DOI:
10.1039/C4RA01921K
(Paper)
RSC Adv., 2014,
4, 22288-22293
A selective colorimetric and fluorescent chemosensor for Cu2+ in living cells†
Received
5th March 2014
, Accepted 6th May 2014
First published on 12th May 2014
Abstract
A simple colorimetric and fluorescent chemosensor MP was designed and synthesized and its selective signaling behaviors toward Cu2+ were investigated. Under the optimized conditions, the sensor exhibited specific absorbance-on and fluorescence-on responses to Cu2+ only, which can be tuned for detection with the naked eye. These remarkable properties may allow Cu2+ to be detected directly in the presence of the other examined metal ions. The limit of detection for Cu2+ was up to 0.096 μM. As a fluorescence turn-on sensor, cell experiments showed MP can permeate through cell membranes and react to Cu2+ within living cells.
Introduction
The development of selective and sensitive chemosensors targeting heavy and transition metal cations has received considerable attention because of their important roles in vital movement.1,2 As the third most abundant transition metal after zinc and iron in the human body, copper plays significant roles in many fundamental physiological processes of organisms as a catalytic cofactor for a variety of metalloenzymes, including superoxide dismutase, cytochrome oxidase and tyrosinase.3–5 However, excess copper will cause oxidative stress and disorders associated with neurodegenerative diseases, such as Wilson's disease, Alzheimer's disease, and Menke's disease.6–10 Furthermore, copper can also be a significant environmental pollutant because of its widespread use in industry and agriculture.11 Thus far, chemosensors for copper have been extensively explored owing to its biological significance. Although great achievements have been obtained in the field, some of the reported Cu2+ chemosensors suffered from shortcomings of practical application in terms of complex synthetic procedures, poor selectivity, and low sensitivity.12–18 What's more, it is a challenge to explore more “off-on” chemosensors for copper due to the fluorescence-quenching nature of paramagnetic Cu2+. Therefore, it is significant to develop novel chemosensors which have excellent recognition properties and outstanding practicability. Recently, You and co-workers19 have synthesized a new coumarin-based fluorescent chemosensor, which can selectively and sensitively recognize Cu2+ in aqueous acetonitrile solutions. What's more, their design is the first example of a novel turn-on fluorescent selective probe demonstrating intracellular detection of histidine in “hard-to-transfect” glioblastoma cells. Lu and co-workers20 have reported a mesoporous shell containing fluorescence resonance energy transfer (FRET) type ratiometric Cu2+ fluorescent sensor. The coordination of Cu2+ and its ligand led to the variation of FRET efficiency between neighboring FRET pair benefited from the nanosized pore system of mesoporous matrix, which finally resulted in the ratiometric detection of Cu2+ with excellent sensing properties.
Rhodamine B is an ideal platform for development of colorimetric or fluorescent chemosensors owning to its high absorption coefficient, high fluorescent quantum yield and excitation within visible wavelengths.21 Furthermore, rhodamine derivatives bearing a spirolactam structure are non-fluorescent and colorless (off), whereby ring-opening of the corresponding spirolactam gives rise to strong fluorescence emission and a pink color (on). This phenomenon gives them an excellent potential to construct “off-on” type chemosensors toward Cu2+ by the naked eye. Recently, a few rhodamine based chemosensors, which are driven by visible light excitation and show turn-on response to the targeted metal ions, such as Cu2+, Ag+, Au+, Cr3+, Fe3+, Hg2+ have been proposed.22–29
Our research work involves the design, synthesis, spectroscopy, and biological applications of fluorescent chemosensors for selective sensing of Cu2+ ions. Here we are reporting a new rhodamine based chemosensor MP which was prepared by a conventional three-step synthesis using inexpensive starting materials. Especially, the chemosensor showed highly sensitive and selective fluorescence “turn-on” behaviors toward Cu2+ as well as the remarkable color changes from colorless to pink, which can be visualized by the naked eye. The limit of detection for Cu2+ is up to 0.096 μM. In addition, cell experiments showed MP can permeate through cell membranes and react to Cu2+ within living cells.
Experimental
Apparatus reagents and chemicals
Absorbance spectra measurements were performed on a Shimadzu UV-1700 spectrophotometer. The fluorescence spectra were measured on a HITACHI F-4500 fluorescence spectrophotometer. ESI-MS data were recorded on an AXIMA-CFRTM plus MALDI-TOF mass spectrometer. NMR spectra were recorded on a Varian INOVA-400 MHz spectrometer (at 400 MHz for 1H NMR and 100 MHz for 13C NMR) with tetramethylsilane (TMS) as internal standard. IR spectra were taken in KBr disks on a Bruker Tensor 27 spectrometer. X-ray data were collected on Bruker Smart APEX II CCD diffractometer. Bioimaging of the sensors were performed on an Olympus FV1000 confocal microscopy and the excitation wavelength was set as 550 nm. Column flash chromatography was carried out on Merck silica gel (250–400 mesh ASTM). Thin-layer chromatography (TLC) was performed on silica gel GF254.
The solvents and chemicals used for synthesis were commercially available and were used without further purification unless otherwise noted. The solutions of metal ions were performed from their nitrate or chloride salts. Double distilled water was used throughout the experiment.
Preparation of solutions for absorption and fluorescence measurements
A stock solution of MP (10−4 M) was prepared in methanol. Before spectroscopic measurements, the stock solution of MP was then diluted to the corresponding concentration. Stock solutions of other cation ions were prepared by dissolving corresponding nitrate or chloride salts in water. All the measurements were made according to the following procedure: To 10 mL glass tubes containing the appropriate amount of solution of MP, proper amounts of different metal ions were added, then diluted with methanol–water (3/7, v/v) solution. All samples were shaken for 10 s and waited for 20 min at room temperature before determination. Excitation wavelength was 535 nm. The excitation and emission wavelength bandpasses were both set at 5.0 nm.
Synthesis
Compound 1 and rhodamine B hydrazide 2 were facilely synthesized in high yield according to literatures.30,31
Rhodamine B hydrazide (4.56 g, 10 mmol) was dissolved in 20 mL ethanol, then compound 1 (3.40 g, 10 mmol) was added, then the mixture was stirred for 6 h at room temperature. When the reaction was complete, the solvent was evaporated under reduced pressure, and the residue was purified by column chromatography on silica gel, yield: 45%. 1H NMR (400 MHz, CDCl3) δ (ppm) 11.51 (s, 1H), 9.14 (s, 1H), 8.00 (d, J = 7.1 Hz, 1H), 7.83 (d, J = 7.9 Hz, 3H), 7.74 (s, 1H), 7.60–7.40(m, 5H), 7.18 (d, J = 7.0 Hz, 1H), 6.99 (d, J = 8.8 Hz, 1H), 6.53 (s, 1H), 6.50 (d, J = 5.1 Hz, 3H), 6.28 (d, J = 8.5 Hz, 2H), 3.33 (q, J = 8 Hz, 8H), 1.16 (t, J = 6.7 Hz, 12H). 13C NMR (100 MHz, CDCl3) δ 164.61, 161.64, 153.65, 152.82, 151.31, 151.11, 149.36, 145.75, 133.95, 130.64, 129.58, 129.28, 128.86, 128.26, 127.62, 125.27, 124.36, 123.69, 122.73, 118.88, 118.09, 108.37, 105.19, 98.17, 77.60, 77.29, 76.97, 66.52, 44.59, 12.86. HRMS (ESI) calcd for C41H40N6O3 [M + H]+: 665.3235, found: 665.3263.
Cell culture and fluorescence imaging
The human cancer cell line HepG2 (liver cells) were cultured in RPMI 1640 supplemented with 10% FBS. Immediately before the experiments, cells were pretreated with sensor MP (20 μM) for 1 h at 37 °C in humidified air and 5% CO2, washed 3 times with PBS and imaged. After incubation with CuCl2 (40 μM) for another 2 h at 37 °C, cells were washed 3 times with PBS to remove remaining CuCl2 and imaged.
Results and discussion
Synthesis and structure characterization
As shown in Scheme 1, MP can be facilely synthesized from rhodamine B by a three-step reaction. The target compound MP was fully characterized by 1H NMR, 13C NMR spectra, and Mass spectra. A single crystal of MP was obtained from dichloromethane and methanol solutions (5
:
5, v/v) by slow evaporation at ambient temperature, and a unique spirolactam structure ring formation was observed (Fig. 1).
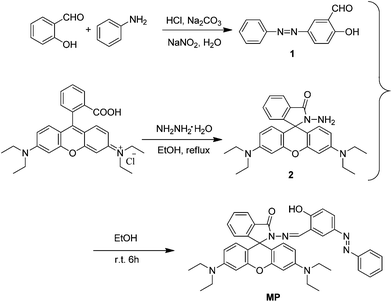 |
| Scheme 1 Synthesis of MP. | |
 |
| Fig. 1 X-ray crystal structure of MP. | |
Spectral characteristics
The effect of methanol content on the fluorescent measurement of Cu2+ was investigated and the results were shown in Fig. S1.† It was found that organic solvent had great effect on the coordination reaction. When methanol content is above 30%, a high and stable fluorescence signal was obtained. Therefore, 30% aqueous methanol solution was chosen in subsequent experiments.
The absorption spectra of MP with varying Cu2+ concentrations in methanol–water (3/7, v/v) solution was shown in Fig. 2. Initially, chemosensor MP was colorless and exhibited almost no characteristic absorption peak of rhodamine moiety, indicating that the spirocyclic form was present predominantly. Upon addition of Cu2+, the absorbance was enhanced obviously and a new peak at 552 nm was observed, with a color change of the solution from colorless to pink (Fig. 3). This indicated that the spriolactam structure MP was induced to form open-ring structure by the addition of Cu2+. The enhancement was saturated with more than 1.0 equiv. of Cu2+, which revealed that the binding mode was most probably of 1
:
1 stoichiometry. According to the data of Job's plots of sensor and Cu2+ (Fig. 2b, inset), the same conclusion was obtained. More direct evidence was obtained by studying the ESI-MS spectra of the components of the mixture of MP and Cu2+ (Fig. S2†). The unique peak at m/z = 726.2370 corresponding to [MP + Cu–H]+ was clearly observed. Moreover, the linear relationships of MP toward Cu2+ could be built with a correlation coefficient of 0.9915 when the concentration of Cu2+ ions did not exceed that of sensor. Based on the definition of detection limit,32 the limit of detection for Cu2+ is up to 0.096 μM (Fig. S3†). Moreover, the dramatical color change ensured MP as a sensitive “naked-eye” sensor for Cu2+.
 |
| Fig. 2 Changes in UV-vis absorption spectra of MP (10 μM) with various amounts of Cu2+ in methanol–water (3/7, v/v) solution. Inset: (a) the linear relationships of MP toward Cu2+ (below 10 μM) (b) Job's plot of MP and Cu2+ (the total concentration was 10 μM). | |
 |
| Fig. 3 Pictures of MP as a selective naked-eye chemosensor (top) and the visual fluorescence emissions by using a UV lamp (365 nm) (bottom) for Cu2+. From the left to right: blank, Fe3+, NH4+, Li+, Ag+, Ba2+, Al3+, Cd2+, Cr3+, Sn2+, Hg2+, K+, Mg2+, Mn2+, Na+, Ni2+, Pb2+, Zn2+ and Cu2+. | |
Furthermore, fluorescence titrations of MP with Cu2+ in methanol–water (3/7, v/v) solution were performed. Upon gradual addition of Cu2+, there was also a new emission band peaked at 588 nm (Fig. 4), which was reasonably assigned to the delocalized xanthene tautomer of the rhodamine group. As expected, a linear increase of fluorescence intensity was observed with increasing Cu2+ concentrations over the range of 0–10 μM (Fig. 4, inset). As shown in Fig. S4,† the association constant K of MP with Cu2+ was estimated to be 5.95 × 104 M−1.33 To study the stability of the complex formed, we also analyzed the chemical reversibility behavior of the binding of MP and Cu2+. Upon addition of ethylenediamine to the mixture of MP (10 μM) and Cu2+ (10 μM), the fluorescence disappeared and the pink solution turned back to colorless owing to the decoordination of Cu2+ (Fig. 5). Thus, MP can be classified as a reversible chemosensor for Cu2+.
 |
| Fig. 4 Changes in fluorescence intensity of MP (10 μM) with various amounts of Cu2+ in methanol–water (3/7, v/v) solution. Inset: the linear relationship of MP toward Cu2+ (below 10 μM), λex = 535 nm, λem = 588 nm. | |
 |
| Fig. 5 Fluorescence intensity changes of MP (10 μM) upon the addition of each equiv. of ethylenediamine with the presence of Cu2+ (10 μM) in methanol–water (3/7, v/v) solution, λex = 535 nm. | |
Selectivity was a very important parameter to evaluate the performance of a fluorescence chemosensor. Therefore, the selectivity experiments were extended to various metal ions. Fig. 6 showed absorption and fluorescence changes of MP in the presence of various metal ions, such as Fe3+, NH4+, Li+, Ag+, Ba2+, Al3+, Cd2+, Cr3+, Sn2+, Hg2+, K+, Mg2+, Mn2+, Na+, Ni2+, Pb2+ and Zn2+. From UV-vis spectra (Fig. 6a), we can clearly observe a new absorption at 552 nm in the presence of Cu2+ ions. In contrast, other metal ions did not lead to any distinct spectral changes. On the other hand, fluorescence spectra (Fig. 6b) was well consistent with that of UV-vis spectra.
 |
| Fig. 6 UV-vis spectra (a) and fluorescence spectra (b) of MP (10 μM) upon addition of different metal ions (10 μM) in methanol–water (3/7, v/v) solution. | |
Similarly, competition experiments in the presence of potentially competitive metal ions were also carried out and the results are shown in Fig. 7. Most of ions gave no visible change and were not found to induce any detectable fluorescence changes even at higher concentrations. These results demonstrated that the recognition of Cu2+ by MP was hardly influenced by other coexisting metal ions.
 |
| Fig. 7 Fluorescence intensity changes of MP (10 μM) upon the addition of various metal ions (20 μM) in and without the presence of Cu2+ (20 μM). The black bars represent the fluorescence response of MP and competing ions: (1) blank; (2) Fe3+; (3) NH4+; (4) Li+; (5) Ag+; (6) Ba2+; (7) Al3+; (8) Cd2+; (9) Cr3+; (10) Sn2+; (11) Hg2+; (12) K+; (13) Mg2+; (14) Mn2+; (15) Na+; (16) Ni2+; (17) Pb2+; (18) Zn2+; (19) Cu2+. The red bars represent the subsequent addition of 20 μM Cu2+ to the above solutions. λex = 535 nm, λem = 578 nm. | |
For practical application, the fluorescence spectra response of MP in the absence and presence of Cu2+ in different pH values were evaluated. As shown in Fig. 8, solution of MP has almost no fluorescence between pH 6 and 12, whereas, it exhibits apparent fluorescence emission when the pH value is smaller than 6, which might be caused by the H+-induced ring opening of rhodamine spirolactam. Upon the addition of Cu2+ ions, there was an obvious fluorescence emission of MP under pH range 6.0–8.0. These results indicated that MP would provide the potential applications in some environmental and physiological regions for the detection of Cu2+.
 |
| Fig. 8 Effect of pH on the reaction of Cu2+ with MP (1 : 1, 10 μM) and only MP (10 μM). λex = 535 nm. | |
Mechanism
To further confirm why the significant absorbance and fluorescence of MP changed, the IR and 1H NMR spectra experiments were carried out. IR titration results clearly supported the proposed ring-opening mechanism. As demonstrated in Fig. S5,† the carbonyl absorption of MP at 1697.63 cm−1 disappeared after Cu2+ was added. These results indicate that carbonyl group is involved in Cu2+ coordination. Addition of Cu2+ resulted in shifting and broadening of the peak at δ 6.6–6.0 ppm (Fig. S6†), which revealed opening of the spirolactam ring on coordination to Cu2+ in the aromatic rings of the xanthenes moiety. The broadening of the peak at δ 9.14 (imine hydrogen) and δ 11.51 (hydroxyl hydrogen) indicated a decrease in electron density at imine nitrogen and hydroxyl group resulting from direct coordination with Cu2+. Thus, in accordance with the 1
:
1 stoichiometry, the possible binding mode between MP and Cu2+ is proposed in Scheme 2.
 |
| Scheme 2 Proposed mechanism for the fluorescent changes of MP upon the addition of Cu2+. | |
Fluorescence imaging
Owing to its favorable spectroscopic properties of the response to Cu2+, MP should be suitable for fluorescence imaging in living cells. HepG2 cells were incubated with sensor MP (20 μM) for 1 h at 37 °C, and no intracellular fluorescence was observed (Fig. 9a). After three times washing with PBS buffer, the cells were incubated with CuCl2 (40 μM) for another 2 h at 37 °C. A significant fluorescence from the intracellular area was observed (Fig. 9b). Bright-field measurements after treatment with Cu2+ and MP confirmed that the cells were viable throughout the imaging experiments (Fig. 9c). These results demonstrate that MP is cell-permeable and effective intracellular imaging agent for Cu2+ ions.
 |
| Fig. 9 Bioimaging application of chemosensors in HepG2 cells. Representative fluorescence images of HepG2 cells treated with MP (20 μM) in either the absence (a) or the presence (b) of 40 μM Cu2+ for 2 h at 37 °C; (c) bright-field image of cells shown in panel; (d) Overlay image of (b) and (c). | |
Conclusion
In summary, a fluorescent chemosensor MP based on rhodamine was synthesized and its chemosensing properties were investigated. Sensor MP can detect Cu2+ by both distinct color changes and switch-on fluorescence. Moreover, this sensor showed highly selective and sensitive responses to Cu2+ over other examined metal ions. Importantly, we have demonstrated the application of MP in biology by imaging intracellular Cu2+ in HepG2 cells. We expect that this sensor would help to promote the studies of Cu2+ in biological systems.
Acknowledgements
This work was supported by the National Natural Science Foundation of China (no. 21172178).
Notes and references
- A. P. de Silva, H. Q. N. Gunaratne, T. Gunnlaugsson, A. J. M. Huxley, C. P. McCoy, J. T. Rademacher and T. E. Rice, Chem. Rev., 1997, 97, 1515 CrossRef CAS PubMed.
- B. Valeur and I. Leray, Coord. Chem. Rev., 2000, 205, 3 CrossRef CAS.
- R. McRae, P. Bagchi, S. Sumalekshmy and C. J. Fahrni, Chem. Rev., 2009, 109, 4780 CrossRef CAS PubMed.
- K. J. Barnham, C. L. Masters and A. L. Bush, Drug Discovery, 2004, 3, 205 CAS.
- J. Li, Y. Zeng, Q. Hu, X. Yu, J. Guo and Z. Pan, Dalton Trans., 2012, 41, 3623 RSC.
- E. L. Que, D. W. Domaille and C. J. Chang, Chem. Rev., 2008, 108, 1517 CrossRef CAS PubMed.
- S. G. Kaler, Nat. Rev. Neurol., 2011, 7, 15 CrossRef CAS PubMed.
- G. L. Millhauser, Acc. Chem. Res., 2004, 37, 79 CrossRef CAS PubMed.
- D. R. Brown and H. Kozlowski, Dalton Trans., 2004, 13, 1907 RSC.
- C. Deraeve, C. Boldron, A. Maraval, H. Mazarguil, H. Gornitzka, L. Vendier, M. Pitie and B. Meunier, Chem.–Eur. J., 2008, 14, 682 CrossRef CAS PubMed.
- R. Krämer, Angew. Chem., Int. Ed., 1998, 37, 772 CrossRef.
- Z. C. Xu, J. Y. Yoon and D. R. Spring, Chem. Commun., 2010, 46, 2563 RSC.
- P. Kaur, S. Kaur, K. Singh, P. R. Sharma and T. Kaur, Dalton Trans., 2011, 40, 10818 RSC.
- L. Yuan, W. Lin, B. Chen and Y. Xie, Org. Lett., 2012, 14, 432 CrossRef CAS PubMed.
- Z. Liu, C. Zhang, X. Wang, W. He and Z. Guo, Org. Lett., 2012, 14, 4378 CrossRef CAS PubMed.
- A. Kumar, V. Kumar, U. Diwan and K. K. Upadhyay, Sens. Actuators, B, 2013, 176, 420 CrossRef CAS PubMed.
- H. S. Jung, P. S. Kwon, J. W. Lee, J. I. Kim, C. S. Hong, J. W. Kim, S. Yan, J. Y. Lee, J. H. Lee, T. Joo and J. S. Kim, J. Am. Chem. Soc., 2009, 131, 2008 CrossRef CAS PubMed.
- V. Chandrasekhar, S. Das, R. Yadav, S. Hossain, R. Parihar, G. Subramanium and P. Sen, Inorg. Chem., 2012, 51, 8664 CrossRef CAS PubMed.
- Q. H. You, A. W. M. Lee, W. H. Chan, X. M. Zhu and K. C. F. Leung, Chem. Commun., 2014 10.1039/c4cc00521j.
- D. Lu, F. Teng, Y. Liu, L. Lu, C. Chen, J. Lei, L. Wang and J. Zhang, RSC Adv., 2014, 4, 18660 RSC.
- M. J. Jou, X. Q. Chen, K. M. K. Swamy, H. N. Kim, H. J. Kim, S. Lee and J. Yoon, Chem. Commun., 2009, 7218 CAS.
- X. Q. Chen, J. Jia, H. M. Ma, S. J. Wang and X. C. Wang, Anal. Chim. Acta, 2009, 632, 9 CrossRef CAS PubMed.
- K. W. Huang, H. Yang, Z. G. Guo, M. X. Yu, F. Y. Li, X. Gao, T. Yi and C. Huang, Org. Lett., 2008, 10, 2557 CrossRef CAS PubMed.
- Z. G. Zhou, M. X. Yu, H. Yang, K. W. Huang, F. Y. Li, T. Yi and C. H. Huang, Chem. Commun., 2008, 3387 RSC.
- Y. Xiang, L. Mei, N. Li and A. J. Tong, Anal. Chim. Acta, 2007, 581, 132 CrossRef CAS PubMed.
- L. Dong, C. Wu, X. Zeng, L. Mu, S. F. Xue, Z. Tao and J. X. Zhang, Sens. Actuators, B, 2010, 145, 433 CrossRef CAS PubMed.
- G. Q. Shang, X. Gao, M. X. Chen, H. Zheng and J. G. Xu, J. Fluoresc., 2008, 18, 1187 CrossRef CAS PubMed.
- W. Shi and H. Ma, Chem. Commun., 2008, 1856 RSC.
- J. Y. Kwon, Y. J. Jang, Y. J. Lee, K. M. Kim, M. S. Seo, W. Nam and J. Yoon, J. Am. Chem. Soc., 2005, 127, 10107 CrossRef CAS PubMed.
- Y. Xiang, A. Tong, P. Jin and Y. Ju, Org. Lett., 2006, 8, 2863 CrossRef CAS PubMed.
- J. Shao, Dyes Pigm., 2010, 87, 272 CrossRef CAS PubMed.
- Z. Xu, L. Zhang, R. Guo, T. Xiang, C. Wu, Z. Zheng and F. Yang, Sens. Actuators, B, 2011, 156, 546 CrossRef CAS PubMed.
- Z. K. Song, B. Dong, G. J. Lei, M. J. Peng and G. Yuan, Tetrahedron Lett., 2013, 54, 4945 CrossRef CAS PubMed.
Footnote |
† Electronic supplementary information (ESI) available: CCDC 989882. For ESI and crystallographic data in CIF or other electronic format see DOI: 10.1039/c4ra01921k |
|
This journal is © The Royal Society of Chemistry 2014 |