DOI:
10.1039/C4RA02621G
(Paper)
RSC Adv., 2014,
4, 26787-26795
Phloroglucinol-encapsulated starch biopolymer: preparation, antioxidant and cytotoxic effects on HepG2 liver cancer cell lines
Received
25th March 2014
, Accepted 27th May 2014
First published on 29th May 2014
Abstract
Phloroglucinol-encapsulated starch biopolymer was prepared by graft polymerization in the presence of sodium hydroxide as catalyst. The biopolymer was characterized by Fourier Infrared spectroscopy (FT-IR), scanning electron microscopy (SEM), X-ray diffraction (XRD), dynamic light scattering and 1H nuclear magnetic resonance spectroscopy (1H NMR). The in vitro quantification of the biopolymer revealed that phloroglucinol was encapsulated by soluble starch at a ratio of 3
:
1. It is also observed that the antioxidant potential of the biopolymer were significantly high in terms of concentration. The cytotoxic assay showed that the biopolymer is effective in killing HepG2 liver cancer cells lines in a dose-dependent manner with morphological evidence. To conclude, the comprehensive applications of phloroglucinol-encapsulated starch biopolymer agrees with green chemistry principles to satisfy future needs in the field of medical and pharmaceutical industry.
1. Introduction
Natural phenolic compounds are valuable substances that have a wide range of biological and pharmaceutical applications.1–3 However, the lack of long-term stability makes these compounds sensitive to physical, chemical and biological stress.4 To overcome this, better formulation of phenolic compounds is required to maintain their structural integrity and bioavailability towards physiological targets. In the recent past, there has been huge interest in the development of biomolecules with pharmaceutically active ingredients for sustained release. To achieve this, quite a number of challenging criteria need to be fulfilled: (a) the formulation of patient-friendly drugs, (b) sustained release with prolonged pharmaceutical activity, and (c) the risk associated with the frequent administration of drugs. Keeping this in mind, researchers are in an urge to develop new delivery system substantially over the present methodologies. It is also important to note that, the material used in the formulation of drug should not harm the environment.5
Phloroglucinol (1,3,5-trihydroxybenzene) is a phenolic compound restricted to marine brown seaweeds (Phaeophyceae). In microbes, these compounds are biosynthesized via acetyl-malonate pathway in the perinuclear region.6 The monomeric units of phloroglucinol abide to form the backbone of over 700 secondary metabolites.7 Phloroglucinol and its derivatives are shown to have antiplasmodic, antibacterial, antiviral, antimalarial, antioxidant, antidepresstive and anticarcinogenic activities.8–17 However, reports on the encapsulation of phloroglucinol are rare. On the basis of the above background, an approach has been made towards better encapsulation of phloroglucinol using starch.
Starch is a natural, renewable, biopolymer available from plant sources and is entirely biodegradable. In recent years, the use of starch as a pharmaceutical excipient, tablet disintegrant, controlled/sustained release polymer for drugs and hormones, plasma volume engineering, artificial red cells and nanotechnology have increased dramatically.18 This can be achieved by several chemical modifications made on starch for the production of starch-based byproducts for the controlled release of materials.19 However, graft polymerization is a specialized route that demonstrates the combinatorial approach of binding natural and synthetic polymers. Keeping this in mind, we herein report the grafting process by altering the polar hydroxyl groups on the surface of starch catalyzed by sodium hydroxide onto phloroglucinol (biopolymer). In addition, in vitro antioxidant (DPPH, ABTS+ and reducing power) and cytotoxic activity against HepG2 human liver cancer cells were also evaluated.
2. Materials and methods
2.1 Chemicals
Phloroglucinol and 2,4-dimethoybenzaldehde (DMBA) used in this study were purchased from Sigma-Aldrich Chemie GmbH (St. Louis, USA). 2,2-Diphenyl-1-picrylhydrazyl (DPPH) and 2,2′-azino-bis(3-ethylbenzothioazoline-6-sulfonic acid) diammonium salt (ABTS+) were procured from Alfa Aesar (A Johnson Mathew Company). Soluble starch and sodium hydroxide (NaOH) were obtained from Fisher Scientific respectively. All other chemicals used this study were of analytical grade and used as received.
2.2 Graft copolymerization
Graft copolymerization of phloroglucinol onto soluble starch was carried out in a round bottom flask containing soluble starch (5 g) and 30 mL of 0.4 M of sodium hydroxide (NaOH) solution. The mixture was heated at 80 °C to complete the gelatinization of the soluble starch and cooled rapidly to room temperature. To the above, phloroglucinol (1 g) and distilled water (30 mL) was added under constant stirring (1000 rpm) for 12 h at 60 °C alongside the dropwise addition of acetone (50 mL). The obtained final product was extracted with acetone (thrice) to remove unreacted phloroglucinol followed by drying at room temperature and ground to powder. Finally, the end product was dried in a hot air oven at 80 °C for 12 h for the removal of residual solvent and stored at room temperature.
2.3 In vitro quantification and loading efficiency
The in vitro quantification of starch and phloroglucinol present was determined by the anthrone and DMBA method respectively.20,21 The loading efficiency of phloroglucinol incorporated in the biopolymer was determined by centrifugation at 10
000 rpm for 30 min in Milli-Q water. The resultant supernatant was extracted with acetone for the separation of phloroglucinol. The acetone layer was evaporated under reduced pressure and dissolved in double distilled water before being assayed using a UV-visible spectrophotometer (Elico, SL-210) at 267 nm. The loading efficiency was calculated using the formula:
Loading efficiency = (total amount of phloroglucinol − free phloroglucinol)/total amount of phloroglucinol × 100. |
2.4 Characterization
The morphology and size of the grafted material was studied using a JOEL neoscope JSM-5000 scanning electron microscope (SEM) operating at an accelerating voltage of 10 kV. A Perkin-Elmer FT-IR spectrum-RX-1 spectrophotometer was used to record the functional groups at a resolution of 2 cm−1 ranging from 4000 to 400 cm−1 with KBr pellets in diffuse reflectance mode. In addition, XRD measurements were carried out using a Philips 86 X’Pert Pro X-ray diffractometer with a thin film glass substrate. Dynamic light scattering analysis such as particle size distribution and dynamic light scattering analysis were performed to study the average size distribution and stability of the biopolymer using Zetasizer Nano ZS90 (Malvern, UK). 1H-NMR spectra was recorded in dimethyl sulfoxide (DMSO) using a Bruker AV-400 instrument (400 MHz).
2.5 Release kinetics of phloroglucinol from biopolymer
The release kinetics of phloroglucinol from the biopolymer were determined using a dialysis bag membrane (MW cut off = 12–14 kDa, HiMedia) at physiological pH (pH 7.4). For the release to occur, 100 μg mL−1 of biopolymer was placed in the dialysis bag and dialyzed against 20 mL of phosphate buffer solution (pH 7.4). The setup was constantly stirred without disturbing the dialysis bag at 100 rpm for 24 h. Meanwhile, 2 mL of phosphate buffer solution was collected (the collected amount was replaced with freshly prepared phosphate buffer at the same pH) at predetermined intervals for the quantification of phloroglucinol using a UV-visible spectrophotometer (Elico, SL-210) at 267 nm.
2.6 In vitro antioxidant activity
2.6.1 DPPH assay. DPPH radical scavenging activity of the biopolymer was measured as described with minor modification.22 A 65 μM solution of DPPH (1.5 mL) was prepared in methanol and blended with 1.5 mL of biopolymer at different concentrations ranging from 0 to 200 μg mL−1 prepared in sterile distilled water. The reaction mixture was left undisturbed in dark at room temperature for 30 min. The optical density of the mixture was measured by a UV-visible spectrophotometer at 517 nm. The radical scavenging ability of the biopolymer was calculated using the following equation:
Percentage of DPPH radical scavenging activity = [A0 − A1]/A0 × 100. |
where, A0 is the absorbance of the control (DPPH in methanol + water), A1 is the absorbance of sample (biopolymer in water + DPPH in methanol). A standard calibration curve was plotted with phloroglucinol.
2.6.2 ABTS. The ABTS radical scavenging is determined by the methodology adopted by Re et al.23 Briefly, stock solutions (7 mM ABTS and 2.4 mM potassium persulphate) in equal volume were allowed to react for 12 h at room temperature in the dark for the preparation of a working solution. The absorbance (0.70 ± 0.02) of the working solution was measured spectrophotometrically at 734 nm with proper dilution with distilled water. Further, a freshly prepared solution of ABTS (1.5 mL) was mixed with 1.5 mL of biopolymer, and the mixture was vortexed followed by measuring the absorbance at 734 nm after 5 min incubation at room temperature. Phloroglucinol was used as a standard, and the percentage of ABTS+ inhibition by the biopolymer was calculated from the equation shown in Section 2.6.1.
2.6.3 Reducing power. According to Oyaizu,24 various concentrations (0–200 μg mL−1) of standard/test samples were made up to 0.5 mL using 20 mM of phosphate buffer (pH 6.6). To this, 0.5 mL of potassium hexacyanoferrate (0.1%) was added and incubated at 50 °C for 20 min in a water bath. Subsequent to the incubation, 0.5 mL of 10% TCA was added to terminate the reaction. The upper portion of the solution (approximately 1 mL) was collected by centrifugation at 5000 rpm for 20 min. To this, 1 mL of distilled water and 0.1 mL of 0.01% of ferric chloride solution was added. The reaction mixture was kept undisturbed for 15 min at ambient temperature, and the absorbance was recorded at 700 nm against the blank. Phloroglucinol was used as a standard for setting up the calibration curve.
2.7 Cytotoxicity effect on human liver cancer HepG2 cell lines
Human liver cancer HepG-2 cell lines were obtained from the National Centre for Cell Science, Pune, India. It was cultured in Dulbecco's modified Eagle's medium (DMEM: HiMedia Laboratories Mumbai, India), supplemented with 10% fetal bovine serum and 1% penicillin/streptomycin (HiMedia Laboratories Mumbai, India). Prior to the experiment, the compound was dissolved in 10% dimethyl sulfoxide (DMSO) to give a final concentration of not more than 0.5% that affect cell survival. Cytotoxicity assay was performed using an MTT (dimethyl thiazolyltetrazolium bromide) assay.25 Briefly, at a concentration of 1 × 105, HepG-2 cells were placed in 96 well plates. After 24 h, cells were washed twice with 100 μL of serum-free medium and starved for an hour at 37 °C. After starvation, the cells were treated with different concentrations of test compound (0–250 μg mL−1) for 24 h at 37 °C in a humidified atmosphere. At the end of the experiment, the cells-containing medium was aspirated followed by the addition of MTT (0.5 mg mL−1). Further, the cells were incubated under these experimental conditions for 4 h. The medium containing MTT was then discarded and the cells were washed with PBS (200 μL). The formazon crystals were then mixed with 100 μL of DMSO followed by reading their absorbance at 570 nm using a microtiter plate reader (BioRad). All the tests were performed in triplicate (n = 3) and statistical analysis was carried out using SPSS software Version 16 (SPSS Inc., Chicago, IL, USA). In addition, a morphological assessment of the cells treated (IC50 value) with the biopolymer was also studied by phase contrast and fluorescence microscopic (Hoechst 33258) staining.26
3. Results and discussion
3.1 Synthesis of the biopolymer by graft polymerization
The introduction of natural phenolic groups (phloroglucinol) on to the starch backbone was accomplished by graft polymerization.27 The addition of NaOH solution to starch as an initiator, tends to form sodium alkoxide ion-pair sites for the polymerization reaction.28 Meanwhile, the addition of phloroglucinol to the gelatinized starch initiates the process of grafting during incubation (12 h at 60 °C) with constant stirring along with the dropwise addition of acetone. The ion-pair sites on starch continued to react with the hydroxyl end of phloroglucinol, resulting in the formation of non-covalent hydrogen bonding with the sodium alkoxide ion. At the same time, the loss of water molecule along with acetone in the reaction mixture initiates retrogradation followed by re-crystallization of starch in the form of a biopolymer.29 The possible mechanism involved in the synthesis of phloroglucinol-encapsulated starch biopolymer is presented in Scheme. 1. The biopolymer showed good dispersity in water when compared to solvents like methanol, acetone and ethanol. Apart from this, the in vitro quantification of starch (30.30 ± 0.90 μg mL−1) and phloroglucinol (10.90 ± 0.66 μg mL−1) was found to be in the ratio of 3
:
1. It is also interesting to note that the loading efficiency of phloroglucinol onto the soluble starch was found to be 97%.
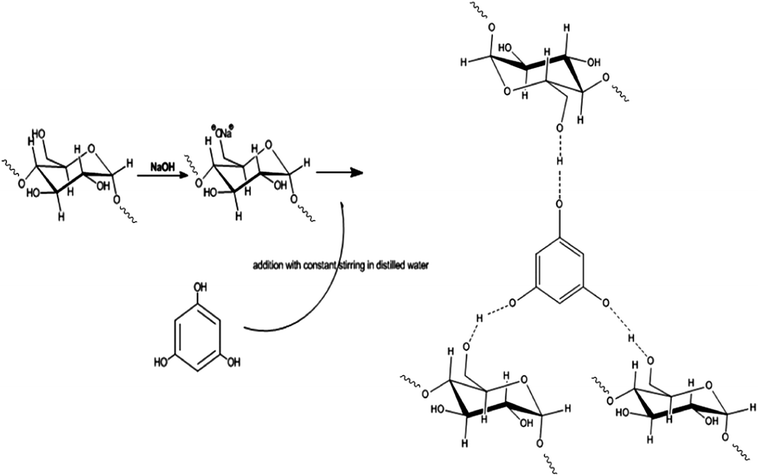 |
| Scheme 1 Possible mechanism involved in the formation of the biopolymer. | |
3.2 Scanning electron microscopic studies
Scanning electron microscopy was performed to study the morphology of soluble starch and the biopolymer. The soluble starch was found to be spherical in shape with sizes ranging between 1–100 μm as shown in Fig. 1a. However, the biopolymer was observed to be irregular in size, shape and texture with no agglomeration (Fig. 2b). The loss of water molecules during the process of grafting modifies the surface pattern of the biopolymer. In addition, there have been increases in the size of the biopolymer due to the compact polymeric surface coating when compared to the ungrafted product.30 We also assume that the structural integrity of phloroglucinol was not destroyed because of its high melting point (219 °C). The process of grafting enables disruption of the inter- and intra-molecular hydrogen bonding of soluble starch for better encapsulation of phloroglucinol possible at a minimum temperature of 60 °C.31,32
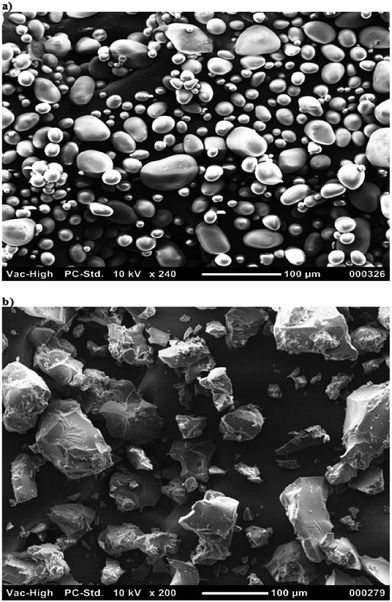 |
| Fig. 1 Scanning electron micrographs of (a) soluble starch and (b) biopolymer. | |
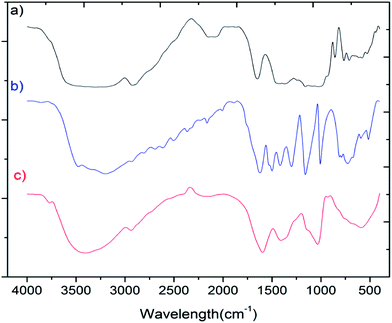 |
| Fig. 2 FT-IR spectra of (a) soluble starch, (b) phloroglucinol, and (c) biopolymer. | |
3.3 FT-IR spectroscopy
The FT-IR spectra of soluble starch, phloroglucinol and the biopolymer is shown in Fig. 2. For soluble starch, the band at 2923 cm−1 can be assigned to CH2 deformation, while the residual bands at 711, 579, 528 and 439 cm−1 infers the skeleton stretching vibrations of the pyranose rings.33–35 In the case of phloroglucinol, intense bands between 1500–1000 cm−1 indicate the presence of functional groups such as ν (C
C), γ (O–H) and ν (C–OH) bands.36 However, in the FT-IR spectra of the biopolymer, new stretching vibrations at 1596 and 1408 cm−1 can be assigned to aromatic C–H bending and CH2/CH3 deformations, confirming the encapsulation of phloroglucinol onto the starch matrix. In addition, the characteristic band were assigned for ν (O–H) stretching centered at 3296 and 3200 cm−1 for soluble starch and phloroglucinol, respectively. This has been shifted to 3404 cm−1 in the case of the biopolymer due to the formation of hydrogen bonds between starch and phloroglucinol.
3.4 X-ray diffraction
As shown in Fig. 3a, a strong diffraction peak was observed around 17.18° 2θ, which indicates B-type crystallinity of starch with a number of spurious peaks near 14°, 22° and 24° 2θ. A similar kind of XRD pattern was reported in Thai yam (Dioscorea hispida).37 For phloroglucinol (Fig. 3b), diffraction peaks at 21.96°, 22.48° and 27.32° 2θ infers the presence of the phloroglucinol molecule, which is similar to phloroglucinol dihydrate (JCPDS No. 65-2971). It is interesting to note that a broad diffraction peak at 18° 2θ infers the formation of the biopolymer with an amorphous structure. This is due to the dropwise addition of acetone during the process of grafting that induces gelatinization resulting in the loss of B-type structural integrity as shown in Fig. 3c.28 According to Tattiyakul et al.38 the moisture content in starch will be transformed during the process of grafting, which eventually breaks the crystallinity of starch.
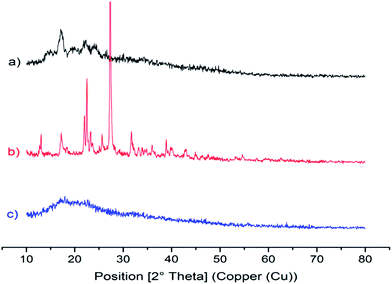 |
| Fig. 3 X-ray diffraction pattern of (a) soluble starch, (b) phloroglucinol, and (c) biopolymer. | |
3.5 Dynamic light scattering measurements
The average particle size distribution and stability (zeta potential) of the biopolymer were examined by dynamic light scattering studies. Recently Kumari et al.39 reported that particles of sizes below 500 nm can traverse the cell membrane by endocytosis. However, in the present study the average size distribution of the biopolymer was found to be 493.3 nm (Fig. 4) when compared to soluble starch, which is above 1000 nm. This will pave way for better cellular uptake of the desired compounds for controlled release in an efficient way. Altogether, the biopolymer dispersed in the aqueous medium exhibit a homogenous and narrow size distribution. In addition, zeta potential measurement was also carried out for the biopolymer. It is reported from the study that phloroglucinol and soluble starch exhibit a negative potential value of −5 mV and −23.3 mV, respectively. However, soluble starch supplemented a net negative charge to the biopolymer (−34.4 mV) and the zeta potential to be more negative (Fig. 5). This negative charge of the biopolymer showed no particle agglomeration in the dispersed medium due to electrostatic repulsion.40 In addition, the particle size observed from the study is in agreement with the scanning electron microscope images.
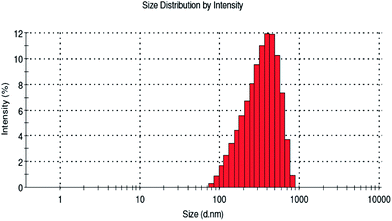 |
| Fig. 4 Particle size distribution of the biopolymer. | |
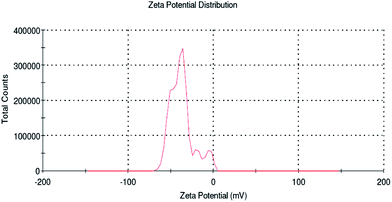 |
| Fig. 5 Zeta potential measurement of the biopolymer. | |
3.6 1H NMR assignment
1H NMR spectra of soluble starch, phloroglucinol and biopolymer are shown in Fig. 6. 1H NMR spectra of soluble starch shows OH and –O–CH protons between 3.5 to 5.5 ppm.41,42 In the spectra of phloroglucinol, two components such as aromatic hydrogen (9 ppm) and aromatic hydroxyl (5.6 ppm) protons were seen.43 A chemical shift in aromatic hydrogen protons of phloroglucinol from 9.0 ppm to 8.4 ppm in the biopolymer showed the process of graft polymerization with starch. This is due to the involvement of hydrogen bonding between the aromatic hydrogen protons of phloroglucinol replacing the ion-pair of sodium alkoxide in starch. This is further confirmed by the chemical shift of the hydroxyl protons of soluble starch from 4.5 to 5.5 ppm in the biopolymer.
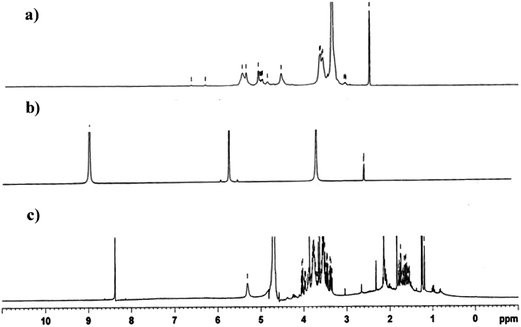 |
| Fig. 6 1H NMR predictions of (a) soluble starch, (b) phloroglucinol, and (c) biopolymer. | |
3.7 In vitro release kinetics of phloroglucinol
The in vitro release kinetics of phloroglucinol from the biopolymer was investigated using a dialysis membrane at room temperature at physiological pH (7.4). The disruption of weak hydrogen bonding between soluble starch and phloroglucinol initiates time dependent release.44 During the start of the reaction (0–15 min), the level of phloroglucinol concentration was undetectable in the phosphate buffer solution. However, a constant increase was observed with a maximum cumulative release of 54.15% during the period of 24 h (Fig. 7). The centrifugal force applied during the process may ensure an even dispersion of phloroglucinol throughout the medium.45 In addition, surface erosion of the encapsulated soluble starch will play a major role in the controlled release of phloroglucinol. Hence, our study demonstrates the slow and possible controlled release kinetics of phloroglucinol into the phosphate buffer solution (pH 7.4).
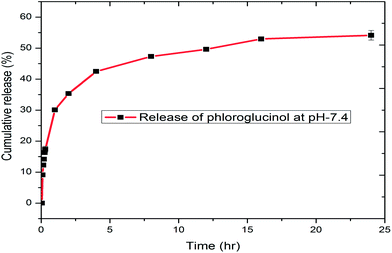 |
| Fig. 7 In vitro release kinetics of phloroglucinol. | |
3.8 Antioxidant properties
Antioxidants are imperative health promoting agents that avoid deterioration of oxidizable products such as food, drugs and cosmetics.46 Intake of these compounds as dietary supplements enhances the body's defense system and reduces oxidative stress. In recent years, the antioxidant activity of phloroglucinol and its derivatives has been better understood.47 The reason is mainly attributed to the presence of three hydroxyl groups that act as hydrogen donors to scavenge free radicals rapidly. However, the antioxidant property of phloroglucinol-encapsulated starch biopolymer is unknown. Hence, in the present study, an effort was made to investigate the antioxidant activities such as DPPH, ABTS+ and reducing power of the biopolymer. DPPH is a stable, long lived-nitrogen centered free radical that can readily accept an electron or hydrogen from any antioxidants to form non-radical DPPH-H.48 It is noted that the biopolymer displayed noticeable radical scavenging activity in a dose-dependent manner (Fig. 8). The EC50 value of the biopolymer was 119.75 μg mL−1 and that of phloroglucinol was found to be 17.40 μg mL−1. However, the soluble starch did not show any radical scavenging ability. The ability of ABTS+ radical scavenging activity is shown in Fig. 9. This method is reflected by the ability of hydrogen or electron-donating antioxidants to scavenge free radicals during the reaction of ABTS+ and potassium persulfate.49 At the end of the ABTS+ assay, biopolymer showed higher antioxidant property (EC50 = 45.45 μg mL−1) as compared to that of soluble starch. The reducing power of the biopolymer was comparatively low when compared to phloroglucinol upon increasing concentration (Fig. 10). The presence of reductones possessing strong antioxidant properties by breaking free radical changes by donating an electron/hydrogen.50
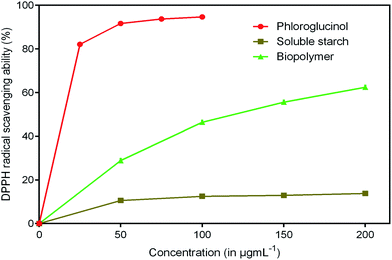 |
| Fig. 8 DPPH radical scavenging activity. | |
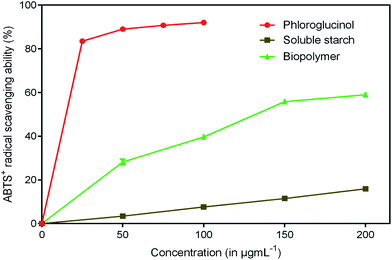 |
| Fig. 9 ABTS+ radical scavenging activity. | |
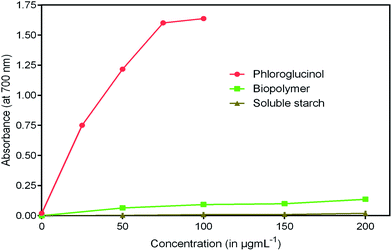 |
| Fig. 10 Reducing power. | |
3.9 Cytotoxicity studies
The cytotoxic effect of the biopolymer was investigated by an MTT (3-(4,5-dimethylthiazol-2-yl)-2,5-diphenyltetrazolium bromide) assay against HepG2 cancer cell lines.51 This assay was based on the ability of mitochondrial dehydrogenase of viable cells to yield a measurable blue formazan product. As shown in the Fig. 11, the killing effect of the biopolymer was found to be in a dose-dependent manner (IC50 = 155.22 μg mL−1). However, the phloroglucinol (IC50 = 254.44 μg mL−1) and soluble starch (IC50 = 859.47 μg mL−1) did not show any significant degree of cytotoxicity. The superior effect of the biopolymer could well be due to the hydrophobicity of the grafted biopolymer that enhances adhesion and adsorption onto the surfaces of cancer cells.39 At the same time, the physiological pH (7.4) within the cancer cell induces the disruption of the hydrogen bonding between starch and phloroglucinol leading to a controlled release of phloroglucinol into the HepG2 cancer cell lines. Several research accomplishments have been made using starch towards targeted drug delivery.52 But till date, no research has shown the cytotoxic effect of the phloroglucinol-encapsulated starch biopolymer. According to Kim et al.53 phloroglucinol exerts protective effects against oxidative stress-induced cell damage in SH-SY 5Y neuroblastoma cell lines. It is also reported that phloroglucinol inhibits HepG2 cancer cell lines at an elevated concentration of 400 μM.54 Further studies conducted on phloroglucinol derivatives illustrate the induction of cell death by the progression of apoptosis in human cancer cell lines.55 It is noteworthy to mention that the concentration of the encapsulated phloroglucinol (10.90 ± 0.66 μg mL−1) within the biopolymer is comparatively low as compared to soluble starch (30.30 ± 0.90 μg mL−1). Further, we confirmed the ability of the biopolymer to inhibit HepG2 cancer cell lines by assessing the cell morphology through phase contrast and fluorescence microscopy. Phase contrast microscopic studies revealed the occurrence of morphological changes such as cell shrinkage and blebbing in respect to the effect of the biopolymer (Fig. 12).56 Altogether, to study the effect of the biopolymer in altering the nuclear morphology of HepG2 cancer cell lines Hoecht staining was performed. Hoechst 33258 is a lipophyllic dye, which is able to permeate cell membranes for visualizing DNA under fluorescence microscopy. Hoechst 33258 effectively binds to AT-rich regions of DNA and exhibits fluorescence.57 Fig. 13 shows the results of Hoechst staining for HepG2 cancer cell lines treated with the biopolymer (IC50 value) at different time intervals (24 and 48 h). The untreated cells showed no sign for apoptosis, whereas the 24 h treated biopolymer displayed apoptotic morphology as a result of condensed chromatin. After 48 h, the effect of biopolymer showed intense apoptotic bodies with intense spots evidencing cell membrane disintegration.58 These significant observations provide valuable evidence of apoptosis. However, the exact molecular mechanism involved in the cell killing effect of the biopolymer needs to be better explained.
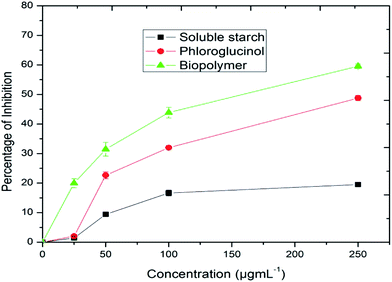 |
| Fig. 11 In vitro cytotoxicity assay. | |
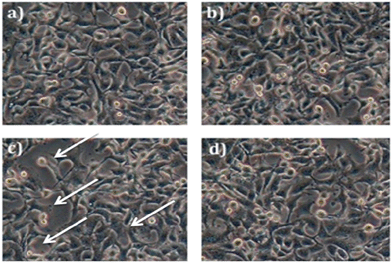 |
| Fig. 12 Morphological assessment of HepG2 cancer cell lines (a) control cells, (b) soluble starch, (c) biopolymer, and (d) phloroglucinol. | |
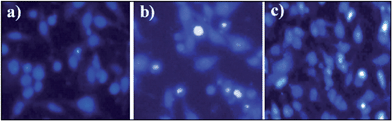 |
| Fig. 13 Hoechst staining at different time intervals of (a) untreated cells, (b) treatment after 24 h, and (c) treatment after 48 h. | |
4. Conclusion
To conclude, the purpose of the present investigation was to develop a novel biopolymer by encapsulating phloroglucinol onto soluble starch by graft polymerization. The process of grafting onto starch was confirmed by FT-IR, SEM, XRD, DLS and 1H-NMR analysis. The encapsulation of soluble starch provides significant protection to phloroglucinol and thereby extending it for controlled release. The experimental investigations of the biopolymer for antioxidant (DPPH, ABTS+ and reducing power) and cytotoxic activities against HepG2 human cell lines were found to be remarkable. There is no report so far on the preparation and biological activity of the biopolymer. However, the present findings will lead to further research on the encapsulation of bioactive compounds and to develop new products for therapeutic applications.
Acknowledgements
The authors are grateful to the Natural Resource Data Management System (DST-NRDMS), Government of India, New Delhi for their financial assistance through the major research project. The authors acknowledge Dr Hairul Islam, Pondicherry Centre for Biological Sciences, Puducherry for his help in cell culture facilities.
References
- K. Park, J. Controlled Release, 2007, 120, 1 CrossRef CAS PubMed.
- Z. Bahadoran, P. Mirmiran and F. Azizi, J. Diabetes Metab. Disord., 2013, 12, 43 CrossRef PubMed.
- C. Manach, A. Scalbert, C. Morand, C. Rémésy and L. Jiménez, Am. J. Clin. Nutr., 2004, 79, 727 CAS.
- K. B. Pandey and S. I. Rizvi, Oxid. Med. Cell. Longevity, 2009, 2, 270 CrossRef PubMed.
- A. Munin and F. Edwards-Lévy, Pharmaceutics, 2011, 3, 793 CrossRef CAS PubMed.
- T. Wang, R. Jonsdottir, H. Liu, L. Gu, H. G. Kristinsson and S. Raghavan, J. Agric. Food Chem., 2012, 60, 5874 CrossRef CAS PubMed.
- I. Pal Singh and S. B. Bharate, Nat. Prod. Rep., 2006, 23, 558 RSC.
- H.-C. Shin, H. J. Hwang, K. J. Kang and B. H. Lee, Arch. Pharmacal Res., 2006, 29, 165 CrossRef CAS.
- M.-J. Ahn, K.-D. Yoon, S.-Y. Min, J. S. Lee, J. H. Kim and T. G. Kim, Biol. Pharm. Bull., 2004, 27, 544 CAS.
- K. Kang, H. J. Hwang, D. H. Hong, Y. Park, S. H. Kim and B. H. Lee, Res. Commun. Mol. Pathol. Pharmacol., 2004, 77, 115 Search PubMed.
- Y. Fukuyama, M. Kodama, I. Miura, Z. Kinzyo, M. Kido and H. Mori, Chem. Pharm. Bull., 1989, 37, 349 CrossRef CAS.
- S. J. Heo, J. Y. Hwang, J. I. Choi, J. S. Han, H. J. Kim and Y. J. Jeon, Eur. J. Pharmacol., 2009, 615, 252 CrossRef CAS PubMed.
- S. J. Heo, J. P. Kim, W. K. Jung, N. H. Lee, H. S. Kang and E. M. Jun, J. Microbiol. Biotechnol., 2008, 18, 676 CAS.
- H. S. Kang, H. R. Kim, D. S. Byun, B. W. Son, T. J. Nam and J. S. Choi, Arch. Pharmacal Res., 2004, 27, 1226 CrossRef CAS.
- K. A. Kang, K. H. Lee, S. Chae, Y. S. Koh, B. S. Yoo and J. H. Kim, Free Radical Res., 2005, 39, 883 CrossRef CAS PubMed.
- Y. Sugiura, K. Matsuda, Y. Yamada, M. Nishikawa, K. Shioya and H. Katsuzaki, Biosci., Biotechnol., Biochem., 2006, 70, 2807 CrossRef CAS.
- N. Y. Yoon, H. R. Kim, H. Y. Chung and J. S. Choi, Arch. Pharmacal Res., 2008, 31, 1564 CrossRef CAS PubMed.
- D. Le Corre, J. Bras and A. Dufresne, Biomacromolecules, 2010, 11, 1139 CrossRef CAS PubMed.
- D. R. Lu, C. M. Xiao and S. J. Xu, eXPRESS Polym. Lett., 2009, 3, 666 Search PubMed.
- P. S. Chow and S. M. Landhausser, Tree Physiol., 2004, 24, 1129 CrossRef CAS.
- J. L. Stern, A. E. Hagerman, P. D. Steinberg, F. C. Winter and J. A. A. Estes, J. Chem. Ecol., 1996, 22, 1273 CrossRef CAS PubMed.
- Y. Zou, Z. J. Qian, Y. Li, M. M. Kim, S. H. Lee and S. K. Kim, J. Agric. Food Chem., 2008, 56, 7001 CrossRef CAS PubMed.
- R. Re, N. Pellegrini, A. Pannala, M. Yang and C. R. Evans, Free Radical Biol. Med., 1996, 26, 1231 CrossRef.
- M. Oyaizu, Jpn. J. Nutr., 1986, 44, 307 CrossRef CAS.
- T. Mosmann, J. Immunol. Methods, 1983, 65, 55 CrossRef CAS.
- S. Yamakawa, A. Demizu, Y. Kawaratani, Y. Nagaoka, Y. Terada, S. Maruyama and S. Uesato, Biol. Pharm. Bull., 2008, 31, 916 CAS.
- K. Ishimoto, M. Arimoto, T. Okuda, S. Yamaguchi, Y. Aso, H. Ohara, S. Kobayashi, M. Ishii, K. Morita, H. Yamashita and N. Yabuuchi, Biomacromolecules, 2012, 12, 3757 CrossRef PubMed.
- Q. Wang, Y. Hu, J. Zhu, Y. Liu, X. Yang and J. Bian, Bull. Mater. Sci., 2013, 35, 415 CrossRef PubMed.
- R. A. D. Graaf, G. Lammers, L. P. B. M. Janssen and A. A. C. M. Beenackers, Starch, 1995, 47, 469 CrossRef.
- S. Hornig and T. Dufresne, Biomacromolecules, 2008, 9, 1487 CrossRef CAS PubMed.
- X. Ma, R. Jian, P. R. Chang and J. Yu, Biomacromolecules, 2008, 9, 3314 CrossRef CAS PubMed.
- M. Labet, W. Thielemans and A. Dufresne, Biomacromolecules, 2007, 8, 2916 CrossRef CAS PubMed.
- R. Kizil, J. Irudayaraj and K. Seetharaman, J. Agric. Food Chem., 2002, 50, 3912 CrossRef CAS PubMed.
- A. T. Tu, J. Lee and F. P. Milanovich, Carbohydr. Res., 1979, 76, 239 CrossRef CAS.
- M. Sekkal, V. Dincq, P. Legrand and J. P. Huvenee, J. Mol. Struct., 1995, 349, 349 CrossRef CAS.
- N. Li, L. Wang, X. He, C. Wan and C. Jiang, Ionics, 2008, 14, 463 CrossRef CAS.
- J. Tattiyakul, T. Naksriarporn and P. Pradipasena, Food Bioprocess Technol., 2012, 5, 964 CrossRef CAS PubMed.
- J. Tattiyakul, T. Naksriarporn, P. Pradipasena and O. Miyawaki, Starch, 2006, 58, 170 CrossRef CAS.
- S. Kumari, S. Mg and S. Mayor, Cell Res., 2010, 20, 256 CrossRef CAS PubMed.
- L. Lin, P. Qiu, X. Cao and L. Jin, Electrochim. Acta, 2008, 53, 5368 CrossRef CAS PubMed.
- C. Fringant, J. Desbrieres and M. Rinaudo, Polymer, 1996, 37, 2663 CrossRef CAS.
- S. D. Zhang, Y. Zhan, H. Huang, B. Yan, X. Zhang and Y. Tang, J. Polym. Res., 2010, 17, 43 CrossRef CAS.
- B. Queguineur, L. Goya, S. Ramos, M. A. Martin, R. Mateos and L. Bravo, Food Chem. Toxicol., 2012, 50, 2886 CrossRef CAS PubMed.
- R. Venkatesan, A. Pichaimani, K. Hari, P. K. Balasubramanian, J. Kulandaivel and K. Premkumar, J. Mater. Chem. B, 2013, 1, 1010 RSC.
- P. Dandekar, R. Jain, T. Stauner, B. Loretz, M. Koch, G. Wenz and C. M. Lehr, Macromol. Biosci., 2012, 12, 184 CrossRef CAS PubMed.
- C. A. Rice-Evans and N. J. Miller, Free Radical Biol. Med., 1996, 20, 933 CrossRef CAS.
- J. Y. Woo and J. Y. Je, Int.
J. Food Sci. Technol., 2013, 48, 1172 CrossRef CAS PubMed.
- X. Lian, X. Wang, Z. Li, Q. Hao and S. Wang, J. Agric. Food Chem., 2010, 58, 11548 CrossRef PubMed.
- A. Serpen, E. Capuano, V. Fogliano and V. Gokmen, J. Agric. Food Chem., 2007, 55, 7676 CrossRef CAS PubMed.
- J. Pouvreau, M. Morancais, F. Taran, P. Rosa, L. Dufosse, F. Guerard, S. Pin, J. Fleurence and P. Pondaven, J. Agric. Food Chem., 2008, 56, 6278 CrossRef CAS PubMed.
- E. Fuhrmann and J. Talbiersky, Org. Process Res. Dev., 2005, 9, 206 CrossRef CAS.
- A. Rodrigues and M. Emeje, Carbohydr. Polym., 2012, 87, 987 CrossRef CAS PubMed.
- M. M. Kim and S. K. Kim, Food Chem. Toxicol., 2012, 50, 2886 CrossRef PubMed.
- S. M. Huang, C. W. Cheung, C. S. Chang, C. H. Tang, J. F. Liu, Y. H. Lin, J. H. Chen, S. H. Ko, K. L. Wong and D. Y. Du, J. Cell. Biochem., 2011, 112, 643 CrossRef CAS PubMed.
- K. A. Kang, K. H. Lee, S. Chae, R. Zhang, M. S. Jung, Y. M. Ham, J. S. Baik, N. H. Lee and J. W. Hyun, J. Cell. Biochem., 2006, 97, 609 CrossRef CAS PubMed.
- R. Zhao, T. Z. Wang, D. Kong, L. Zhang, H. X. Meng, Y. Jiang, Y. Q. Wu, Z. X. Yu and X. M. Jin, World J. Gastroenterol., 2011, 17, 1152 CrossRef PubMed.
- Y. Guo, W. Zhang, Y. Y. Yan, C. G. Ma, X. Wang, C. Wang and J. L. Zhao, J. BUON., 2013, 18, 2 Search PubMed.
- Y. Zakaria, A. Rahmat, A. H. L. Pihie, N. R. Abdullah and P. J. Houghton, Cancer Cell Int., 2009, 9, 16 CrossRef PubMed.
|
This journal is © The Royal Society of Chemistry 2014 |