in the number of N2H4 molecules per unit volume concentration of TiO2 colloids. At the same time, if the N2H4·H2O/TiO2 colloids ratio was below 2.8, ellipsoidal nanoparticles were observed. Under visible light, electrons from localized N 2p states in both anatase and brookite phases were excited to individual CB leaving holes in localized states. Moreover, band edge positions of anatase and brookite facilitate the interfacial migration of electrons from brookite to anatase. The energy barrier between anatase and brookite suppresses back electron transfer, which functions like a one-way valve inhibiting the recombination rate.284–288 The same scenario occurs under UV light except that electrons in VB are excited to CB (Fig. 16).282 N–P25TiO2/amorphous Al2O3 composites fabricated via one-step solution combustion method exhibited enhanced activity for MO and MB degradation mediated by hydroxyl and superoxide radicals.289 This was ascribed to the cooperative effects of amorphous A12O3, and the nitrogen doping in TiO2 and large surface area.289 When visible light was irradiated, electron was either transferred from the nitrogen impurity state to CB of Al 3d level or from direct excitation from VB to Al 3d level (Fig. 17). The distance between aluminium and oxygen atoms in combustion-synthesized amorphous Al2O3 samples became shorter and energy bandgap became smaller, which favored electron transitions.290 Amorphous Al2O3 has a high electron transfer ability from P25 as it contains more defect sites than crystalline A12O3.289
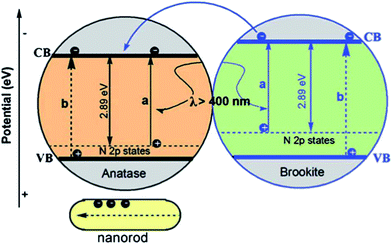 |
| Fig. 16 Electron migration process in one-dimensional nanorod along with nitrogen-doped anatase and brookite structures: (path a) under visible light irradiation; (path b) under UV light illumination. Reprinted with permission from ref. 282, Copyrights (2012) Royal Society of Chemistry. | |
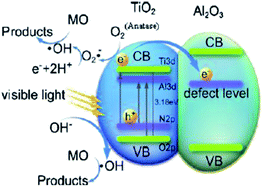 |
| Fig. 17 Pictorial representation of the possible photodegradation mechanisms of MO over N–TiO2/Al2O3 composite under visible light irradiation. Reprinted with permission from ref. 289, Copyrights (2012) Elsevier Publications. | |
The pore wall structure and surface properties of mesoporous N–TiO2/ZrO2 resulted in fast ethylene decomposition in air under visible light.291 The introduction of ZrO2 stabilized the porous structure by rendering large surface to volume ratio, inhibited anatase to rutile transition and further stabilized nitrogen in the N–Ti–O structure. The preservation of interconnected porous nanonetworks facilitate the transport of small molecules through interior space and favored visible light harvesting through multiple scattering within the solid framework.292 The performance of N–TiO2/V2O5 (0.5 wt% V2O5) was appreciable for gaseous toluene degradation under visible light compared to N–TiO2, SiO2/V2O5 and V2O5.293 Since the band edge potential of V2O5 (ECB0 = 0.48 eV) was lower than that of N–TiO2 (ECB0 = −0.19 eV) CB level, electron transfer from N–TiO2 to V2O5 was thermodynamically favorable, which facilitated the partial reduction of V5+ to V4+, the electron from which was then detrapped to the adsorbed oxygen to promote charge separation.294–297
|
V5+ (V2O5) + e− → V4+
| (51) |
|
V4+ + O2 → V5+ (V2O5) + O2−˙
| (52) |
The mesoporous SiOC/20 wt% N–TiO2 (65 wt% anatase and 35 wt% rutile) ceramic composites prepared by the incorporation of N–TiO2 powders into the vinyl-functionalized polysiloxane polymer, followed by pyrolysis (700 °C, 2 h in argon atmosphere), enhanced the MB degradation rate under UV/vis illumination.298 This was attributed to changes in electronic structure, synergistic effects of higher surface area and high mesoporosity that promoted the pollutant adsorption on catalyst surface. XRD results showed that pyrolysis temperature strongly influenced the phase stability as pure TiO2 was stable till 800 °C, and the aggregation of dopants in TiO2 retarded the phase transformation from anatase to rutile at this temperature. On increasing the pyrolysis temperature to 900 °C, the anatase phase disappeared completely with the formation of new Ti4O7 phase and a small amount of rutile. Above 900 °C, rutile peaks vanished completely, and Ti4O7 and Ti2O3 phases started to dominate. In addition, microporous pure SiOC gets was transformed to mesoporous composite due to a structural rearrangement of polymer chains on the addition of N–TiO2. The intensity of UV/visible absorption spectra was high for SiOC/20 wt% N–TiO2 owing to the presence of amorphous SiOC matrix incorporation to crystalline oxide powders, which increased the electric charge of the oxide and resulted in the modification of electron–hole formation process.299 The hollow N–Co–TiO2/SiO2 microspheres showed an enhanced RhB degradation under visible light irradiation compared to N–TiO2/SiO2, TiO2/SiO2 and Co–TiO2/SiO2.300 Cobalt served to broaden the visible light absorption.
The N–TiO2/ZnO nanotube arrays composite with well-aligned surface structure showed gaseous NOx decomposition under UV/visible light, which was superior to ZnO nanorods, ZnO nanotube, N–TiO2, commercial TiO2 and ZnO nanorod arrays/N–TiO2 composite.301 The heterojunction structure promoted vectorial charge transfer from one semiconductor to another with thermodynamically favorable band edge position, leading to an increased interfacial charge transfer and catalytic efficiency (Fig. 18). The peculiar tubular nanostructure provided large surface area for NOx adsorption and the photons were perfectly trapped and absorbed by both inner and outer walls of the nanotubes.301 The N–TiO2/Bi20TiO32 (10% Bi) increased the rate of 2,4-DCP degradation under visible light due to the combined effects of visible light absorption, low charge carrier recombination and improved crystallinity accompanied by a reduction in intrinsic surface defects.302,303 XRD and XPS results showed the presence of Bi20TiO32 on titania surface, ascribed to the abundant bismuth ions and interactions between surface titanium and bismuth ions during calcination. Apparently, Bi20TiO32 has a small bandgap of 2.09 eV, where VB is composed of hybridized energy levels which are largely dispersed involving Bi 6s and O 2p orbitals, which increased the charge carrier mobility.304,305 The ability of Bi–O bond to donate electrons helps in transferring the electrons to surface adsorbed species, which eliminates the recombination pathways. The excited electrons from nitrogen impurity band are transferred to titania and Bi20TiO32 CB under visible light. Moreover, these excited electrons can also be captured by doped Bi4+/Bi3+ ions with energy levels below titania CB and subsequently transferred to adsorbed oxygen to produce superoxide radicals and VB holes, which directly oxidizes hydroxyl anion. Finally, Bi20TiO32 also absorbs visible light generating charge carriers for further redox reactions.302
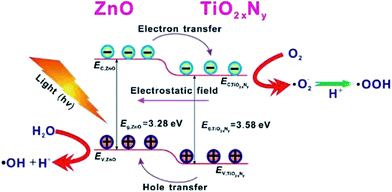 |
| Fig. 18 Schematic illustration of charge carrier separation and band structure of ZnO/N–TiO2 semiconductor heterojunction. Reprinted with permission from ref. 301, Copyrights (2012) Elsevier Publications. | |
The high adsorption of SMZ on N–P25–TiO2/activated carbon (AC) resulted in its faster degradation under solar light.306 This composite had an anatase–rutile mixture that increased the efficiency of charge-carrier separation by transferring electrons from anatase to rutile CB307,308 and also from rutile CB to anatase trapping sites (Fig. 19).309 In addition, AC support reduced the agglomeration of supported N–TiO2 nanoparticles, whereas doped nitrogen created localized states within the bandgap, which served as trap sites for holes.310,311 The effect of ultrasonication disruption on the physical stability revealed that N–P25–TiO2 (20 + 15)/AC exhibited a greater resistance to titania dislodgement due to a stronger chemical bonding between N–P25 and N–TiO2 (sol).306 The N–TiO2/AC exhibited high adsorption capacity and degradation rates for BPA over a range of excitation sources and demonstrated high potential for reuse compared to unsupported photocatalyst and binary mixture of titania and AC.312 Furthermore, the calcination of the composite under air removed organic residues to permit greater exposure of titania surface to receive incident light. The creation of interfacial energy between surface AC and N–TiO2 resulted in an anti-calcination effect, significantly restraining rutile growth.313 The carbon-coated N–TiO2 mesostructure prepared by one-step solvothermal method with chitosan as carbon and nitrogen source showed high efficiency in the visible light degradation of MB compared to only carbon coated TiO2, which was attributed to bandgap narrowing and the heterojunction formed between the carbon and N–TiO2.314 The nitrogen doping level above the VB shifted the absorption to visible light region315 and converted some Ti4+ to Ti3+, which then formed a donor energy level below the CB, enhancing its light absorption. The carbon species acted as surface sensitizers and transported the electrons to TiO2 CB, in addition to enhancing the MB adsorption on the catalyst surface due to the existence of acidic surface groups.314 The N–TiO2 nanoparticles decorated on graphene sheets [N–TiO2/GB] was active for MO degradation under visible light (Fig. 20).316 The graphene in composite extends visible light absorption as a result of a chemical binding via Ti–O–C bond between TiO2 and graphene. The excellent conductivity and two-dimensional planar structures facilitated rapid transport and the separation of charge carriers.317,318 The N–S–TiO2/graphene oxide (5% GO) composite showed an enhanced activity for MO degradation under UV light irradiation.319 The high activity was ascribed to a large surface area, oxygen-containing functional groups and large aromatic domains that were inclined to be bound by conjugated MO molecules via π–π stacking. In the composite, excited electrons were transferred from CB to GO via percolation mechanism that accelerates the interfacial electron transfer process.320 In addition, the dopants increased the number of electrons and holes to form reactive species, and the number of oxygen vacancies induced for charge compensation was reduced by co-doping.319 The N–TiO2/8 wt% graphite-like carbon nitride (g-C3N4) showed a significantly enhanced activity for RhB decomposition under fluorescence light irradiation due to dye adsorption and the sensitizing role of g-C3N4.321 SPS revealed a small SPS signal for N–TiO2/g-C3N4 (8 wt%), indicating a minimum band bending difference and an accumulation of static electrons on the surface.
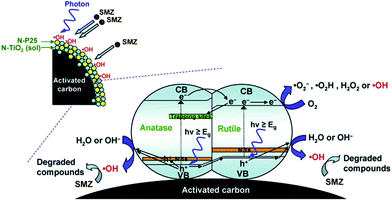 |
| Fig. 19 Schematic illustration of the proposed photocatalytic mechanisms of anatase and rutile N–TiO2 supported on activated carbon. Reprinted with permission from ref. 306, Copyrights (2012) Elsevier Publications. | |
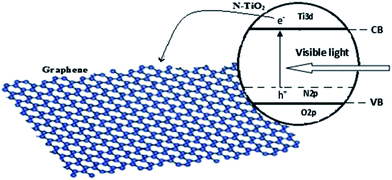 |
| Fig. 20 Proposed mechanism for the enhanced visible light photocatalytic activity of graphene/N–TiO2 composite photocatalysts. Reprinted with permission from ref. 316, Copyrights (2012) Elsevier Publications. | |
A dual phase material (DP160) comprising hydrated titanates (H2Ti3O7·xH2O) and anatase TiO2 showed phenol degradation under visible light attributed to the excitation via inter band states, surface sensitization, improved adsorptive properties of aromatic compounds due to the presence of N-carbonaceous species layer containing Ti–N bonds (e.g. –O–Ti–NH+–R–NH+–Ti–O–) and heterojunction-like structure promoting directional interfacial charge transfer.322
A lepidocrocite-type titanate K0.81Ti1.73Li0.27O4 coupled with N–TiO2 composite showed excellent photocatalytic activity for the decomposition of NOx gas under UV/visible light.323 This activity was attributed to the bridging structure formed between nano- and plate-like particles together with the increased specific surface area, which provided better accessibility for the target molecules. Moreover, sandwich-like structure increased the dispersion of N–TiO2, thereby inhibiting their agglomeration. The recombination of charge carriers was effectively depressed due to the different bandgap structures of K0.81Ti1.73Li0.27O4 and N–TiO2.323 The N–TiO2/CaAl2O4: (Eu, Nd) luminescent composite prepared by a soft planetary ball milling at 200 rpm for 20 min exhibited potential activity for gaseous NO degradation under UV light and the persistent activity continued even after the light source was turned off for 3 h, which was attributed to the depression of agglomerated N–TiO2 nanoparticles and visible light absorption by N–TiO2 via long afterglow emitted by CaAl2O4: (Eu, Nd) even in the dark.324 On irradiating CaAl2O4: (Eu, Nd), electrons and holes were produced in Eu2+ ions while Nd3+ ions captured some free holes moving into the VB. When the excitation source was cut off, some holes captured by the Nd3+ ions acting as traps were thermally released to the excited state of Eu2+, accompanied by the emission of light. This emission was a symmetrical band at 440 nm, a strong blue emission, which was attributed to the typical 4f6 5d1–4f7 transition of Eu2+.325 The N–TiO2 with nitrogen impurity band above VB induced a second bandgap about 2.34 eV, which was lower than the emitted blue luminescent energy.45 Hence N–TiO2 was excited once the light was turned off. When the composite sample was prepared by planetary ball milling at 200 rpm, the extent of degradation of de NOx increased with an increase in the ball-milling speed due to homogeneous mixing. However, the de NOx degradation decreased at ball-milling speed >200 rpm due to an increase in lattice strain and defect, which promoted the recombination of charge carriers. The optimum loading was found to be 40% N–TiO2, leading to a better dispersion of N–TiO2 on the CaAl2O4: (Eu, Nd) surface, which is a phosphor material.324 Similarly, N–TiO2/Sr4Al14O25: (Eu, Dy) was active for acetaldehyde removal in the dark compared to N–TiO2/CaAl2O4: (Eu, Nd).324
6 Metal deposition on N–TiO2 (M/N–TiO2)
The deposition of various metals like Pt, Ag, Au, Pd, Ni, Rh and Cu on semiconductor surface enhances photocatalytic activity by modifying semiconductor surface properties, discharging photogenerated electrons across the interface and providing a redox pathway with low overpotentials to inhibit the recombination of charge carriers.326–330 The formation of a space-charge layer at the semiconductor–metal interface facilitates charge separation under bandgap excitation. It is known that the surface plasmons created by the noble metal deposits on the catalyst surface couple with the electric fields of the incident radiations to enhance the separation of photogenerated charge carriers and assist in the charge carrier transfer process across the catalyst/liquid interface.331,332
The photoactivity of various catalysts towards MO and MB degradation under visible light shows the following order: Ag/N–TiO2 hollow spheres > Ag-hollow spheres > Ag–N/P25 > Ag–P25 > N/hollow sphere > N/P25.333 This was ascribed to the bandgap narrowing by nitrogen doping and the presence of silver clusters, which served as an electron sink thereby improved the charge separation.334 The embedded silver nanoparticles between N–TiO2 pillars degraded RhB under visible light. The induced localized surface plasmon absorption edge of silver particles in the visible region and its role as an electron sink favored efficient photocatalysis.335 The N–TiO2 pillar was synthesized by reactive dc sputtering to produce TiN porous film followed by a simple oxidation process at elevated temperature in oxygen or air, while the silver nanoparticles were embedded between N–TiO2 pillars by the photoreduction of Ag+ ions in aqueous solution under visible light. The bandgap of N–TiO2 was tuned by controlling the oxidation temperature, oxygen concentration and oxidation time. In air at 900 °C, maximum oxidation was achieved in 5 h, corresponding to the blue shift from 530 (at 1 h oxidation) to 500 nm (after 5 h). In contrast, in pure oxygen at 900 °C, the blue shift to 500 nm was completed within 1 h and no further change took place upon increasing duration (up to 10 h), suggesting that the replacement of nitrogen by oxygen is a gradual process and saturation level is achieved after 5 h.335 The Ag/N–TiO2 prepared via hydrothermal process in silver–ammonia solutions showed better visible light activity for RhB degradation.336 XPS results suggested that Ag loading on TiO2 surface significantly restrained the removal of nitrogen dopant from the bulk lattice during the hydrothermal treatment (130 °C, 3 h) and its probable removal gradually decreased with an increase in Ag content (Fig. 21). This stabilization of nitrogen dopant was attributed to the electron transfer from Ag 5s to N 2p orbital. Since the energy of N 2p (N2−) is lower than Ag 5s orbital, the electron transfers from Ag 5s to N 2p orbital to make a completely filled (N3−) electronic configuration. The fluorescence spectra revealed that the activity increases gradually with increasing Ag content initially and decreases after reaching the optimal Ag/Ti molar ratio of 0.92 mol%, suggesting that optimum content of Ag will serve as electron traps, while superfluous Ag will be detrimental.336 The Ag/N–TiO2 hollow nanorod arrays synthesized on glass substrate by one-pot liquid phase deposition method using ZnO nanorod arrays as template exhibited the highest photocatalytic activity for MB degradation under UV and visible light illumination with 0.03 and 0.07 M AgNO3 concentrations, respectively.337 This enhanced activity was attributed to the synergistic effects of Ag loading, nitrogen doping and the multiphase structure of anatase–rutile.337 Raman results suggested that AgNO3 additive in the precursor solution promoted anatase to rutile phase transition. XPS studies showed an Ag 3d peak around 367.8 eV with a negative shift compared to bulk Ag (368.2 eV) owing to electron transfer from TiO2 to metallic Ag deposit.338–340 Under visible light, electrons were excited from VB and nitrogen impurity energy levels to TiO2 CB, which were then trapped by Ag deposits, favoring interfacial charge transfer process.341 The Ag/N–C–TiO2 showed an enhanced activity for DCP degradation under visible light and inhibited luminescent activity of Vibrio fisheri owing to TiO2-induced oxidative stress, which led to genotoxicity and cytotoxicity in the microbial organism.342,343
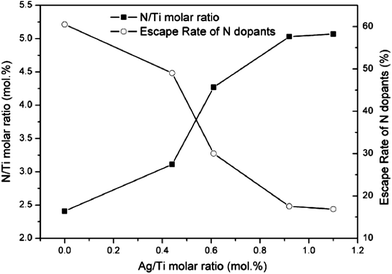 |
| Fig. 21 Effect of the amount of Ag loading on the stability of implanted nitrogen in TiO2 after hydrothermal treatment. Reprinted with permission from ref. 336, Copyrights (2013) Elsevier publications. | |
The Pt/N–TiO2 exhibited enhanced oxidation kinetics for acetic acid, toluene and acetaldehyde. Compared to N–TiO2, Cu- and Fe-loaded N–TiO2 showed high rates for acetic acid and formic acid photooxidation, respectively.344 The extremely high rate of formic acid oxidation over Pt/N–TiO2 was due to the combined effect of photocatalysis and thermal catalysis (in the dark) at room temperature, facilitated by the presence of nanosized Pt (1–2 nm) on the surface. The ESR studies confirmed that the presence of O− and O3− stabilized on the catalyst surface were responsible for this dark thermal catalysis. The Fenton-like reactions were found to be operative with Fe- and Cu-loaded N–TiO2 leading to high degradation rates.344 Fe/N–TiO2 and Pt/N–TiO2 improved the visible light induced activity for the decomposition of de NOx, whereas Pt/N–TiO2 exhibited activity several times higher than that of N–TiO2.345 The activity was related to the chemiluminescence intensity generated by singlet oxygen (1O2). The rate of degradation of de NOx (gaseous NOx pollutant) decreased with an increase in chemiluminescence emission intensity, indicating that singlet oxygen generation was detrimental for de NOx degradation. The photoexcitation of titania led to the formation of superoxide radicals, which are a doublet with one unpaired electron (−41.4 kJ mol−1). The holes may trap one electron from a superoxide radical to produce a singlet oxygen or a triplet oxygen. The singlet oxygen has a pair of electrons in one orbital and a second equal-energy empty orbital. Metastable singlet oxygen possesses more energy (94.7 kJ mol−1) than ground-state triplet oxygen (0 kJ mol−1).346 From the energy level, it is observed that superoxide radical is produced easily and quickly, while singlet oxygen is formed slowly and requires extended energy (Fig. 22). The formation of singlet oxygen competes with the formation of superoxide and hydroxyl radicals:345
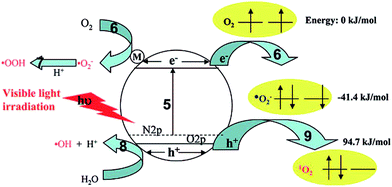 |
| Fig. 22 Schematic illustration of the photoinduced charge transformation on N–TiO2 with metallic loading. The formation of singlet oxygen competes with the formation of superoxide and hydroxyl radicals. Reprinted with permission from ref. 345, Copyrights (2008) American Chemical Society. | |
The simultaneous surface and bulk modification achieved through Pt or Au deposition and N–F-codoping was favorable for downhill reaction (formic acid oxidation) and uphill reaction (H2 production).347 The NH4F doping guarantees that the most active TiO2 phase is stabilized at high calcination temperatures, ensuring high crystallinity and good photoinduced charge carrier production, whereas Pt or Au deposition on the catalyst surface increased the charge carrier separation. However, Pt was a better co-catalyst than Au for photocatalytic activity, which was related to their work function, i.e. energy required to promote an electron from the Fermi level to vacuum. The greater the difference between the metal work function and the semiconductor is, the higher will be the height of Schottky barrier generated by the band alignment at the metal semiconductor heterojunction.348 The efficiency of photogenerated electron trapping by the metal and subsequent transfer to oxygen molecules adsorbed on the catalyst is consequently increased.347 The high photoactivity of Pd/N–TiO2 (0.6% Pd) for eosin yellow decomposition under visible light arise from the synergistic effects of palladium deposition and nitrogen doping.349 The palladium on TiO2 surface creates a Schottky junction between the metal and the semiconductor, which acts as a sink for photogenerated electrons.349 The noble metal loading (Pt, Au and Ag) on mesoporous N–TiO2 improved the CO2 photoreduction to methane by water under visible light. XPS results indicated that the dopant nitrogen existed as a molecularly chemisorbed nitrogen species (N2) or nitroxide species (e.g. NO and NO2), which induced visible light activity.350 The efficiency of the co-catalyst followed the order of Pt > Au > Ag with an optimum loading of 0.2 wt%, 0.02 wt% and 0.1 wt%, respectively. This optimum metal island traps more number of electrons, whereas an excess amount would serve as recombination centers and reduce the light absorption capability of the catalyst. The Pt has a higher work function of 5.65 eV than Au (5.1 eV) and Ag (4.26 eV);351 thus, electrons can transfer more efficiently to Pt. Simultaneously, the adsorbed water molecules react with holes to form H+, which in turn reacts with electrons detrapped by Pt to produce H˙ radicals that subsequently reduce CO2 to hydrocarbons.352–354 The optimum nitridation temperature to achieve the best activity was 525 °C, and the optimum amount of nitrogen was 0.84% on the basis of lattice oxygen atoms. A larger amount of nitrogen would result in defect sites and nonstoichiometry in the material. The blank test performed with 0.2 wt% Pt/TiO2 in the presence of water vapor, and in the absence of CO2 under UV illumination in N2 atmosphere revealed that methane could be produced from the catalyst associated with carbon residues.350 The N–TiO2 (B) nanofibers decorated with Pt nanoparticles showed the highest hydrogen generation rates of 700 μmol h−1 and 2250 μmol h−1 under UV-A (365 nm) and UV-B (312 nm) light irradiation compared to Pd-decorated N–TiO2 nanofibers, which was attributed to the direct electron transition from p-states to the empty states of Pt nanoparticle that are more energetically favored compared to the conventional transition involving VB or impurity level to CB, followed by a subsequent transfer to the metal.355 The higher activity under UV-B exposure was due to the presence of a sufficient amount of high energy photons to induce the generation of electron–hole pairs. The N–TiO2 (A) nanofibers were synthesized by the calcination of H2−xNaxTiyO2y−1 nanofibers at 600 °C in ammonia gas flow for 15 h, while N–TiO2 (B) was synthesized from H2−xNaxTiyO2y−1 calcined in air at 600 °C for 12 h to form TiO2 anatase nanofibers, followed by a second calcination step in ammonia gas at same temperature for 3 h.355 In Pt/N–TiO2, Pt has a smaller particle size and a high dispersion value on catalyst surface compared to Pd.355 The amine-functionalized, silicate sol–gel supported, gold-deposited N–P25 (APS/Au–N–P25) showed an enhanced photocatalytic oxidation for CO and reduction of Hg(II) ions under visible light, attributed to the synergistic effects of gold nanoparticles (with sub 5 nm size) deposited on N–P25.356–360 When Au and NP25 are connected electrically, electron migration from TiO2 to Au occurs until the two Fermi levels are aligned. Hence, the metal surface acquires the excess negative charges and semiconductor becomes positively charged. The Au acts as an electron sink, which increases the interfacial charge transfer process and minimizes the recombination rates. The presence of –NH2 group in amine-functionalized silicate sol–gel with nitrogen having nonbonding electrons allows the interaction of Hg(II) ions with the APS/Au–N–P25 surface.361,362 This results in the increased adsorption and preconcentration of Hg(II) ions on the surface. The Au transfers electrons instantaneously to reduce Hg(II) to Hg and the holes in the VB oxidize the oxalic acid which acts as a sacrificial electron donor. The optimization of Au was found to be 2 and 4 wt% for the reduction of Hg(II) ions and CO oxidation, respectively. The excess Au loading decreased the activity due to the following reasons: (i) absorption and scattering of incident light by excess Au;363,364 (ii) the shielding of incident photon at N–P25 by Au; and (iii) the negative effect on photoreduction due to the oxidation of Au by holes or surface hydroxyl radicals.356
The mesoporous Cu/N–TiO2 showed good degrading ability for gaseous xylene under UV/visible light, which was ascribed to its mesoporous structure.365,366 The catalytic activity decreased for Cu loadings higher than 0.6 mol% as the active sites on the catalyst were covered by excess Cu with a simultaneous increase of recombination kinetics.367 The bottles coated with Cu/N–TiO2 films and annealed at 600 °C degraded MB more rapidly than uncoated bottles in field trials, while N–TiO2 coating did not show any change as the holes produced by visible light irradiation in mid gap level (induced by doping) did not have sufficient redox potential to oxidize MB.368 The Cu-doped TiO2 showed E. coli and Enterococcus faecalis inactivation due to the increased visible light absorption and anti-microbial properties of copper atoms on exposed surfaces. However, the doping with nitrogen atoms (Cu/N–TiO2) reduced the degree of inactivation as nitrogen atom in lattice acted as recombination centers. The Cu/N–TiO2 accelerated bacterial inactivation when coated on glass beads, but not on internal surface of glass bottles, indicating that reactive species produced at Cu–N–TiO2 surface are short-lived and can only diffuse short distances (in the order of μm) and that bacterial inactivation by such reactive species may thus be transport limited. The H2O2 and superoxide radical are important reactive species that might plausibly have a mean diffusion distance on this length scale.369 Bacterial cells may also adhere to catalytic surface, thereby magnifying the effects of short-lived radical species.370 Note that the formation of singlet oxygen, a less oxidative reactive oxidation species, was reported to be responsible for bacterial decontamination.371 The loading of copper (0.5 wt%) on N–TiO2 was found to be beneficial for gaseous acetaldehyde and bactericidal activity under visible light, while loading with Pt, Zn and La did not exhibit any effect, suggesting that Cu serves as electron trap sites and extends the carrier lifetime.367,372
7 Hydrogen evolution using N–TiO2
The solar energy available in nature is 3 × 1024 J per year, which is 10
000 times higher than the present energy need.373 This provides a wide scope for photocatalytic reduction of water to generate clean and green fuel, i.e., hydrogen (H2), as an energy carrier with a high calorific value 122 kJ g−1 (2.75 times greater than hydrocarbon fuels).374 The recent findings reveal that N–TiO2 could be a better photocatalyst for H2 production.
N–TiO2 prepared by heating urea and TiO2 at 350–700 °C in air favored hydrogen evolution under UV/visible light in the presence of Na2SO3 as a positive hole scavenger.373 XPS studies showed both molecularly chemisorbed N2 and substituted nitrogen contributed to visible light response. The calcination temperature of the catalyst removed chemisorbed nitrogen, which had the least influence on photoactivity. The high activity of the sample calcined at 600 °C resulted from phase transformation based on the CB edge position. The flat band potential of rutile coincides exactly with NHE potential (H+–H2 level), whereas anatase is shifted cathodically by ∼200 mV, suggesting that the driving force for water reduction is very small for rutile.375 It is also evident that anatase–rutile mixture enhances electron–hole separation due to the operation of surface charge layer and space charge layer between the anatase and thin rutile layer. This combined space-charge layer acts cooperatively towards the migration of holes from anatase to rutile and to the surface.373 The platinized N–B–TiO2 (B = 1.5 at%) calcined at 350 °C showed remarkable photocatalytic activity for hydrogen production under visible light compared to N–TiO2, attributed to synergistic effects of nitrogen and boron doping.376 The nitrogen doping extends the visible light absorption via N–Ti–O bond while boron plays multiple roles: (i) B3+ substitutes Ti4+ lattice sites and serves as shallow traps for carriers;377 (ii) boron eliminates oxygen vacancies induced by nitrogen doping and enhances surface hydroxyl groups on its surface;378 and (iii) boron distributed on the catalyst surface forms space charge layer to separate electron–hole pairs.379 The N–Ce–TiO2 (0.6% molar ratio) calcined at 500 °C exhibited the highest hydrogen evolution under visible light irradiation, ascribed to synergistic effects of co-doping.380 XRD results indicated that as the ionic radii of Ce3+ and Ce4+ are larger than Ti4+, and they lead to lattice distortion and expansion where some strain energy is accumulated in the crystal, reducing the phase transition from anatase to rutile.380
The hydrogen evolution with Pd co-catalyst and methanol as sacrificial reagent for various photocatalysts followed the order of N–In–TiO2 > In–TiO2 > N–TiO2 > TiO2.381 The indium doping at cationic site decreased the bandgap by mixing of 5s and 5p orbitals with Ti 3d orbital without generating any trap sites, making the electrons available at CB for the reduction of protons to hydrogen.382 In addition, impregnated Pd facilitated interfacial electrons transfer and reduced the overpotential for hydrogen evolution by accumulating the electrons on Pd deposits.383–385 The co-catalyst NiO supported on N–TiO2 showed high hydrogen generation from aqueous methanol solution under sunlight-type irradiation, which was ascribed to electron trapping nature of NiO.386 These trapped electrons in NiO initiate H+ reduction formed by water splitting to liberate hydrogen.387 The nitrogen doping decreased the bandgap and increased the number of electrons in the presence of NiO. During hydrogen evolution reaction, oxygen and nitrogen were not liberated, suggesting that titanium oxynitride synthesized by trioctyl amine treatment of TiO2 was stable under reaction conditions.386 In another study, Pd served as better co-catalyst compared to NiO on reduced N–TiO2 for hydrogen evolution under visible light.388 The induced Ti3+ state produces anion vacancies in the lattice, which introduces additional level below CB. These levels overlap with TiO2 CB, which decrease the bandgap with increase in its concentration.388 The Pt (1 wt%) loaded N–S–TiO2/V2O5 exhibited the highest activity for hydrogen evolution (296.6 μmol h−1) under visible light, as CB electrons are trapped by Pt to reduce H+ ions and the holes oxidize methanol to form CO2 and H2.389–392
|
 | (55) |
|
 | (58) |
|
 | (59) |
|
 | (60) |
The CB and VB of N–S–TiO2 lie above the energy band of V2O5. Thus, electrons accumulate in N–S–TiO2 CB and holes in V2O5VB effectively separating charge carriers at the interface.393–396 However, Pt-deposited N–TiO2 thin film showed poor hydrogen gas evolution under visible light compared to UV light due to the presence of oxygen defects, which trapped the electrons necessary to initiate hydrogen production.50 The nanocomposite of graphene oxide with N–TiO2 exhibited enhanced photocatalytic efficiency for hydrogen evolution under UV/visible light, which was attributed to nitrogen doping and incorporation of graphene oxide.397 The nitrogen doping and formation of Ti–C and Ti–O–C bonds along with the existence of Ti3+ red shifted the bandgap of TiO2. The Fermi level in graphene is −4.42 eV398 is close to CB energy (−4.21 eV) of TiO2. Therefore, the CB electrons are injected into the Fermi level orbital in graphene, while the holes are trapped by N–TiO2. At the same time, graphene can withdraw and shuttle electrons as electron transporting bridges and electron sinks because of its ultrahigh electron mobility (>1000 cm2 V−1 s−1), and the plate-like monolayer textural nature with a large specific surface area affords quick electron transfer resulting in charge carrier separation. The electrons from graphene are transferred to H+ in aqueous methanol to afford hydrogen generation, and the holes irreversibly oxidize the sacrificial reagents instead of water.397
8 Reactivity of N–TiO2 with exposed facets
Engineering TiO2 material with exposed crystal facets is currently a hot area in photocatalytic reactions. Both theoretical and experimental studies have revealed that {001} surface of anatase TiO2 nanosheets is much more reactive than the thermodynamically stable {101} surface.399 Based on thermodynamics, {101} facet with low surface energy remains the most stable, accompanied by diminishing {001} and {110} facets, during crystallization. The average surface energy of {001}, {100} and {101} facets are 0.90, 0.53 and 0.44 J m−2, respectively.400 Owing to the low atomic coordination numbers of exposed atoms and the wide bond angle of Ti–O–Ti, the anatase TiO2 {001} facet is theoretically considered to be more reactive than the {101} facet in heterogeneous reactions.
Doping with nonmetals is reported to shift the bandgap response of {001} facets of anatase titania towards the visible region. N–TiO2 with an exposed {001} facet (∼67%) prepared by solvothermal synthesis of TiN in a HNO3–HF ethanolic solution exhibited a higher activity for hydrogen evolution compared to N–TiO2 microcrystallite with exposed {001} facet due to the larger surface area of the former compared to the latter. In addition, ethanol used in the synthesis process served as a capping agent, which hindered the growth of the single crystal anatase TiO2 because of its specific bonding with the TiO2 surface via the Ti–O–C bond.400b The solvothermal treatment of TiN in acidic NaBF4 resulted in N–TiO2 with a dominant {001} facet, which exhibited high activity for MB degradation and excellent photoelectrochemical properties under visible light. The presence of weak Ti–B–F surface structure facilitated easy dissociation and exchange of surface hydroxyl groups to form hydrogen peroxide and peroxide radicals.401,402 The photocurrent (400 μA cm−2) response of N–TiO2 with a dominant {001} facet is 3.3 times higher than N–P25, indicating efficient charge separation and transportation of electrons from TiO2 surface to counter electrode via an external circuit under visible light illumination.401a Hydrogenated N–H–F–TiO2 with {001} facet exhibited high activity for RhB degradation under visible light due to the formation of energy belts and disorder structures.403 The differently coordinated Ti3+ and oxygen vacancies like Ovac (Fe), Ovac (Ne) and Ti3+ (Fe), Ti3+ (Ne), Ti3+ (H), and Ti3+ (H′) (e refers to escape, for example, Ovac (Fe) refers to oxygen vacancy induced by the escape of F− ions) narrowed the bandgap and significantly enhanced light absorption to facilitate charge carrier migration (Fig. 23).403 The N–Mo–TiO2 sheets with dominant {001} facets obtained by a hydrothermal process using TiN, MoO3, HF and HNO3 showed activity for MB and methyl violet degradation under visible light.189 The XPS spectra confirmed the nitrogen at interstitial site or O–Ti–N structure and Mo6+ substitution in TiO2. The Mo doping elevated the CB edge and expanded the TiO2 bandgap, raising the reduction potential energy and improving the H+ reduction ability. The removal of fluorine from the catalyst surface reduced its activity, although the adsorption of the pollutant on the catalyst was enhanced.189 The N–Ni–TiO2 with exposed {001} facets prepared by a two-step hydrothermal reaction using H2Ti3O7 and HF (shape controlling agent) showed enhanced activity for MB degradation under UV/visible light. This can be attributed to its suitable bandgap (2.0 eV) and band edge positions, which matched the redox potential of water.404 The dopants, nitrogen and nickel, shifted VB edge up significantly but left CB unchanged. The n-type (Ni) and p-type (N) with unequal charge states decreased the density of recombination centers and increased the migration efficiency of charge carriers. For the synthesis process, H2Ti3O7 nanotubes were obtained by treating TiO2 with NaOH. At this stage, few Ti–O–Ti bonds were broken, followed by the formation of Ti–O–Na bonds, which on washing with acid and water generated new Ti–O–H bonds to produce metastable H2Ti3O7 that were further transformed into TiO2 nanotubes as shown below.404
|
TiO2 + NaOH → Na2Ti3O7
| (61) |
|
Na2Ti3O7 + HCl → H2Ti3O7
| (62) |
|
H2Ti3O7 → 3TiO2 + H2O
| (63) |
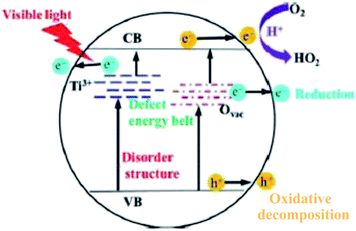 |
| Fig. 23 The schematic illustration of band structure, disorder structure and defect energy belt of N–H–F–TiO2 and its visible light photocatalytic activity. Reprinted with permission from ref. 403, Copyrights (2012) Elsevier publications. | |
The hierarchical N–TiO2 hollow sphere-in-sphere microstructure comprised of nanothorns with exposed anatase {101} facets exhibited potential activity for MB and orange II degradation under visible light. This was ascribed to the synergistic effects of large surface area, hierarchical structure, and crystalline {101} facets on the surface and inner sphere, which allowed multiple reflections of light with effective usage of incident photons.405 Water was found to play a major role for hollowing process, which promoted the crystallization and dissolution processes. When the molar ratio of H2O/Ti is 0.3, formation of nanothorns on the surface of spheres occurs, while heavily aggregated TiO2 solid microspheres with nanoflakes are formed without water. The hollow structure improves the specific surface area dispersion ability and light harvesting ability of the photocatalyst. However, hollow spheres containing {101} facets without sphere-in-sphere structures exhibited low activity.405–408
The MB degradation was achieved at a faster rate under visible light on the surface of N–C–TiO2 nanosheets with exposed {001} facet compared to their microsheets and nanoparticles counterparts.409 The fluorine ions preferentially adsorbed on {001} facets hindering crystal growth along {001} axis but facilitated crystalline growth in both {100} and {101} directions with lower surface energy.410 Moreover, the ethanol served as a capping agent by adsorbing on TiO2, which hindered the aggregation of nanoparticles and promoted nanocrystals formation.411,412 The carbon and nitrogen co-doping intensified the absorption in the visible region and facilitated the exposure of a large amount of reactive facets.409 The N–F–TiO2 platelets with dominant {001} facets prepared via solid state phase pathway, i.e. by nitriding TiOF2 precursor in NH3 gas flow demonstrated excellent performance for water oxidation under visible light.413 The presence of fluorine dopant facilitates nitrogen doping into the crystal by maintaining charge balance and stabilizes the structure.414 When TiOF2 was treated with NH3 gas flow, the latter facilitated the collapse of TiO2 boxes into platelets and the simultaneous incorporation of nitrogen atoms into the TiO2 lattice. Moreover, a negligible amount of O2 was detected with N–TiO2 because water oxidation reaction requires the removal of four protons and four electrons and the formation of an oxygen–oxygen double bond.413 The N–S–TiO2 nanosheets with exposed {001} facets prepared by a simple mixing-calcination method using the hydrothermally prepared TiO2 nanosheets showed an enhanced degradation of 4-CP under visible light irradiation, which is attributed to the synergetic effects as follows:415 (i) the red-shift of absorption edges and intense absorption in the visible light region, and (ii) the exposure of highly reactive {001} facets of nanosheets. The former effect indicates more photogenerated electrons and holes participating in the photocatalytic reactions. The latter effect is due to 2D nanosheets with exposed {001} facets, which adsorbs pollutant molecules better due to their higher reactivity. Under visible light irradiation, electrons can transfer from VB to impurity states easily, and the electrons in the impurity states move to CB after a secondary excitation. The intermediate states offer “extra steps” for the absorption of low energy photons via the excitation of electrons at the top of VB to the intermediate bands, where they can be excited again to the CB bottom level, resulting in an effective photoresponse. The photocatalytic experiments indicated that pure TiO2 nanosheets show visible light activity for 4-CP degradation on irradiation. The substrate–surface complexation formed by a phenolate linkage reaction between the ligand and Ti(IV) site on the TiO2 surface as shown below.
|
RTi–OH + OH–Ph → RTi–O–Ph + H2O
| (64) |
This complexation extends the absorption of titania to visible light region through ligand to titanium charge transfer.415 The N–S–TiO2 nanocrystals with high percentage of {001} facets synthesized via solvothermal process, followed by calcination with thiourea at 300 °C, demonstrated superior MB degradation and photocatalytic inactivation of E. coli bacteria under visible light.416 This superior activity is ascribed to the reduced crystal size with {001} facets, which largely increased their specific surface area (70 m2 g−1) and the contact efficiency with contaminants.416 The N–F–Cr–TiO2 microspheres were prepared by hydrothermal method by treating the thermally sprayed TiN/Ti coating with HF aqueous solution and chromium powder at 180 °C for 5–24 h.417 In the absence of chromium powders, the interaction of TiN/Ti with HF resulted in an interconnected, truncated bipyramids, while high energy {001} facets were formed in the presence of chromium microspheres. The increase of surface vacancy due to chromium doping increases the surface energy, contributing to the selective erosion of the truncated bipyramids to a drum-like morphology. In addition to the incorporation of nitrogen, fluorine and chromium led to a significantly enhanced solar absorption and reduced the bandgap remarkably.417
9 Application of N–TiO2 in organic reactions and organic synthesis
Since various intermediates are formed during titania photocatalysis, degradation reactions can be tuned at specific intervals so as to obtain the required organic compounds, which otherwise demand harsh conditions or multiple steps for their preparation under organic protocols. Thus, most of the organic compounds can be obtained in a single step under laboratory conditions.418,419 N–TiO2 displayed a reductive cleavage of azoxybenzene into their corresponding amines or 2-phenylindazoles with methanol in the presence of N2 atmosphere under UV/solar light illumination.420 The amines (aniline) were obtained in pure methanol, while 2-phenylindazoles was formed in aqueous methanol (20% water + 80% methanol). The product yield decreased with an increase in carbon chain length of alcohol, highlighting the importance of solvents in tuning the reaction rate and corresponding yields. On illumination electron–hole pairs are generated on TiO2, in which CB electron is captured by oxygen, retarding amine formation via reduction in the aqueous methanol system. The hole oxidizes methanol to generate hydroxymethyl radical and hydrogen radical that react with azobenzene to form a hydroxymethyl adduct, which later dehydrates and cyclizes to indazole or can be cleaved by protons to form amines (Fig. 24).421 The as-prepared tubular TiO2 immersed in 1 M acetic acid was effective in the ring opening reaction of an epoxide and Friedel–Crafts reaction.422 The protonation of oxygen atom in styrene oxide from Bronsted acidic site, followed by the attack of nucleophilic part of methanol onto this carbon center, lead to the ring opening reaction (Fig. 25). Friedel–Crafts reaction is initiated by the activation of CO group in methyl vinyl ketone through protonation, followed by an attack of π electrons in indole upon vinyl group, suggesting that the catalyst exhibited a prominent acidic nature.422
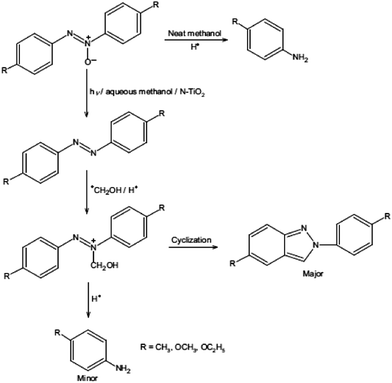 |
| Fig. 24 Possible reaction pathways for the reductive cleavage of azoxybenzene into their corresponding amines. Reprinted with permission from ref. 420, Copyrights (2012) Elsevier publications. | |
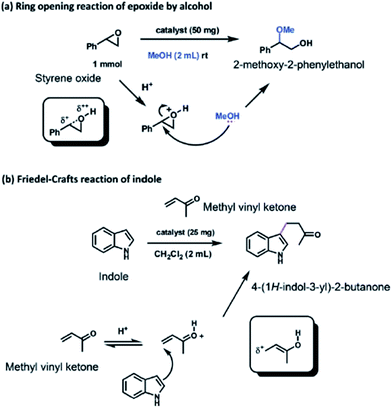 |
| Fig. 25 Schematic representation of (a) ring opening reaction of epoxide by alcohol (b) Friedel–Crafts reaction of indole. Reprinted with permission from ref. 422, Copyrights (2011) American Chemical Society. | |
The activity of various catalysts towards one-pot synthesis of quinaldines from nitrobenzene in ethanol under both UV and visible light followed the order of N–TiO2 > Pt–TiO2 > Au–TiO2 > Ag–TiO2 > bare TiO2.423 The photoirradiation of alcohol solution of different nitroarenes with N–TiO2 showed that the electron releasing group at the para position inhibits the condensation of amino group with aldehyde. In the case of 3,5-dimethylnitrobenzene, cyclization reaction was hindered due to steric effect, which decreased the product yield (66%) compared to 3-nitrobenzene (80%). The photoinduced dehalogenation resulted in lower yields in the case of 4-chloro and 4-fluronitrobenzenes (Table 5). Contrarily, the addition of KI and NaI increased the product yield due to the hole scavenging effect of the iodide ion.423 In another study, N–TiO2 promoted rapid and selective quinaldine production from an ethanolic solution of aniline and with other substituted anilines under UV/visible light.424 The initial step involved the oxidation of alcohol to aldehyde via VB holes from N–TiO2. The oxidized product of ethanol condensed with aniline to produce an imine (Schiff base), which on further condensation and cyclization yielded corresponding quinaldine. There was no significant effect on the product yields with other substituents except for halo anilines. In the case of 4-chloro and 4-fluoroanilines, the yields of halo-substituted quinaldines were very low due to a photoinduced dehalogenation before the reactions. N–TiO2 with poor crystallinity prepared at low temperature using NH4Cl as the nitrogen source showed a partially enhanced oxidative transformation of 4-methoxybenzyl alcohol to p-anisaldehyde under simulated solar light.425 The high reactivity was due to the synergistic effect of anatase–rutile phases,426 which reduced the recombination rate. In addition, the nitrogen in bulk improved the reaction rate and its presence on the catalyst surface reduced mineralization sites, which increased the selectivity. Though the thermal treatments yielded an improvement in crystallinity and activity (in terms of initial disappearance rate) were highly detrimental for selectivity and exploitation of UV light. This is due to the primary influence of particles sintering and higher crystallinity leading to high activity and low selectivity.425 These types of photoinduced chemical transformations, leading to the formation of industrially important compounds, are encouraging to further explore applications of TiO2 in organic synthesis.424
Table 5 Photocatalytic synthesis of various substituted quinaldines using N–TiO2a,b
Reactant |
Product yield (%) |
Byproduct |
Conversion |
All reactions were performed with a 25 mM alcoholic solution of the reactant containing 50 mg of N–TiO2 suspension, intensity (I) = 1.381 × 10−6 einstein L−1 s−1, irradiation time = 5 h. Reprinted with permission from ref. 423, Copyrights (2012) Royal Society of Chemistry. |
Nitrobenzene |
Quinaldine (70) |
29 |
99 |
3-Nitrobenzene |
2,7-Dimethylquinoline (80) |
18 |
98 |
4-Nitrobenzene |
2,6-Dimethylquinoline (75) |
23 |
98 |
4-Methoxynitrobenzene |
6-Methoxy-2-methylquinoline (70) |
26 |
96 |
3-Methoxynitrobenzene |
7-Methoxy-2-methylquinoline (72) |
22 |
94 |
3,5-Dimethoxylnitrobenzene |
2,5,7-Trimethylquinoline (66) |
19 |
85 |
4-Chloronitrobenzene |
6-Chloro-2-methylquinoline (36) |
64 |
99 |
4-Fluoronitrobenzene |
6-Fluoro-2-methylquinoline (20) |
79 |
99 |
10 Conclusion
The superiority of N–TiO2 is demonstrated by its low cost and simple, controllable synthesis, high surface hydrophilicity and photoinduced charge carrier transfer from bulk to surface under visible light. Nitrogen has similar ionic size and posses low ionization energy to that of oxygen, which effectively controls both chemical and electronic state along with the surface structure of TiO2. Because of the comparable ionic size, induced lattice distortion will not be significant; hence a large number of recombination centers are not generated. In addition, nitrogen as a dopant does not segregate or lead to the formation of impure products like dopant oxide. Therefore, the interaction of nitrogen dopant within titania matrix and its homogeneous distribution within the host lattice remains an engaging research topic for researchers.
The following general conclusions can be drawn based from the available literature:
(i) The doping of nitrogen ion at substitutional or interstitial lattice position shifts the bandgap of titania to visible region, allowing it to operate under solar light.
(ii) Na2EDTA, gaseous NH3, NH4OH, NH4Cl, NH4NO3, (NH4)2CO3, NH2CONH2, C2H5NH2, N(C4H9)4–OH, guanidine, HMT and 1,3-diaminopropane are the most used nitrogen precursors.
(iii) The annealing atmosphere (oxidizing/reducing/inert) changes the dopant diffusion properties into the bulk of TiO2 lattice, affecting the relative chemical stability.
(iv) Depending on the experimental conditions the possible nitrogen states in TiO2 lattice are O–Ti–N, Ti–O–N, Ti–N–O, N–Ti–O, N–Ti–N, –(NO), –(NO2), Nb˙.
(v) Nitrogen doping is always accompanied by the creation of defects like Ti3+ ion or oxygen vacancies, which can either suppress or enhance recombination pathways, depending on their distribution in the lattice.
(vi) N–TiO2 with diverse morphologies like nanotubes, nanoflowers, hollow spheres, nanosheets, fibre, nano-octahedral, rice grain, V-shape were more beneficial compared to regular nanoparticles due to their unique structures.
(vii) Various modifications like co-doping with metal/nonmetal ions, coupling with other semiconductors or surface metal deposition influences the electronic band structure and induces exceptional stability to N–TiO2. These modifications impart multiple charge transfer pathways to hinder recombination process.
(viii) The stabilization of {001} facets by the nitrogen dopant is remarkable since such situation is hard to achieve with other metal ions.
(ix) The applications of N–TiO2 for hydrogen evolution and in organic synthesis are still at the initial stages and much more research work is needed to correlate material properties with the above probe reactions.
Acknowledgements
The authors acknowledge University Grants Commission (UGC) and Department of Science and Technology (DST-IDP & DST-SERC), Government of India for their financial supports.
References
- X. Lang, X. Chen and J. Zhao, Chem. Soc. Rev., 2014, 43, 473–486 RSC.
- J. B. Joo, M. Dahl, N. Li, F. Zaera and Y. D. Yin, Energy Environ. Sci., 2013, 6, 2082–2092 CAS.
- L. Q. Jing, W. Zhou, G. H. Tian and H. G. Fu, Chem. Soc. Rev., 2013, 42, 9509–9549 RSC.
- Y. J. Wang, Q. S. Wang, X. Y. Zhan, F. M. Wang, M. Safdar and J. He, Nanoscale, 2013, 5, 8326–8329 RSC.
- X. Y. Pan, M. Q. Yang, X. Z. Fu, N. Zhang and Y. J. Xu, Nanoscale, 2013, 5, 3601–3614 RSC.
- M. A. Lazar and W. A. Daoud, RSC Adv., 2013, 3, 4130–4140 RSC.
- S. Nishimoto and B. Bhushan, RSC Adv., 2013, 3, 671–690 RSC.
-
(a) Y. Q. Qu and X. F. Duan, Chem. Soc. Rev., 2013, 42, 2568–2680 RSC;
(b) Q. Xiang, J. Yu and M. Jaroniec, Chem. Soc. Rev., 2012, 41, 782–796 RSC.
- T. Froeschl, U. Hoermann, P. Kubiak, G. Kucerova, M. Pfanzelt, C. K. Weiss, R. J. Behm, N. Husing, U. Kaiser, K. Lansfester and M. W. Mehrens, Chem. Soc. Rev., 2012, 41, 5313–5360 RSC.
- N. Serpone, A. V Emeline, S. Horikoshi, V. N. Kuznetsov and V. K. Ryabchuk, Photochem. Photobiol. Sci., 2012, 11, 1121–1150 CAS.
-
(a) L. G. Devi and R. Kavitha, Appl. Catal., B, 2013, 140–141, 559–587 CrossRef CAS PubMed;
(b) L. G. Devi and R. Kavitha, Mater. Chem. Phys., 2014, 143, 1300–1308 CrossRef CAS PubMed;
(c) R. Kavitha and L. G. Devi, J. Environ. Chem. Eng., 2014, 2, 857–867 CrossRef CAS PubMed.
-
(a) S. G. Kumar and L. G. Devi, J. Phys. Chem. A, 2011, 115, 13211–13241 CrossRef CAS PubMed;
(b) L. G. Devi and S. G. Kumar, Cent. Eur. J. Chem., 2011, 9, 959–961 CrossRef PubMed.
-
(a) J. Zhang, Y. Wu, M. Xing, S. A. K. Leghari and S. Sajjad, Energy Environ. Sci., 2010, 3, 715–726 RSC;
(b) M. V. Dozzi and E. Selli, J. Photochem. Photobiol., C, 2013, 14, 13–28 CrossRef CAS PubMed;
(c) M. Pelaez, N. T. Nolan, S. C. Pillai, M. K. Serry, P. Falaras, A. G. Kontos, P. S. M. Dunlop, J. W. J. Hamilton, J. A. Byrne, K. O. Shea, M. H. Entezari and D. D. Dionysiou, Appl. Catal., B, 2012, 125, 331–349 CrossRef CAS PubMed.
- N. Zhang, S. Q. Liu and Y. J. Xu, Nanoscale, 2012, 4, 2227–2238 RSC.
- Z. Y. Zhou, N. Tian, J. T. Li, I. Broadwell and S. G. Sun, Chem. Soc. Rev., 2011, 40, 4167–4185 RSC.
- H. Tada, M. Fujishima and H. Kobayashi, Chem. Soc. Rev., 2011, 40, 4232–4243 RSC.
- D. Ravelli, D. Dondi, M. Fagnoni and A. Albini, Chem. Soc. Rev., 2009, 38, 1999–2011 RSC.
- R. Beranek and H. Kisch, Photochem. Photobiol. Sci., 2008, 7, 40–48 CAS.
- C. Aprile, A. Corma and H. Garcia, Phys. Chem. Chem. Phys., 2008, 10, 769–783 RSC.
- A. S. Weber, A. M. Grady and R. T. Koodali, Catal. Sci. Technol., 2012, 2, 683–693 CAS.
- S. Fukuzumi and K. Ohkubo, Chem. Sci., 2013, 4, 561–574 RSC.
- G. Liu, J. C. Yu, G. Q. Lu and H. M. Cheng, Chem. Commun., 2011, 47, 6763–6783 RSC.
- G. Liu, L. Wang, H. G. Yang, H. M. Cheng and G. Q. Lu, J. Mater. Chem., 2010, 20, 831–843 RSC.
- A. Kudo and Y. Miseki, Chem. Soc. Rev., 2009, 38, 253–278 RSC.
- S. Bingham and W. A. Daoud, J. Mater. Chem., 2011, 21, 2041–2050 RSC.
- C. Z. Wen, H. B. Jiang, S. Z. Qiao, H. G. Yang and G. Q. Lu, J. Mater. Chem., 2011, 21, 7052–7061 RSC.
- A. A. Ismail and D. W. Bahenmann, J. Mater. Chem., 2011, 21, 11686–11707 RSC.
- K. Lv, B. Cheng, J. Yu and G. Liu, Phys. Chem. Chem. Phys., 2012, 14, 5349–5362 RSC.
- L. Li, P. A. Salvador and G. S. Rohrer, Nanoscale, 2014, 6, 24–42 RSC.
- C. W. Dunnill and I. P. Parkin, Dalton Trans., 2011, 40, 1635–1640 RSC.
- S. Hu, F. Li and Z. Fan, Appl. Surf. Sci., 2011, 258, 1249–1255 CrossRef CAS PubMed.
- M. R. Hoffmann, S. T. Martin, W. Choi and D. W. Bahnemann, Chem. Rev., 1995, 95, 69–96 CrossRef CAS.
- A. Fujishima, T. N. Rao and D. A. Tryk, J. Photochem. Photobiol., C, 2000, 1, 1–21 CrossRef CAS.
- X. F. Chen, X. C. Wang, Y. D. Hou, J. H. Huang, L. Wu and X. Z. Fu, J. Catal., 2008, 255, 59–67 CrossRef CAS PubMed.
- H. Irie, Y. Wantanabe and K. Hasimoto, J. Phys. Chem. B, 2003, 107, 5483–5486 CrossRef CAS.
- I. Nakamuru, N. Negishi, S. Kutsuna, T. Ihara, S. Sugihara and K. Takeuchi, J. Mol. Catal. A: Chem., 2000, 161, 205–212 CrossRef.
- E. Elgniji, M. Ksibi and E. Elaloui, J. Ind. Eng. Chem., 2012, 18, 178–182 CrossRef PubMed.
- Y. Zhao, X. Qiu and C. Burda, Chem. Mater., 2008, 20, 2629–2636 CrossRef CAS.
- J. Fang, F. Wang, K. Qian, H. Bao, Z. Jiang and W. Huang, J. Phys. Chem. C, 2008, 112, 18150–18156 CAS.
- M. Bellardita, M. Addamo, A. D. Paola, L. Palmisano and A. M. Venezia, Phys. Chem. Chem. Phys., 2009, 11, 4084–4093 RSC.
- S. Hotchandani and P. V. Kamat, J. Phys. Chem., 1992, 96, 6834–6839 CrossRef CAS.
- N. Serpone, P. Maruthamuthu, P. Pichat, E. Pelizzetti and H. Hidaka, J. Photochem. Photobiol., A, 1995, 85, 247–255 CrossRef CAS.
- A. D. Paola, L. Palmisano, M. Derrigo and V. Augugliaro, J. Phys. Chem. B, 1997, 101, 876–883 CrossRef.
- M. Yan, F. Chen, J. Zhang and M. Anpo, J. Phys. Chem. B, 2005, 109, 8673–8678 CrossRef CAS PubMed.
- R. Asahi, T. Morikawa, T. Ohwaki, K. Aoki and Y. Taga, Science, 2001, 293, 269–271 CrossRef CAS PubMed.
- C. Di Valentin, G. Pacchioni and A. Selloni, Phys. Rev. B: Condens. Matter Mater. Phys., 2004, 70, 085116 CrossRef.
- N. Serpone, J. Phys. Chem. B, 2006, 110, 24287–24293 CrossRef CAS PubMed.
- N. T. Nolan, D. W. Synnott, M. K. Seery, S. J. Hinder, A. V. Wassenhoven and S. C. Pillai, J. Hazard. Mater., 2012, 211–212, 88–94 CrossRef CAS PubMed.
- V. Etacheri, M. K. Seery, S. J. Hinder and S. C. Pillai, Chem. Mater., 2010, 22, 3843–3853 CrossRef CAS.
- S. Somekawa, Y. Kusumoto, M. Ikeda, B. Ahmmad and Y. Horie, Catal. Commun., 2008, 9, 437–440 CrossRef CAS PubMed.
- S. Maret, E. A. Bergin and C. J. Lada, Nature, 2006, 442, 425–427 CrossRef CAS PubMed.
- C. Chen, H. Bai and C. Chang, J. Phys. Chem. C, 2007, 111, 15228–15235 CAS.
- A. Kafizas, C. Crick and I. P. Parkin, J. Photochem. Photobiol., A, 2010, 216, 156–166 CAS.
- M. Miyauchi, A. Ikezawa, H. Tobimatsu, H. Irie and K. Hashimoto, Phys. Chem. Chem. Phys., 2004, 6, 865–870 RSC.
- J. Qian, G. Cui, M. Jing, Y. Wang, M. Zhang and J. Yang, Int. J. Photoenergy, 2012, 198497 Search PubMed.
- Y. Wang, C. X. Feng, M. Zhang, J. J. Yang and Z. J. Zhang, Appl. Catal., B, 2011, 104, 268–274 CrossRef CAS PubMed.
- Y. Wang, C. Feng, M. Zhang, J. Yang and Z. Zhang, Appl. Catal., B, 2010, 100, 80–94 Search PubMed.
- S. Zhang, W. Li, Z. Jin, J. Yang, J. Zhang, Z. Du and Z. Zhang, J. Solid State Chem., 2004, 177, 1365–1371 CrossRef CAS PubMed.
- Y. Nosaka, M. Matsushita, J. Nishino and A. Y. Nosaka, Sci. Technol. Adv. Mater., 2005, 6, 143–148 CrossRef CAS PubMed.
- D. Wu, M. Long, W. Cai, C. Chen and Y. Wu, J. Alloys Compd., 2010, 502, 289–294 CrossRef CAS PubMed.
- A. Kachina, E. Puzenat, S. Chikh, C. Geantet, P. Delichere and P. Afanasiev, Chem. Mater., 2012, 24, 636–642 CrossRef CAS.
- V. M. Khomenka, K. Langer, H. Rager and A. Fett, Phys. Chem. Miner., 1998, 25, 338–346 CrossRef.
-
(a) Y. X. Zhang, G. H. Li, Y. X. Jin, Y. Zhang, J. Zhang and L. D. Zhang, Chem. Phys. Lett., 2002, 365, 300–304 CrossRef CAS;
(b) J. Yu, W. Liu and H. Yu, Cryst. Growth Des., 2008, 8, 930–934 CrossRef CAS;
(c) J. Yu, G. Dai and B. Cheng, J. Phys. Chem. C, 2010, 114, 19378–19385 CrossRef CAS.
-
(a) P. D. Cozzoli, A. Kornowski and H. Weller, J. Am. Chem. Soc., 2003, 125, 14539–14548 CrossRef CAS PubMed;
(b) K. Lv, J. Yu, J. Fan and M. Jaroniec, Cryst. Growth Des., 2011, 13, 7044–7148 CAS.
-
(a) W. Zhou, X. Liu, J. Cui, D. Liu, J. Li, H. Jiang, J. Wang and H. Liu, CrystEngComm, 2011, 13, 4557–4563 RSC;
(b) J. Yu and J. Zhang, Dalton Trans., 2010, 5860–5897 RSC.
- C. C. Hu, T. C. Hsu and L. H. Kao, Int. J. Photoenergy, 2012, 391958 Search PubMed.
- J. Wang, W. Zhu, Y. Zhang and S. Liu, J. Phys. Chem. C, 2007, 111, 1010–1014 CAS.
- Z. Zhao, J. Fan, J. Wang and R. Li, Catal. Commun., 2012, 21, 32–37 CrossRef CAS PubMed.
- Y. Wang and D. J. Doren, Solid State Commun., 2005, 136, 186–189 CrossRef CAS PubMed.
- J. Y. Lee, J. Park and J. H. Cho, Appl. Phys. Lett., 2005, 87, 011904 CrossRef PubMed.
- S. In, P. C. K. Vesborg, B. L. Abrams, Y. Hou and I. Chorkendorff, J. Photochem. Photobiol., A, 2011, 222, 258–262 CrossRef CAS PubMed.
- Z. Jiang, F. Yang, N. Luo, B. T. T. Chu, D. Sun, H. Shi, T. Xiao and P. P. Edwards, Chem. Commun., 2008, 6372–6374 RSC.
- Q. Wang, X. Yang, X. Wang, M. Huang and J. Hou, Electrochim. Acta, 2012, 62, 158–162 CrossRef CAS PubMed.
- Y. Lai, J. Huang, H. Zhang, V. Subramaniam, Y. Tang, D. Gong, L. Sundar, L. Sun, Z. Chen and C. Lin, J. Hazard. Mater., 2010, 184, 855–863 CrossRef CAS PubMed.
- M. Sathish, B. Viswanathan and R. P. Viswanath, Appl. Catal., B, 2007, 74, 307–312 CrossRef CAS PubMed.
- Y. N. Huo, Y. Jin, J. Zhu and H. X. Li, Appl. Catal., B, 2009, 89, 543–550 CrossRef CAS PubMed.
- H. Z. Zhang and J. F. Banfield, J. Mater. Res., 2000, 15, 437–448 CrossRef CAS.
- T. Osaki, K. Nagashima, K. Watari and K. Tajiri, J. Non-Cryst. Solids, 2007, 353, 2436–2442 CrossRef CAS PubMed.
- D. P. Subagio, M. Srinivasan, M. Lim and T. T. Lim, Appl. Catal., B, 2010, 95, 414–422 CrossRef CAS PubMed.
- H. J. Cho, P. G. Hwang and D. Jung, J. Phys. Chem. Solids, 2011, 72, 1462–1466 CrossRef CAS PubMed.
- G. Liu, L. Wang, C. Sun, Z. Chen, X. Yan, L. Cheng, H. M. Cheng and G. Q. M. Lu, Chem. Commun., 2009, 1383–1385 RSC.
- T. Sasaki, M. Watanabe, H. Hashizume, H. Yamada and H. Nakazawa, J. Am. Chem. Soc., 1996, 118, 8329–8335 CrossRef CAS.
- G. Liu, L. Wang, C. Sun, X. Yan, X. Wang, Z. Chen, S. C. Smith, H. M. Cheng and G. Q. Lu, Chem. Mater., 2009, 21, 1266–1274 CrossRef CAS.
- J. Fu, Y. Tian, B. Chang, F. Xi and X. Dong, Chem.–Eng. J., 2013, 219, 155–161 CrossRef CAS PubMed.
- Y. Cheng, Y. Huang, P. D. Kanhere, V. P. Subramaniam, D. Gong and S. Zhang, Chem.–Eur. J., 2011, 17, 2575–2578 CrossRef CAS PubMed.
- Z. Xiong and X. Zhao, J. Am. Chem. Soc., 2012, 134, 5754–5757 CrossRef CAS PubMed.
- H. Tong, S. Ouyany, Y. Bi, N. Umezawa, M. Oshikiri and J. Ye, Adv. Mater., 2012, 24, 229–251 CrossRef CAS PubMed.
- Z. Lin, A. Orlov, R. M. Lambert and M. C. Payne, J. Phys. Chem. B, 2005, 109, 20948–20952 CrossRef CAS PubMed.
- K. M. Parida, S. Pany and B. Naik, Int. J. Hydrogen Energy, 2013, 38, 3545–3553 CrossRef CAS PubMed.
- X. Wang, J. C. Yu, C. Ho, Y. Hou and X. Fu, Langmuir, 2005, 21, 2552–2559 CrossRef CAS PubMed.
- J. Du, G. Zhao, Y. Shi, H. Yang, Y. Li, G. Zhu, Y. Mao, R. Sa and W. Wang, Appl. Surf. Sci., 2013, 273, 278–286 CrossRef CAS PubMed.
- V. J. Babu, M. K. Kumar, A. S. Nair, T. L. Kheng, S. I. Allakhverdiev and S. Ramakrishna, Int. J. Hydrogen Energy, 2012, 37, 8897–8904 CrossRef CAS PubMed.
- S. Wang, J. Xu, H. Ding, S. Pan, Y. Zhang and G. Li, CrystEngComm, 2012, 14, 7672–7679 RSC.
- E. Hosono, S. Fujihara, K. Kakiuchi and H. Imai, J. Am. Chem. Soc., 2004, 126, 7790–7791 CrossRef CAS PubMed.
- F. P. Rotzinger and M. Gratzel, Inorg. Chem., 1987, 26, 3704–3708 CrossRef CAS.
- L. Kavan, B. O'Regan, A. Kay and M. Gratzel, J. Electroanal. Chem., 1993, 346, 291–307 CrossRef CAS.
- T. Guillet, R. Grousson, V. Voliotis, X. L. Wang and M. Ogura, Phys. Rev. B: Condens. Matter, 2003, 68, 045319 CrossRef.
- J. M. Wu and B. Qi, J. Phys. Chem. C, 2007, 111, 666–673 CAS.
- S. Yin and T. Sato, J. Mater. Chem., 2005, 15, 4584–4587 RSC.
- J. Wu, B. Haung, M. Wang and A. Osaka, J. Am. Ceram. Soc., 2006, 89, 2660–2663 CrossRef CAS PubMed.
-
(a) A. G. Kontos, M. Pelaez, V. Likodimos, N. Vaenas, D. D. Dionysiou and P. Falaras, Photochem. Photobiol. Sci., 2011, 10, 350–354 RSC;
(b) P. Zhou, J. Yu and Y. Wang, Appl. Catal., B, 2013, 142–143, 45–53 CrossRef CAS PubMed;
(c) X. Wang, K. Wang, K. Feng, F. Chen, H. Yu and J. Yu, J. Mol. Catal. A: Chem., 2014, 391, 92–98 CrossRef CAS PubMed;
(d) J. Yu, M. Zhou, B. Cheng and X. Zhao, J. Mol. Catal. A: Chem., 2006, 246, 179–184 Search PubMed;
(e) M. Zhou and J. Yu, J. Hazard. Mater., 2008, 152, 1229–1236 CrossRef CAS PubMed.
- K. Shankar, K. C. Tep, G. K. Mor and C. A. Grimes, J. Phys. D: Appl. Phys., 2006, 39, 2361 CrossRef CAS.
- Y. C. Nah, I. Paramasivam and P. Schmuki, ChemPhysChem, 2010, 11, 2698–2713 CrossRef CAS PubMed.
- A. E. R. Mohamed and S. Rohani, Energy Environ. Sci., 2011, 4, 1065–1086 CAS.
- J. Yuan, E. Wang, Y. Chen, W. Yang, J. Yao and Y. Cao, Appl. Surf. Sci., 2011, 257, 7335–7342 CrossRef CAS PubMed.
- E. Finazzi, C. D. Valentin and G. Pacchioni, J. Phys. Chem. C, 2009, 113, 220–228 CAS.
- D. Chen, D. Yang, Q. Wang and Z. Y. Jiang, Ind. Eng. Chem. Res., 2006, 45, 4110–4116 CrossRef CAS.
- H. Geng, S. Yin, X. Yang, Z. Shuai and B. Liu, J. Phys.: Condens. Matter, 2006, 18, 87–96 CrossRef CAS.
- Y. Huang, W. Ho, Z. Ai, X. Song, L. Zhang and S. Lee, Appl. Catal., B, 2009, 89, 398–405 CrossRef CAS PubMed.
- A. M. Czoska, S. Livraghi, M. C. Paganini, E. Giamello, C. D. Valentin and G. Pacchioni, Phys. Chem. Chem. Phys., 2011, 13, 136–143 RSC.
- S. C. Moon, H. Mametsuka, S. Tabata and E. Suzuki, Catal. Today, 2000, 58, 125–132 CrossRef CAS.
- S. In, A. Orlov, R. Berg, F. Garcia, S. P. Jimenez, M. S. Tikhov, D. S. Wright and R. M. Lambert, J. Am. Chem. Soc., 2007, 129, 13790–13791 CrossRef CAS PubMed.
- B. Gao, Y. Ma, Y. Cao, W. Yang and J. Yao, J. Phys. Chem. B, 2006, 110, 14391–14397 CrossRef CAS PubMed.
- O. Carp, C. L. Huisman and A. Reller, Prog. Solid State Chem., 2004, 32, 33–177 CrossRef CAS PubMed.
- G. Liu, L. Yin, J. Wang, P. Niu, C. Zhen, Y. Xie and H. Cheng, Energy Environ. Sci., 2012, 5, 9603–9610 CAS.
- X. B. Chen, L. Liu, P. Y. Yu and S. S. Mao, Science, 2011, 331, 746–750 CrossRef CAS PubMed.
- D. Chen, Z. Jiang, J. Geng, Q. Wang and D. Yang, Ind. Eng. Chem. Res., 2007, 46, 2741–2746 CrossRef CAS.
- Y. L. Pang and A. Z. Abdullah, Chem.–Eng. J., 2013, 214, 129–138 CrossRef CAS PubMed.
- R. Vinu and G. Madras, Environ. Sci. Technol., 2009, 43, 473–479 CrossRef CAS.
- F. Dong, W. Zhao and Z. Wu, Nanotechnology, 2008, 19, 1–10 Search PubMed.
- X. Yang, C. Cao, L. Erickson, K. Hohn, R. Maghirang and K. Klabunde, J. Catal., 2008, 260, 128–133 CrossRef CAS PubMed.
- J. Yang, H. Bai, X. Tan and J. Lian, Appl. Surf. Sci., 2006, 253, 1988–1994 CrossRef CAS PubMed.
- K. Suriye, P. Praserthdam and B. Jongsomjit, Appl. Surf. Sci., 2007, 253, 3849–3855 CrossRef CAS PubMed.
- M. Antonopoulou, A. Giannakas and I. Konstantinou, Int. J. Photoenergy, 2012, 520123 Search PubMed.
- Y. Wu, M. Xing, B. Tian, J. Zhang and F. Chen, Chem.–Eng. J., 2010, 162, 710–717 CrossRef CAS PubMed.
- C. Minero, G. Mariella, V. Maurino, D. Vione and E. Pelizzetti, Langmuir, 2000, 16, 8964–8972 CrossRef CAS.
- C. Minero, G. Mariella, V. Maurino, D. Vione and E. Pelizzetti, Langmuir, 2000, 16, 2632–2641 CrossRef CAS.
- Z. He, W. Que, J. Chen, X. Yin, Y. He and J. Ren, ACS Appl. Mater. Interfaces, 2012, 4, 6816–6826 CAS.
- J. Wang, D. N. Tafen, J. P. Lewis, Z. L. Hong, A. Manivannan, M. J. Zhi, M. Li and N. O. Wu, J. Am. Chem. Soc., 2009, 131, 12290–12297 CrossRef CAS PubMed.
- W. Chen, Z. L. Fan, B. Zhang, G. J. Ma, K. Takanabe, X. X. Zhang and Z. P. Lai, J. Am. Chem. Soc., 2011, 133, 14896–14899 CrossRef CAS PubMed.
- C. X. Wang, L. W. Yin, L. Y. Zhang, N. N. Liu, N. Lun and Y. X. Qi, ACS Appl. Mater. Interfaces, 2010, 2, 3373–3377 CAS.
- L. F. Zhang, R. Chandrasekar, J. Y. Howe, M. K. West, N. E. Hedin, W. J. Arbegast and H. Fong, ACS Appl. Mater. Interfaces, 2009, 1, 987–991 CAS.
- P. G. Hu, G. J. Du, W. J. Zhou, J. J. Cui, J. J. Lin, H. Liu, D. Liu, J. Y. Wang and S. W. Chen, ACS Appl. Mater. Interfaces, 2010, 2, 3263–3269 CAS.
- Z. Ai, Z. Gao, K. Su, W. Ho and L. Zhang, Catal. Commun., 2012, 29, 189–193 CrossRef CAS PubMed.
- S. Guo, S. Han, H. Mao, C. Wu, L. Jia, B. Chi, J. Pu and L. Jian, J. Alloys Compd., 2012, 544, 50–54 CrossRef CAS PubMed.
- Y. Sohn, Appl. Surf. Sci., 2010, 257, 1692–1697 CrossRef CAS PubMed.
- Q. Xiao, L. Ouyang, L. Gao and C. Yao, Appl. Surf. Sci., 2011, 257, 3652–3656 CrossRef CAS PubMed.
- V. Etacheri, M. K. Seery, S. J. Hinder and S. C. Pillai, Inorg. Chem., 2012, 51, 7164–7173 CrossRef CAS PubMed.
- X. Tianhua, S. Chen-lu, L. Yong and H. J. Gao-rong, J. Zhejiang Univ., Sci., B, 2006, 7, 299–303 CrossRef PubMed.
- M. D. Segall, P. J. D. Lindan, M. J. Probert, C. J. Pickard, P. J. Hasnip, S. J. Clark and M. C. Payne, J. Phys.: Condens. Matter, 2002, 14, 2717 CrossRef CAS.
- L. Kavan, M. Gratzel, S. E. Gilbert, C. Klemenz and H. J. Scheel, J. Am. Chem. Soc., 1996, 118, 6716–6723 CrossRef CAS.
- G. Zhang, X. Ding, F. He, X. Yu, J. Zhou, Y. Hu and J. Xie, J. Phys. Chem. Solids, 2008, 69, 1102–1106 CrossRef CAS PubMed.
- H. X. Li, J. X. Li and Y. N. Huo, J. Phys. Chem. B, 2006, 110, 1559–1565 CrossRef CAS PubMed.
- A. E. Giannakas, M. Antonopoulou, Y. Deligiannakis and I. Konstantinou, Appl. Catal., B, 2013, 140–141, 636–645 CrossRef CAS PubMed.
- L. Pan, J. J. Zou, S. Wang, Z. F. Haung, X. Zhang and L. Wang, Appl. Surf. Sci., 2013, 268, 252–258 CrossRef CAS PubMed.
- G. Liu, X. Li, J. Zhao, H. Hidaka and N. Serpone, Environ. Sci. Technol., 2000, 34, 3982–3990 CrossRef CAS.
- L. Pan, J. J. Zou, S. Wang, X. Y. Liu, X. Zhang and L. Wang, ACS Appl. Mater. Interfaces, 2012, 4, 1650–1655 CAS.
- Y. Cong, B. Z. Tian and J. L. Zhang, Appl. Catal., B, 2011, 101, 376–381 CrossRef CAS PubMed.
- V. M. Flores, D. W. Bahnemann and T. Ohno, Appl. Catal., B, 2011, 103, 99–108 CrossRef PubMed.
- P. L. Zhang, S. Yin and T. Sato, Appl. Catal., B, 2011, 103, 462–469 CrossRef CAS PubMed.
- R. Jaiswal, N. Patel, D. C. Kothari and A. Miotello, Appl. Catal., B, 2012, 126, 47–54 CrossRef CAS PubMed.
- Y. Cong, J. L. Zhang, F. Chen and M. Anpo, J. Phys. Chem. C, 2007, 111, 6976–6982 CAS.
- S. Liu, T. Xie, Z. Chan and J. Wu, Appl. Surf. Sci., 2009, 255, 8587–8592 CrossRef CAS PubMed.
- R. Dholam, N. Patel and A. Miotello, Int. J. Hydrogen Energy, 2011, 36, 6519–6528 CrossRef CAS PubMed.
- Y. Hu, X. Zhang and C. Wei, Res. Chem. Intermed., 2010, 36, 95–101 CrossRef CAS PubMed.
- A. W. Xu, Y. Gao and H. Q. Liu, J. Catal., 2002, 207, 151–157 CrossRef CAS.
- R. Arroyo, G. Corboda, J. Padilla and V. H. Lara, Mater. Lett., 2002, 54, 397–402 CrossRef CAS.
- I. Othman, R. M. Mohamed and F. M. Ibrahem, J. Photochem. Photobiol., A, 2007, 189, 80–85 CrossRef CAS PubMed.
- L. G. Devi, N. Kottam and S. G. Kumar, J. Phys. Chem. C, 2009, 113, 15593–15601 CAS.
- L. G. Devi, N. Kottam, S. G. Kumar and K. S. A. Raju, Catal. Lett., 2009, 131, 612–617 CrossRef.
- L. G. Devi, S. G. Kumar, B. N. Murthy and N. Kottam, Catal. Commun., 2009, 10, 794–798 CrossRef CAS PubMed.
- L. G. Devi, N. Kottam, B. N. Murthy and S. G. Kumar, J. Mol. Catal. A: Chem., 2010, 328, 44–52 CrossRef CAS PubMed.
- B. Naik and K. M. Parida, Ind. Eng. Chem. Res., 2010, 49, 8339–8346 CrossRef CAS.
- X. Shen, J. Guo, Z. Liu and S. Xie, Appl. Surf. Sci., 2008, 254, 4726–4731 CrossRef CAS PubMed.
- Y. Sakatani, D. Grosso, L. N. C. Boissiere, G. J. A. A. de Soler-Illia and C. Sanchez, J. Mater. Chem., 2006, 16, 77–82 RSC.
- Z. Bian, J. Zhu, S. Wang, Y. Cao, X. Qian and H. Li, J. Phys. Chem. C, 2008, 112, 6258–6262 CAS.
- H. Zhang, Y. Liang, X. Wu and H. Zheng, Mater. Res. Bull., 2012, 47, 2188–2192 CrossRef CAS PubMed.
- C. D. Valentin, G. Pacchioni, H. Onishi and A. Kudo, Chem. Phys. Lett., 2009, 469, 166–171 CrossRef PubMed.
- S. A. Bilmes, P. Mandelbaum, F. Alvarez and N. M. Victoria, J. Phys. Chem. B, 2000, 104, 9851–9858 CrossRef CAS.
- W. Gopel, G. Rocker and R. Feierabend, Phys. Rev. B: Condens. Matter Mater. Phys., 1983, 28, 3427–3438 CrossRef CAS.
- H. Zhang, G. Mia, X. Ma, B. Wang and H. Zheng, Mater. Res. Bull., 2014, 55, 26–32 CrossRef CAS PubMed.
- H. Zhang, K. Tan, H. Zheng, Y. Gu and W. F. Zhang, Mater. Chem. Phys., 2011, 125, 156–160 CrossRef CAS PubMed.
- R. A. Lucky and P. A. Charpentier, Appl. Catal., B, 2010, 96, 516–523 CrossRef CAS PubMed.
- K. M. N. Parida and B. Naik, J. Colloid Interface Sci., 2009, 333, 269–276 CrossRef CAS PubMed.
- J. Zhu, J. Ren, Y. Huo, Z. Bian and H. Li, J. Phys. Chem. C, 2007, 111, 18965–18969 CAS.
- F. K. Han, V. S. R. Kambala, M. Srinivasan, D. Rajarathnam and R. Naidu, Appl. Catal., A, 2009, 359, 25–40 CrossRef CAS PubMed.
- M. M. Joshi, N. K. Labhsetwar, P. A. Mangrulkar, S. N. Tijare, S. P. Kamble and S. S. Rayalu, Appl. Catal., A, 2009, 357, 26–33 CrossRef CAS PubMed.
- B. Gao, X. Luo, H. Fu, B. Lin, Y. Chen and Z. Gu, Mater. Res. Bull., 2013, 48, 587–594 CrossRef CAS PubMed.
- T. M. Breault and B. M. Bartlett, J. Phys. Chem. C, 2013, 117, 8611–8618 CAS.
- M. A. Rauf and S. S. Ashraf, Chem.–Eng. J., 2009, 151, 10–18 CrossRef CAS PubMed.
- X. T. Pian, B. Z. Lin, Y. L. Chen, J. D. Kaung, K. Z. Zhang and L. M. Fu, J. Phys. Chem. C, 2011, 115, 6531–6539 CAS.
- X. Chen and S. S. Mao, Chem. Rev., 2007, 107, 2891–2959 CrossRef CAS PubMed.
- J. F. Baumard and E. Tani, J. Chem. Phys., 1977, 67, 857–860 CrossRef CAS PubMed.
- T. Cottineau, N. Bealu, P. A. Gross, S. N. Pronkin, N. Keller, E. R. Savinova and V. Keller, J. Mater. Chem. A, 2013, 1, 2151–2160 CAS.
- R. Long and N. J. English, Chem. Phys. Lett., 2009, 478, 175–179 CrossRef CAS PubMed.
- R. Long and N. J. English, Appl. Phys. Lett., 2009, 94, 132102 CrossRef PubMed.
- M. Li, J. Zhang and Y. Zhang, Catal. Commun., 2012, 29, 175–179 CrossRef CAS PubMed.
- X. G. Ma, Y. Wu, Y. H. Lu, Y. J. Wang and Y. F. Zhu, J. Phys. Chem. C, 2011, 115, 16963–19969 CAS.
- J. Zhang, J. H. Xi and Z. G. Ji, J. Mater. Chem., 2012, 22, 17700–17708 RSC.
- X. Cheng, X. Yu and Z. Xing, J. Colloid Interface Sci., 2012, 372, 1–5 CrossRef CAS PubMed.
- H. Gao, B. Lu, F. Liu, Y. Liu and X. Zhao, Int. J. Photoenergy, 2012, 453018 Search PubMed.
- E. Wang, T. He, L. Zhao, Y. Chen and Y. Cao, J. Mater. Chem., 2011, 21, 144–150 RSC.
- Y. A. Cao, W. S. Yang, W. F. Zhang, G. Z. Liu and P. Yue, New J. Chem., 2004, 28, 218–222 RSC.
- Q. Kang, B. Yuan, J. Xu and M. Fu, Catal. Lett., 2011, 141, 1371–1377 CrossRef CAS.
- D. E. Gu, B. C. Yang and Y. D. Hu, Catal. Commun., 2008, 9, 1472–1476 CrossRef CAS PubMed.
- E. Wang, P. Zhang, Y. Chen, Z. Liu, T. He and Y. Cao, J. Mater. Chem., 2012, 22, 14443–14449 RSC.
- S. S. Thind, G. Wu and A. Chen, Appl. Catal., B, 2012, 111–112, 38–45 CrossRef CAS PubMed.
- A. Kubacka, G. Colon and M. Garcia, Appl. Catal., B, 2010, 95, 238–244 CrossRef CAS PubMed.
- A. Kubacka, M. Garcia and G. Colon, J. Catal., 2008, 254, 272–284 CrossRef CAS PubMed.
- J. Li, J. Xu, W. Dai, H. Li and K. Fan, Appl. Catal., B, 2008, 82, 233–243 CrossRef CAS PubMed.
- Y. Wang, B. Wu and Q. Xu, Appl. Catal., B, 2005, 59, 139–146 CrossRef PubMed.
- M. T. Tsai, J. Non-Cryst. Solids, 2002, 298, 116–130 CrossRef CAS.
- Y. Shen, T. Xiong, T. Li and K. Yang, Appl. Catal., B, 2008, 83, 177–185 CrossRef CAS PubMed.
- A. M. Marquez, J. J. Plata, Y. Ortega, J. F. Sanz, G. Colon, A. Kubacka and M. F. Garcia, J. Phys. Chem. C, 2012, 116, 18759–18767 CAS.
- J. Gong, W. Pu, C. Yang and J. Zhang, Chem.–Eng. J., 2012, 209, 94–101 CrossRef CAS PubMed.
- H. G. Yu, H. Irie, Y. Shimodajra, Y. Hosogi, Y. Kuroda, M. Miyauchi and K. Hashimoto, J. Phys. Chem. C, 2010, 114, 16481–16487 CAS.
- J. J. Xu, Y. H. Ao, D. G. Fu and C. W. Yuan, Colloids Surf., A, 2009, 334, 107–111 CrossRef CAS PubMed.
- K. T. Ranjit, I. Willner, S. H. Bossmann and A. M. Braun, J. Catal., 2001, 204, 305–313 CrossRef CAS.
- K. T. Ranjit, I. Willner, S. H. Bossmann and A. M. Braun, Environ. Sci. Technol., 2001, 35, 1544–1549 CrossRef CAS.
- B. M. Reddy, P. M. Sreekanth, E. P. Reddy, Y. Yamada, Q. Xu, H. Sakurai and T. Kobayashi, J. Phys. Chem. B, 2002, 106, 5695–5700 CrossRef CAS.
- C. Liu, X. Tang, C. Mo and Z. Qiang, J. Solid State Chem., 2008, 181, 913–919 CrossRef CAS PubMed.
- C. Wang, Y. Ao, P. Wang, J. Hou and J. Qian, Powder Technol., 2011, 210, 203–207 CrossRef CAS PubMed.
- P. F. Ji, J. L. Zhang, F. Chen and M. Anpo, Appl. Catal., B, 2009, 85, 148–154 CrossRef CAS PubMed.
- P. F. Ji, J. L. Zhang, F. Chen and M. Anpo, J. Phys. Chem. C, 2008, 112, 17809–17813 CAS.
- P. F. Ji, L. Z. Wang, F. Chen and J. L. Zhang, ChemCatChem, 2010, 2, 1552–1554 CrossRef CAS.
- B. M. Reddy, A. Khan, Y. Yamada, T. Kobayashi, S. Loridant and J. Volta, J. Phys. Chem. B, 2003, 107, 5162–5167 CrossRef CAS.
- J. Lin and J. C. Yu, J. Photochem. Photobiol., A, 1998, 116, 63–67 CrossRef CAS.
- J. Fang, X. Bi, D. Si, Z. Jiang and W. Huang, Appl. Surf. Sci., 2007, 253, 8952–8961 CrossRef CAS PubMed.
- Z. Liu, B. Guo, L. Hong and H. Jiang, J. Phys. Chem. Solids, 2005, 66, 161–167 CrossRef CAS PubMed.
- J. Yang, J. Dai and J. Li, Appl. Surf. Sci., 2011, 257, 8965–8973 CrossRef CAS PubMed.
- J. Arbiol, J. Cerda, G. Dezanneau, A. Cirera, F. Peiro, A. Cornet and J. R. Morante, J. Appl. Phys., 2002, 92, 853–861 CrossRef CAS PubMed.
- R. Kralchevska, M. Milanova, D. Hristov, A. Pintar and D. Todorovsky, Mater. Res. Bull., 2012, 47, 2165–2177 CrossRef CAS PubMed.
- Y. Ma, J. Zhang, B. Tian, F. Chen and L. Wang, J. Hazard. Mater., 2010, 182, 386–393 CrossRef CAS PubMed.
- A. W. Xu, Y. Gao and H. Q. Liu, J. Catal., 2002, 207, 151–157 CrossRef CAS.
- L. G. Devi, B. N. Murthy and S. G. Kumar, Chemosphere, 2009, 76, 1163–1166 CrossRef CAS PubMed.
- L. G. Devi, N. Kottam, S. G. Kumar and K. E. Rajashekhar, Cent. Eur. J. Chem., 2010, 8, 142–148 CrossRef.
- L. G. Devi and S. G. Kumar, Appl. Surf. Sci., 2011, 257, 2779–2790 CrossRef CAS PubMed.
- Y. Pleskov, Sov. Electrochem., 1981, 17, 1–25 Search PubMed.
- L. G. Devi and S. G. Kumar, Appl. Surf. Sci., 2012, 261, 137–146 CrossRef CAS PubMed.
- J. Xu, Y. Ao, D. Fu and C. Yuan, J. Colloid Interface Sci., 2008, 328, 447–451 CrossRef CAS PubMed.
- E. Borgarello, J. Kiwi, M. Gratzel, E. Pelizzetti and M. Visca, J. Am. Chem. Soc., 1982, 104, 2996–3002 CrossRef CAS.
- F. B. Li, X. Z. Li, M. F. Hou, K. W. Cheah and W. C. H. Choy, Appl. Catal., A, 2005, 285, 181–189 CrossRef CAS PubMed.
- J. Shi, H. Cui, X. Zong, S. Chen, J. Chen, B. Xu, W. Yang, L. Wang and M. Fu, Appl. Catal., A, 2012, 435–436, 86–92 CrossRef CAS PubMed.
- Y. Cui, L. Liu, B. Li, X. Zhou and N. Xu, J. Phys. Chem. C, 2010, 114, 2434–2439 CAS.
- H. G. Yang and H. C. Zeng, J. Phys. Chem. B, 2004, 108, 3492–3495 CrossRef CAS.
- X. W. Lou, C. L. Yuan and L. A. Archer, Small, 2007, 3, 261–265 CrossRef CAS PubMed.
- J. Li and H. C. Zeng, J. Am. Chem. Soc., 2007, 129, 15839–15847 CrossRef CAS PubMed.
- Y. Xie and C. Yuan, Mater. Res. Bull., 2004, 39, 533–543 CrossRef CAS PubMed.
- F. B. Li, X. Z. Li, M. F. Hou, K. W. Cheah and W. C. H. Choy, Appl. Catal., A, 2005, 285, 181–189 CrossRef CAS PubMed.
- J. W. Shi, X. Yan, H. J. Cui, X. Zong, M. L. Fu, S. Chen and L. Wang, J. Mol. Catal. A: Chem., 2012, 356, 53–60 CrossRef CAS PubMed.
- H. Liu, G. Liu, G. Xie, M. Zhang, Z. Hou and Z. He, Appl. Surf. Sci., 2011, 257, 3728–3732 CrossRef CAS PubMed.
- Z. B. Zhang, C. C. Wang, R. Zakaria and J. Y. Ying, J. Phys. Chem. B, 1998, 102, 10871 CrossRef CAS.
- B. Singhal, A. Porwal, A. Sharma, R. Ameta and S. C. Ameta, J. Photochem. Photobiol., A, 1997, 108, 85–88 CrossRef CAS.
- K. L. Lv, J. Hu, X. Li and M. Li, J. Mol. Catal. A: Chem., 2012, 356, 78–84 CrossRef CAS PubMed.
- K. L. Lv, J. G. Yu, K. J. Deng, X. H. Li and M. Li, J. Phys. Chem. Solids, 2010, 71, 519–522 CrossRef CAS PubMed.
- Y. M. Xu, K. L. Lv, Z. G. Xiong, W. H. Leng, W. P. Du, D. Liu and X. J. Xue, J. Phys. Chem. C, 2007, 111, 19024–19032 CAS.
- K. L. Lv, X. F. Li, K. J. Deng, J. Sun, X. H. Li and M. Li, Appl. Catal., B, 2010, 95, 383–392 CrossRef CAS PubMed.
- Y. Wang, Y. Huang, W. Ho, L. Zhang, Z. Zou and S. Lee, J. Hazard. Mater., 2009, 169, 77–87 CrossRef CAS PubMed.
- A. Scafani and J. M. Hermann, J. Phys. Chem., 1996, 100, 13655–13661 CrossRef.
- M. Andersson, L. Osterlund, S. Ljungstrom and A. Palmqvist, J. Phys. Chem. B, 2002, 106, 10674–10679 CrossRef CAS.
- Y. Cong, J. L. Zhang, F. Chen, M. Anpo and D. N. He, J. Phys. Chem. C, 2007, 111, 10618–10623 CAS.
- X. Cheng, X. Yu and Z. Xing, Appl. Surf. Sci., 2012, 258, 7644–7650 CrossRef CAS PubMed.
- J. Liqiang, F. Honggang, W. Baiqi, W. Dejun, X. Baifu, L. Sudan and S. Jiazhang, Appl. Catal., B, 2006, 62, 282–291 CrossRef PubMed.
- J. Liqiang, S. Xiaojun, S. Jing, C. Weimin, X. Zili, D. Yaoguo and F. Honggang, Sol. Energy Mater. Sol. Cells, 2003, 79, 133–151 CrossRef.
- Q. Xu, D. V. Wellia, M. Alam Sk, K. H. Lim, J. S. C. Loo, D. W. Lia, R. Amal and T. T. Y. Tan, J. Photochem. Photobiol., A, 2010, 210, 181–187 CrossRef CAS PubMed.
- X. Yang, C. Cao, L. Erickson, K. Hohn, R. Maghirang and K. Klabunde, J. Catal., 2008, 260, 128–133 CrossRef CAS PubMed.
- D. G. Haung, S. J. Liao, J. M. Liu, Z. Dang and L. Petrik, J. Photochem. Photobiol., A, 2006, 184, 282–288 CrossRef PubMed.
- E. Allain, S. Besson, C. Durand, M. Moreau, T. Gacoin and J. P. Boilot, Adv. Funct. Mater., 2007, 17, 549–554 CrossRef CAS.
- W. Y. Gan, S. W. Lam, K. Chiang, R. Amal, H. Zhao and M. P. Brungs, J. Mater. Chem., 2007, 17, 952–954 RSC.
- X. Cheng, X. Yu and Z. Xing, Mater. Res. Bull., 2012, 47, 3804–3809 CrossRef CAS PubMed.
- X. Shi, H. Qin, X. Yang and Q. Zhang, Mater. Res. Bull., 2012, 47, 4347–4352 CrossRef CAS PubMed.
- Y. Yang, H. Y. Wang, X. Li and C. Wang, Mater. Lett., 2009, 63, 331–333 CrossRef CAS PubMed.
- D. N. Ke, H. J. Liu, T. Y. Peng, X. Liu and K. Dai, Mater. Lett., 2008, 62, 447–450 CrossRef CAS PubMed.
- A. Charanpahari, S. S. Umare, S. P. Gokhale, V. Sudarsan, B. Sreedhar and R. Sasikala, Appl. Catal., A, 2012, 443–444, 96–102 CrossRef CAS PubMed.
- J. Xu, Y. Ao, D. Fu and C. Yuan, J. Hazard. Mater., 2009, 164, 762–768 CrossRef CAS PubMed.
- Y. Xie and C. Yuan, Appl. Catal., B, 2003, 46, 251–259 CrossRef CAS.
- F. Dong, W. Zhao and Z. Wu, Nanotechnology, 2008, 19, 365607–365617 CrossRef PubMed.
- W. Wang, C. Lu, Y. Ni, M. Su, W. Huang and Z. Xu, Appl. Surf. Sci., 2012, 258, 8696–8703 CrossRef CAS PubMed.
- K. Obata, H. Irie and K. Hashimoto, Chem. Phys., 2007, 339, 124–132 CrossRef CAS PubMed.
- X. P. Cao, D. Li, W. H. Jing, W. H. Xing and Y. Q. Fan, J. Mater. Chem., 2012, 22, 15309–15315 RSC.
- H. Jiang, P. Yan, Q. Wang, S. Zang, J. Li and Q. Wang, Chem.–Eng. J., 2013, 215–216, 348–357 CrossRef CAS PubMed.
- S. H. Wang and S. Q. Zhou, J. Hazard. Mater., 2011, 185, 77–85 CrossRef CAS PubMed.
- Y. Shen, T. Xiong, H. Du, H. Jin, J. Shang and K. Yang, J. Sol–Gel Sci. Technol., 2009, 50, 98–102 CrossRef CAS PubMed.
-
(a) N. Serpone, E. Borgarello and M. J. Gratzel, J. Chem. Soc., Chem. Commun., 1984, 342–344 RSC;
(b) J. Yu, S. Wang, J. Low and W. Xiao, Phys. Chem. Chem. Phys., 2013, 15, 16883–16890 RSC;
(c) M. Zhou, J. Yu, S. Liu, P. Zhai and L. Jiang, J. Hazard. Mater., 2008, 157, 1141–1148 CrossRef PubMed;
(d) T. Giannakopoulou, N. Todorova, M. Giannoui, J. Yu and C. Trapalis, Catal. Today, 2014, 230, 174–180 CrossRef PubMed;
(e) G. Dai, J. Yu and G. Liu, J. Phys. Chem. C, 2011, 115, 7339–7346 CrossRef CAS;
(f) X. Wang, S. Li, Y. Ma, H. Yu and J. Yu, J. Phys. Chem. C, 2011, 115, 14648–14655 CrossRef CAS.
-
(a) N. Serpone, P. Maruthamuthu, P. Pichat, E. Pelizzetti and H. J. Hidaka, J. Photochem. Photobiol., A, 1995, 85, 247–255 CrossRef CAS;
(b) S. G. Kumar and K. S. R. Koteswara Rao, Energy Environ. Sci., 2014, 7, 45–102 RSC.
- K. Vinodgopal and P. V. Kamat, Environ. Sci. Technol., 1995, 29, 841–845 CrossRef CAS PubMed.
- Y. Cao, X. Zhang, W. Yang, H. Du, Y. Bai, T. Li and J. Yao, J. Chem. Mater., 2000, 12, 3445–3448 CrossRef CAS.
- W. Ho, J. C. Yu, J. Lin, J. Yu and P. Li, Langmuir, 2004, 20, 5865–5869 CrossRef CAS.
- H. Park, W. Choi and M. R. Hoffmann, J. Mater. Chem., 2008, 18, 2379–2385 RSC.
- F. Peng, L. Cai, L. Huang, H. Yu and H. Wang, J. Phys. Chem. Solids, 2008, 69, 1657–1664 CrossRef CAS PubMed.
- M. Sathish, B. Viswanathan, R. P. Viswanath and C. S. Gopinath, Chem. Mater., 2005, 17, 6349–6353 CrossRef CAS.
- L. Gai, X. Duan, H. Jiang, Q. Mei, G. Zhou, Y. Tian and H. Li, CrystEngComm, 2012, 14, 7662–7671 RSC.
- N. Wu, J. Wang, D. Nyago Tafen, H. Wang, J. Zheng, J. P. Lewis, X. Liu, S. S. Leonard and A. Manivannan, J. Am. Chem. Soc., 2010, 132, 6679–6685 CrossRef CAS PubMed.
- T. A. Kandiel, A. Feldhoff, L. Robben, R. Dillert and D. W. Bahnemann, Chem. Mater., 2010, 22, 2050–2060 CrossRef CAS.
- T. A. Kandiel, L. Robben, A. Alkaim and D. Bahnemann, Photochem. Photobiol. Sci., 2013, 12, 602–609 CAS.
- J. C. Yu, L. Zhang and J. Yu, Chem. Mater., 2002, 14, 4647–4653 CrossRef CAS.
- G. Tian, H. Fu, L. Jing, B. Xin and K. Pan, J. Phys. Chem. C, 2008, 112, 3083–3089 CAS.
- H. U. Lee, S. C. Lee, J. H. Seo, W. G. Hong, H. Kim, H. J. Yun, H. J. Kim and J. Lee, Chem.–Eng. J., 2013, 223, 209–215 CrossRef CAS PubMed.
- F. Li, Y. Zhao, Y. Hao, X. Wang, R. Liu, D. Zhao and D. Chen, J. Hazard. Mater., 2012, 239–240, 118–127 CrossRef CAS PubMed.
- M. Ishimaru, Y. Hirotsu, I. V. Afanasyev-Charkin and K. E. Sickafus, J. Phys.: Condens. Matter, 2002, 14, 1237–1247 CrossRef CAS.
- X. Wang, J. C. Yu, Y. Chen, L. Wu and X. Fu, Environ. Sci. Technol., 2006, 40, 2369–2374 CrossRef CAS.
- A. Hagfeldt and M. Gratzel, Acc. Chem. Res., 2000, 33, 269–277 CrossRef CAS PubMed.
- F. Dong, Y. Sun and M. Fu, Int. J. Photoenergy, 2012, 569716 Search PubMed.
- Z. Wu, F. Dong, Y. Liu and H. Wang, Catal. Commun., 2009, 11, 82–86 CrossRef CAS PubMed.
- J. Yu and J. Ran, Energy Environ. Sci., 2011, 4, 1364–1371 CAS.
- H. Jiang, M. Nagai and K. Kobayashi, J. Alloys Compd., 2009, 479, 821–827 CrossRef CAS PubMed.
- X. Yong and M. A. A. Schoonen, Am. Mineral., 2000, 85, 543–556 Search PubMed.
- M. Hojamberdiev, R. Prasad, K. Morita, Y. Zhu, M. A. Schiavon, A. Gurlo and R. Riedel, Appl. Catal., B, 2012, 115–116, 303–313 CrossRef CAS PubMed.
- T. Xu, L. Zhang, H. Cheng and Y. Zhu, Appl. Catal., B, 2011, 101, 382–387 CrossRef CAS PubMed.
- L. Zhang, X. Li, Z. Chang and D. Li, Mater. Sci. Semicond. Process., 2011, 14, 52–57 CrossRef CAS PubMed.
- Y. Haung, Y. Wei, J. Wu, C. Guo, M. Wang, S. Yin and T. Sato, Appl. Catal., B, 2012, 123–124, 9–17 CrossRef PubMed.
- S. Bagwasi, Y. Niu, M. Nasir, B. Tian and J. Zhang, Appl. Surf. Sci., 2013, 264, 139–147 CrossRef CAS PubMed.
- A. Testino, I. R. Bellobono, V. Buscaglia, C. Canevali, M. D'Arienzo, S. Polizzi, R. Scotti and F. Morazzoni, J. Am. Chem. Soc., 2007, 129, 3564–3575 CrossRef CAS PubMed.
- L. Kong, H. Chen, W. Hua, S. Zhang and J. Chen, Chem. Commun., 2008, 4977–4979 RSC.
- H. Cheng, B. Huang, Y. Dai, X. Qin, X. Zhang, Z. Wang and M. Jiang, J. Solid State Chem., 2009, 182, 2274–2278 CrossRef CAS PubMed.
- P. Yap, Y. Cheah, M. Srinivasan and T. Lim, Appl. Catal., A, 2012, 427–428, 125–136 CrossRef CAS PubMed.
- K. M. Schindler and M. Kunst, J. Phys. Chem., 1990, 94, 8222–8226 CrossRef CAS.
- R. I. Bickley, T. G. Carreno, J. S. Lees, L. Palmisano and R. J. D. Tilley, J. Solid State Chem., 1991, 92, 178–190 CrossRef CAS.
- D. C. Hurum, A. G. Agrios, K. A. Gray, T. Rajh and M. C. Thurnauer, J. Phys. Chem. B, 2003, 107, 4545–4549 CrossRef CAS.
- R. Beranek, B. Neumann, S. Sakthivel, M. Janczarek, T. Dittrich, H. Tributsch and H. Kisch, Chem. Phys., 2007, 339, 11–19 CrossRef CAS PubMed.
- C. D. Valentin, E. Finazzi, G. Pacchioni, A. Selloni, S. Livraghi, M. C. Paganini and E. Giamello, Chem. Phys., 2007, 339, 44–56 CrossRef PubMed.
- P. Yap, T. Lima and M. Srinivasan, Catal. Today, 2011, 161, 46–52 CrossRef CAS PubMed.
- Y. Li, S. Zhang, Q. Yu and W. Yin, Appl. Surf. Sci., 2007, 253, 9254–9258 CrossRef CAS PubMed.
- X. M. Yan, J. Kang, L. Gao, L. Xiong and P. Mei, Appl. Surf. Sci., 2013, 265, 778–783 CrossRef CAS PubMed.
- D. Mitoraj and H. Kisch, Angew. Chem., Int. Ed., 2008, 47, 9975–9978 CrossRef CAS PubMed.
- N. R. Khalid, E. Ahmed, Z. Hong, Y. Zhang and M. Ahmad, Curr. Appl. Phys., 2012, 12, 1485–1492 CrossRef PubMed.
- N. L. Yang, J. Zhai, D. Wang, Y. S. Chen and L. Jiang, ACS Nano, 2010, 4, 887–894 CrossRef CAS PubMed.
- B. Neppolian, A. Bruno, C. L. Bianchi and M. A. Kumar, Ultrason. Sonochem., 2012, 19, 9–15 CrossRef CAS PubMed.
- X. Shang, M. Zhang, X. Wang and Y. Yang, J. Exp. Nanosci., 2012, 1–13 Search PubMed.
- Y. P. Zhang and C. X. Pan, J. Mater. Sci., 2011, 46, 2622–2626 CrossRef CAS.
- N. Yang, G. Li, W. Wang, X. Yang and W. F. Zhang, J. Phys. Chem. Solids, 2011, 72, 1319–1324 CrossRef CAS PubMed.
- Y. Cheng, V. Subramaniam, D. Gong, Y. Tang, J. Highfield, S. Pehkonen, P. Pichat, M. Schreyer and Z. Chen, J. Solid State Chem., 2012, 196, 518–527 CrossRef CAS PubMed.
- P. Zhang, X. Liu, S. Yin and T. Sato, Appl. Catal., B, 2010, 93, 299–303 CrossRef CAS PubMed.
- H. Li, S. Yin and T. Sato, Appl. Catal., B, 2011, 106, 586–591 CrossRef CAS PubMed.
- S. H. Choi, N. H. Kim, Y. H. Yun and S. C. Choi, J. Ceram. Process, Res., 2006, 7, 62–65 Search PubMed.
-
(a) M. V. Dozzi, L. Prati, P. Canton and E. Selli, Phys. Chem. Chem. Phys., 2009, 11, 7171–7180 RSC;
(b) Y. Wang, J. Yu, W. Xiao and Q. Li, J. Mater. Chem. A, 2014, 2, 3847–3855 RSC;
(c) J. Yu, W. Wang, B. Cheng and B. L. Su, J. Phys. Chem. C, 2009, 113, 6743–6750 CrossRef CAS.
- M. I. Litter, Appl. Catal., B, 1999, 23, 89–114 CrossRef CAS.
- A. Primo, A. Corma and H. Garcia, Phys. Chem. Chem. Phys., 2011, 13, 886–910 RSC.
-
(a) P. Kamat, J. Phys. Chem. B, 2002, 106, 7729–7744 CrossRef CAS;
(b) Z. Xu, J. Yu and G. Liu, Electrochem. Commun., 2011, 13, 1260–1263 CrossRef CAS PubMed;
(c) J. Yu, L. Yue, S. Liu, B. Huang and X. Zhang, J. Colloid Interface Sci., 2009, 334, 58–64 CrossRef CAS PubMed;
(d) J. Yu, L. Qi and M. Jaroniec, J. Phys. Chem. C, 2010, 114, 13118–13125 CrossRef CAS.
- V. Brezova, A. Blazkova, L. Karpinsky, J. Groskova, B. Havlinova, V. Jorik and M. Ceppan, J. Photochem. Photobiol., A, 1997, 109, 177–183 CrossRef CAS.
- J. Lee and W. Choi, Environ. Sci. Technol., 2004, 38, 4026–4033 CrossRef CAS.
- P. D. Cozzoli, M. L. Curri and A. Agostiano, Chem. Commun., 2005, 3186–3188 RSC.
- J. Lu, F. Su, Z. Haung, C. Zhang, Y. Liu, X. Ma and J. Gong, RSC Adv., 2013, 3, 720–724 RSC.
- E. W. McFarland and J. Tang, Nature, 2003, 421, 616–618 CrossRef CAS PubMed.
- W. Jiang, N. Ullah, G. Divitini, C. Ducati, R. V. Kumar, Y. Ding and Z. H. Barber, Langmuir, 2012, 28, 5427–5431 CrossRef CAS PubMed.
- Y. Gao, P. Fang, F. Chen, Y. Liu, Z. Liu, D. Wang and Y. Dai, Appl. Surf. Sci., 2013, 265, 796–801 CrossRef CAS PubMed.
- M. Wu, B. Yang, Y. Lv, Z. Fu, J. Xu, T. Guo and Y. Zhoa, Appl. Surf. Sci., 2010, 256, 7125–7130 CrossRef CAS PubMed.
- J. X. Li, J. H. Xu, W. L. Dai and K. N. Fan, J. Phys. Chem. C, 2009, 113, 8343–8349 CAS.
- E. Stathatos, P. Lianos, P. Falaras and A. Siokou, Langmuir, 2000, 16, 2398–2400 CrossRef CAS.
- Y. H. Ao, J. J. Xu, D. G. Fu and C. W. Yuan, J. Phys. Chem. Solids, 2008, 69, 2660–2664 CrossRef CAS PubMed.
- B. Z. Tian, C. Z. Li, F. Gu and H. B. Jiang, Catal. Commun., 2009, 10, 925–929 CrossRef CAS PubMed.
- J. Virkutyte and R. S. Varma, RSC Adv., 2012, 2, 1533–1539 RSC.
- A. Kumar, A. K. Pandey, S. S. Singh, R. Shanker and A. Dhawan, Free Radical Biol. Med., 2011, 51, 1872–1881 CrossRef CAS PubMed.
-
(a) T. Morikawa, T. Ohwaki, K. Suzuki, S. Moribe and S. Kubota, Appl. Catal., B, 2008, 83, 56–62 CrossRef CAS PubMed;
(b) L. G. Devi, S. G. Kumar, K. M. Reddy and C. Munikrishnappa, J. Hazard. Mater., 2009, 164, 459–467 CrossRef PubMed;
(c) L. G. Devi, K. E. Rajashekhar, K. S. A. Raju and S. G. Kumar, J. Mol. Catal. A: Chem., 2009, 314, 88–94 CrossRef CAS PubMed;
(d) L. G. Devi, K. E. Rajashekhar, K. S. A. Raju and S. G. Kumar, Desalination, 2011, 270, 31–39 CrossRef PubMed;
(e) L. G. Devi, K. S. A. Raju and S. G. Kumar, J. Environ. Monit., 2009, 11, 1397–1404 RSC;
(f) L. G. Devi, S. G. Kumar and K. M. Reddy, Cent. Eur. J. Chem., 2009, 7, 468–477 CrossRef CAS PubMed.
- S. Yin, B. Liu, P. Zhang, T. Morikawa, K. Yamanaka and T. Sato, J. Phys. Chem. C, 2008, 112, 12425–12431 CAS.
- J. R. Kanofsky, Chem.-Biol. Interact., 1989, 70, 1–28 CrossRef CAS.
- M. V. Dozzi, A. Saccomanni, M. Altomare and E. Selli, Photochem. Photobiol. Sci., 2013, 12, 595–601 CAS.
- P. V. Kamat, J. Phys. Chem. Lett., 2012, 3, 663–672 CrossRef CAS.
- A. T. Kuvarega, R. W. M. Krause and B. B. Mamba, J. Phys. Chem. C, 2011, 115, 22110–22120 CAS.
- X. Li, Z. Zhuang, W. Li and H. Pan, Appl. Catal., A, 2012, 429–430, 31–38 CrossRef CAS PubMed.
- N. Strataki, N. Boukos, F. Paloukis, S. G. Neophytides and P. Lianos, Photochem. Photobiol. Sci., 2009, 8, 639–643 CAS.
- A. H. Yahaya, M. A. Gondal and A. Hameed, Chem. Phys. Lett., 2004, 400, 206–212 CrossRef CAS PubMed.
- K. Koci, L. Obalova, L. Matejova, D. Placha, Z. Lacny, J. Jirkovsky and O. Solcova, Appl. Catal., B, 2009, 89, 494–502 CrossRef CAS PubMed.
- S. S. Tan, L. Zou and E. Hu, Catal. Today, 2008, 131, 125–129 CrossRef CAS PubMed.
- M. C. Wu, J. Hiltunen, A. Sapi, A. Avila, W. Larsson, H. C. Lia, M. Huuhtanen, G. Toth, A. Shchukarev, N. Laufer, A. Kukovecz, Z. Konya, J. P. Mikkola, R. Keiski, W. F. Su, Y. F. Chen, H. Jantunen, P. M. Ajayan, R. Vajtai and K. Korda, ACS Nano, 2011, 5, 5025–5030 CrossRef CAS PubMed.
- A. Pandikumar, K. Sivaranjani, C. S. Gopinath and R. Ramaraj, RSC Adv., 2013, 3, 13390–13398 RSC.
- A. Sanchez, S. Abbet, U. Heiz, W. D. Schneider, H. Hakkinen, R. N. Barnett and U. Landman, J. Phys. Chem. A, 1999, 103, 9573–9578 CrossRef CAS.
- S. Laursen and S. Linic, Phys. Rev. Lett., 2006, 97, 026101 CrossRef.
- S. Laursen and S. Linic, Phys. Chem. Chem. Phys., 2009, 11, 11006–11012 RSC.
- C. T. Campbell, Science, 2004, 306, 234–235 CrossRef CAS PubMed.
- M. S. Chen and D. W. Goodman, Science, 2004, 306, 252–255 CrossRef CAS PubMed.
- R. G. Pearson, J. Am. Chem. Soc., 1963, 85, 3533–3539 CrossRef CAS.
- V. Subramanian, E. E. Wolf and P. V. Kamat, J. Phys. Chem. B, 2001, 105, 11439–11446 CrossRef CAS.
- K. Yu, Y. Tian and T. Tatsuma, Phys. Chem. Chem. Phys., 2006, 8, 5417–5420 RSC.
- C. S. Kim, J. W. Shin, Y. H. Cho, H. D. Jang, H. S. Byun and T. O. Kim, Appl. Catal., A, 2013, 455, 211–218 CrossRef CAS PubMed.
- W. N. Wang, J. H. Park and P. Biswas, Catal. Sci. Technol., 2011, 1, 593–600 CAS.
- T. Morikawa, Y. Irokawa and T. Ohwaki, Appl. Catal., A, 2006, 314, 123–127 CrossRef CAS PubMed.
- M. B. Fishera, D. A. Keaneb, P. F. Ibanez, J. Colreavy, S. J. Hinderd, K. G. McGuigana and S. C. Pillai, Appl. Catal., B, 2013, 130–131, 8–13 CrossRef PubMed.
- Y. Kikuchi, K. Sunada, T. Iyoda, K. Hashimoto and A. Fujishima, J. Photochem. Photobiol., A, 1997, 106, 51–56 CrossRef CAS.
- Y. Horie, M. Taya and S. Tone, J. Chem. Eng. Jpn., 1998, 31, 922–929 CrossRef CAS.
- J. A. R. Herrera and C. Pulgarin, Sol. Energy, 2010, 84, 37–43 CrossRef PubMed.
- S. Ikeda, N. Sugiyama, B. Pal, G. Marci, L. Palmisano, H. Noguchi, K. Uosaki and B. Ohtani, Phys. Chem. Chem. Phys., 2001, 3, 267–273 RSC.
-
(a) J. Yuan, M. Chen, J. Shi and W. Shangguan, Int. J. Hydrogen Energy, 2006, 31, 1326–1331 CrossRef CAS PubMed;
(b) Q. Xiang and J. Yu, J. Phys. Chem. Lett., 2013, 4, 753–759 CrossRef CAS;
(c) Q. Xiang, J. Yu and M. Jaroniec, J. Am. Chem. Soc., 2012, 134, 6575–6578 CrossRef CAS PubMed.
-
(a) M. M. Joshi, P. A. Mangrulkar, S. N. Tijare, P. S. Padole, D. V. Parwate, N. K. Labhsetwar and S. S. Rayalu, Int. J. Hydrogen Energy, 2012, 37, 10457–10461 CrossRef CAS PubMed;
(b) Q. Li, H. Meng, P. Zhou, Y. Zheng, J. Wang, J. Yu and J. Gong, ACS Catal., 2013, 3, 882–889 CrossRef CAS;
(c) Q. Xiang, J. Yu and M. Jaroniec, J. Phys. Chem. C, 2011, 115, 7355–7363 CrossRef CAS.
- K. E. Karakitsou and X. E. Verykios, J. Phys. Chem., 1993, 97, 1184–1189 CrossRef CAS.
- Y. Li, G. Ma, S. Peng, G. Lu and S. Li, Appl. Surf. Sci., 2008, 254, 6831–6836 CrossRef CAS PubMed.
- F. Zhuge, L. Jin and G. Lu, J. Mol. Catal., 2007, 21, 233–238 CAS.
- T. Ihara, M. Miyoshi, Y. Iriyama, O. Matsumoto and S. Sugihara, Appl. Catal., B, 2003, 42, 403–409 CrossRef CAS.
- Y. V. Pleskov, Sov. Electrochem., 1981, 17, 1–25 Search PubMed.
- X. Sun, H. Liu, J. Dong, J. Wei and Y. Zhang, Catal. Lett., 2010, 135, 219–225 CrossRef CAS PubMed.
- R. Sasikala, A. R. Shirole, V. Sudarsan, Jagannath, C. Sudakar, R. Naik, R. Rao and S. R. Bharadwaj, Appl. Catal., A, 2010, 377, 47–54 CrossRef CAS PubMed.
- X. Yang, Y. Wang, L. Xu, X. Yu and Y. Guo, J. Phys. Chem. C, 2008, 112, 11481–11489 CAS.
- R. Sasikala, V. Sudarshan, C. Sudakar, R. Naik, L. Panicker and S. R. Bharadwaj, Int. J. Hydrogen Energy, 2009, 34, 6105–6113 CrossRef CAS PubMed.
- W. Sun, S. Zhang, Z. Liu, C. Wang and Z. Mao, Int. J. Hydrogen Energy, 2008, 33, 1112–1117 CrossRef CAS PubMed.
- P. V. Kamat, J. Phys. Chem. C, 2007, 111, 2834–2860 CAS.
- O. D. Jayakumar, R. Sasikala1, C. A. Betty, A. K. Tyagi, S. R. Bharadwaj, U. K. Gautam, P. Srinivasu and A. Vinu, J. Nanosci. Nanotechnol., 2009, 9, 4663–4667 CrossRef CAS PubMed.
- K. Domen, S. Naito, T. Onishi, K. Tamaru and M. Soma, J. Phys. Chem., 1982, 86, 3657–3661 CrossRef CAS.
- F. N. Sayed, O. D. Jayakumar, R. Sasikala, R. M. Kadam, S. R. Bharadwaj, L. Kienle, U. Schürmann, S. Kaps, R. Adelung, J. P. Mittal and A. K. Tyagi, J. Phys. Chem. C, 2012, 116, 12462–12467 CAS.
- S. Martha, D. P. Das, N. Biswal and K. M. Parida, J. Mater. Chem., 2012, 22, 10695–10703 RSC.
- H. Lv, L. Ma, P. Zeng, D. Ke and T. Peng, J. Mater. Chem., 2010, 20, 3665–3672 RSC.
- K. M. Parida, M. Satpathy and L. Mohapatra, J. Mater. Chem., 2012, 22, 7350–7357 RSC.
- J. Lv, T. Kako, Z. Li, Z. Zou and J. Ye, J. Phys. Chem. C, 2010, 114, 6157–6162 CAS.
- Y. Wang, Y. R. Su, L. Qiao, L. X. Liu, Q. Su, C. Q. Zhu and X. Q. Liu, Nanotechnology, 2011, 22, 225702 CrossRef CAS PubMed.
- M. Gratzel, Nature, 2001, 414, 338–344 CrossRef CAS PubMed.
- R. Souda, W. Hayami, T. Aizawa and Y. Ishizawa, Surf. Sci., 1993, 285, 265–274 CrossRef CAS.
- A. Suli, M. I. Torok and I. Hevesi, Thin Solid Films, 1986, 139, 233–246 CrossRef CAS.
- F. Pei, Y. Liu, S. Xu, J. Lu, C. Wang and S. Cao, Int. J. Hydrogen Energy, 2013, 38, 2670–2677 CrossRef CAS PubMed.
- X. Wang, L. J. Zhi and K. Mullen, Nano Lett., 2008, 8, 323–327 CrossRef CAS PubMed.
- X. Q. Gong and A. Selloni, J. Phys. Chem. B, 2005, 109, 19560–19562 CrossRef CAS PubMed.
-
(a) M. Lazzeri, A. Vittadini and A. Selloni, Phys. Rev. B: Condens. Matter Mater. Phys., 2002, 65, 119901 CrossRef;
(b) L. Qi, J. Yu and M. Jaroniec, Phys. Chem. Chem. Phys., 2011, 13, 8915–8923 RSC;
(c) Q. Xiang, J. Yu and M. Jaroniec, Chem. Commun., 2011, 47, 4532–4534 RSC;
(d) J. Yu, J. Fan and K. Lv, Nanoscale, 2010, 2, 2144–2149 RSC;
(e) J. Yu, G. Dai, Q. Xiang and M. Jaroniec, J. Mater. Chem., 2011, 21, 1049–1057 RSC;
(f) K. Lv, Q. Xiang and J. Yu, Appl. Catal., B, 2011, 104, 275–281 CrossRef CAS PubMed.
-
(a) X. Zhou, F. Peng, H. Wang, H. Yu and Y. Fang, Chem. Commun., 2012, 48, 600–602 RSC;
(b) Q. Xiang, J. Yu, W. Wang and M. Jaroniec, Chem. Commun., 2011, 47, 6906–6908 RSC.
- T. Hirakawa and Y. Nosaka, Langmuir, 2002, 18, 3247–3254 CrossRef CAS.
- W. Wang, C. Lu, Y. Ni, M. Su and Z. Xu, Appl. Catal., B, 2012, 127, 28–35 CrossRef CAS PubMed.
- Y. Zhang, C. Li and C. Pan, J. Am. Ceram. Soc., 2012, 95, 2951–2956 CrossRef CAS PubMed.
- J. H. Pan, G. Han, R. Zhou and X. S. Zhao, Chem. Commun., 2011, 47, 6942–6944 RSC.
- J. H. Pan, X. Zhang, A. J. Du, D. D. Sun and J. O. Leckie, J. Am. Chem. Soc., 2008, 130, 11256–11257 CrossRef CAS PubMed.
- J. H. Pan, H. Dou, Z. Xiong, C. Xu, J. Ma and X. S. Zhao, J. Mater. Chem., 2010, 20, 4512–4528 RSC.
- Z. Bian, J. Zhu, F. Cao, Y. Lu and H. Li, Chem. Commun., 2009, 3789–3791 RSC.
- G. Dai, S. Liu, Y. Liang, H. Liu and Z. Zhong, J. Mol. Catal. A: Chem., 2013, 368–369, 38–42 CrossRef CAS PubMed.
- H. M. Zhang, P. Liu, F. Li, H. W. Liu, Y. Wang, S. Q. Zhang, M. X. Guo, H. M. Cheng and H. J. Zhao, Chem.–Eur. J., 2011, 17, 5949–5957 CrossRef CAS PubMed.
- Q. J. Xiang and J. G. Yu, Chin. J. Catal., 2011, 32, 525–531 CrossRef CAS.
- M. Y. Xing, D. Y. Qi, J. L. Zhang and F. Chen, Chem.–Eur. J., 2011, 17, 11432–11436 CrossRef CAS PubMed.
- X. Zong, Z. Xing, H. Yu, Z. Chen, F. Tang, J. Zou, G. Q. Lu and L. Wang, Chem. Commun., 2011, 47, 11742–11744 RSC.
- K. Maeda, B. Lee, D. Lu and K. Domen, Chem. Mater., 2009, 21, 2286–2291 CrossRef CAS.
- Q. Xiang, J. Yu and M. Jaroniec, Phys. Chem. Chem. Phys., 2011, 13, 4853–4861 RSC.
- W. Shi, W. Yang, Q. Li, S. Gao, P. Shang and J. K. Shang, Nanoscale Res. Lett., 2012, 7, 590 CrossRef PubMed.
- J. M. Wu and M. L. Tang, Nanoscale, 2011, 3, 3915–3922 RSC.
- M. A. Fox and M. T. Dulay, Chem. Rev., 1993, 93, 341–357 CrossRef CAS.
- N. Serpone, J. Phys. Chem. B, 2006, 110, 24287–24293 CrossRef CAS PubMed.
- K. Selvam, S. Balachandran, R. Velmurugan and M. Swaminathan, Appl. Catal., A, 2012, 413–414, 213–222 CrossRef CAS PubMed.
- K. Shibata, T. Mimura, M. Matsui, T. Sugiura and H. Minoura, J. Chem. Soc., Chem. Commun., 1988, 1318–1320 RSC.
- Y. Hirose, T. Mori, Y. Morishita, A. Itadani, T. Kudoh, T. Ohkubo, T. Matsuda, S. Kittaka and Y. Kuroda, Inorg. Chem., 2011, 50, 9948–9957 CrossRef CAS PubMed.
- K. Selvam and M. Swaminathan, RSC Adv., 2012, 2, 2848–2855 RSC.
- K. Selvam and M. Swaminathan, Synth. React. Inorg. Met.-Org. Chem., 2013, 43, 500–508 CrossRef CAS.
- S. Yurdakal, V. Augugliaro, V. Loddo, G. Palmisano and L. Palmisano, New J. Chem., 2012, 36, 1762–1768 RSC.
- T. Ohno, K. Sarukawa, K. Tokieda and M. Matsumura, J. Catal., 2001, 203, 82–86 CrossRef CAS.
|
This journal is © The Royal Society of Chemistry 2014 |