DOI:
10.1039/C4RA01170H
(Paper)
RSC Adv., 2014,
4, 15983-15994
Anode-selective coating of titanium(IV) oxide (TiO2) using electrophoretic sulfone-containing click polyester
Received
10th February 2014
, Accepted 6th March 2014
First published on 6th March 2014
Abstract
We synthesized, at room temperature, aliphatic (1–4) and aromatic (5–8) poly(ester-sulfide)s, via a thiol–ene click polymerization of bis(pentenyl)methylsuccinate (BPMSA) and bis(pentenyl)phthalate (BPPh), respectively, with several dithiols including 1,2-ethanedithiol (EtDt), 1,3-propanedithiol (PrDt), 1,4-butanedithiol (BuDt), and 1,5-pentanedithiol (PeDt) (Mn = 0.7–5.2 × 104, Mw/Mn = 1.6–2.6). Subsequent Oxone oxidation of poly(BPPh-alt-BuDt) (7), as an example, led to the corresponding poly(ester-sulfone) 7′ (Mn = 30
000, Mw/Mn = 1.8). We then prepared a composite of 7′ and TiO2 by using electrophoretic deposition (EPD). The TiO2/poly(ester-sulfone) 7′ composite was selectively deposited onto a stainless-steel anode. The electrode's morphology was confirmed by scanning electron microscopy. We characterized the relationship between the structure of the composite-coated electrode and the zeta potential of an N,N-dimethylformamide/alcohol suspension of poly(ester-sulfone) 7′. The measured values, −(33.27–18.3) mV, indicate that the composite had indeed been selectively deposited on the anode. Notably, from the suspension with the negative potential of −33.27 mV, the thickest composite film was obtained. Furthermore, photo-catalytic activity occurred on the surface of the composite when immersed in aqueous rhodamine-B for 1 month.
Introduction
Titanium dioxide (TiO2) is used in a wide range of applications including as a photocatalyst, printing photoconductor, catalyst support, and solar-battery component. In particular, numerous investigations and patents have been published concerning TiO2 as a photocatalyst because, to date, only TiO2 has been found to have practical applications.1–5 Among the major findings, one that is particularly important, is that the photocatalytic activity of TiO2 depends strongly on its morphology (surface area),6–8 crystal phase,9–11 and efficient use of light energy.12,13 Electrophoretic coating of TiO2 onto a variety of metal surfaces is a promising approach for frontier material studies and applications (smart coating). Several investigations have reported on the electrophoretic deposition (EPD) of TiO2 nanoparticles using organic suspensions14a–c or mixtures of organic solvents and water,14d–g but there has been no previous work, to the authors' knowledge, on the anode (+) selective EPD of TiO2. Instead, the EPD of TiO2 nanoparticles without the addition of stabilizers and using water–ethanol mixtures has been achieved at low voltages.14h Also, the EPD of TiO2 without bubble formation has been possible by using mixed water–alcohol suspensions with the addition of compounds such as (C2H5)4NOH and Tiron molecule,14i,14j in which addition of ethanol reduces the zeta potential but prevents water electrolysis. The preparation of TiO2 coatings from aqueous suspensions with the addition of stabilizers such as carboxylic acids and NaOH has also been reported.14h Recently, Boccaccini et al. reported the preparation of TiO2 coatings from suspensions of TiO2 nanoparticles in water without the addition of organic stabilizers has been achieved by not direct current (DC) but alternating current (AC) electrophoretic deposition (AC-EPD).14i In the all reports, TiO2 deposited on the cathode, because the nanoparticles of TiO2 acquired a positive surface charge at pH ca. 4 and the zeta potential of these TiO2 nanoparticles in water has been documented in the literature.14j
Polyesters are interesting candidates for use as coating materials, because of their excellent physical, mechanical, and bioactive properties,15 which are usually produced by condensation of a diol and a dicarboxylic acid or a hydroxylcarboxylic acid,16 or by ring-opening polymerization of a lactone.17 The former method requires severe reaction conditions even when a customized catalyst is used,18 and the latter uses highly strained cyclic monomers, which makes it difficult to synthesize polyesters with specific functionalities and/or properties. During this decade, we have reported on low-temperature polycondensations of diols and dicarboxylic acids,19 which allowed us to use thermally labile monomers that contain functional groups,20 in which we could not observe electrophoretic behavior of the functional polyesters. On the other hand, click chemistry, introduced by Sharpless and co-workers,21–23 has been shown to be an efficient tool for the preparation of a wide range of organic compounds. We have also reported on the syntheses of polyesters that contain main-chain triazole units by Cu(I)-catalyzed click polymerization of dialkynes and diazides24a,24b and sulfide-containing polyesters by thiol–ene click25 polymerization.24c Recently, we unexpectedly found a series of electrophoretic nonionic poly(ester-sulfone)s 1′–4′ (Fig. 1) prepared via oxidation of a series of bis(pentenyl) methylsuccinate (BPMSA)-containing poly(esters-sulfide), poly(BPMSA-alt-dithiols) 1–4 (Scheme 1), via thiol–ene click polymerization of BPMSA with dithiols and subsequent oxidation,24c in which we demonstrated anode-selective coating of a bioactive glass, 45S5 bioglass, on the stainless steel by electrophoretic deposition (EPD). Given the electrical characteristics of sulfones, i.e., electrochemical stability, oxidation potential, and ionic conductivity, we could then selectively deposit the composite onto a stainless steel anode by EPD.
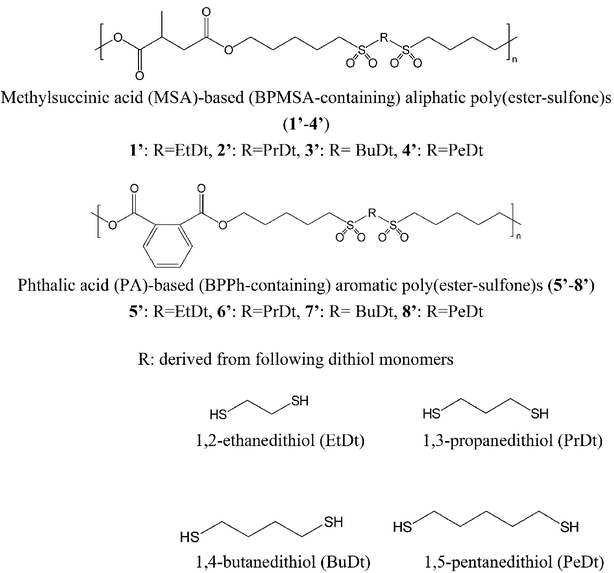 |
| Fig. 1 Chemical structures of aliphatic (1′–4′) and aromatic (5′–8′) poly(ester-sulfone)s used for this study. | |
EPD is a useful technique for the fabrication of inorganic and organic films26 and involves the controlled electric-field-induced deposition of charged particles or molecules onto a metal electrode. Nano- to micrometer-thick films of organic/inorganic composites can be prepared using EPD in an expected thickness of the films,26 in which the thickness was easily controlled by electronic current and voltage, electrophoretic time.
These research background prompted us to explore anode selective coating of TiO2 particle (Fig. 2 and 3). For this article, we demonstrate that aliphatic (1′–4′) and aromatic (5′–8′) poly(ester-sulfone)s can form a composite with titanium(IV) oxide (TiO2), thereby providing a self-cleaning material for EPD. Finally, we demonstrate the photo-catalytic activity of the composite-coated by aromatic (5′–8′) poly(ester-sulfone)s when immersed in aqueous rhodamine-B and compare its activity with that coated by the aliphatic poly(ester-sulfone) (1′–4′).
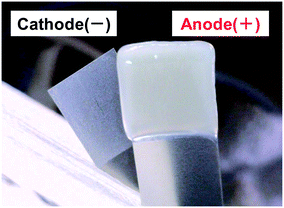 |
| Fig. 2 Anode-selective electrophoretic deposition of the TiO2/poly(ester-sulfone) 7′ (Table 1, entry 7). | |
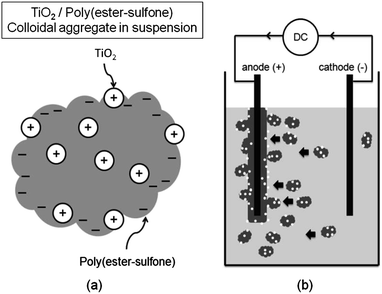 |
| Fig. 3 (a) Schematic of a charged TiO2/poly(ester-sulfone) colloid in an organic solvent (gray background). (b) Schematic of an electrophoretically controlled deposition of the TiO2/poly(ester-sulfone) colloid onto a stainless steal anode. | |
Experimental section
Materials and methods
Chemicals were obtained from commercial sources and used without further purification. 1H-NMR spectra were acquired at 27 °C using a Bruker Analytik DPX200 spectrometer (200 MHz). Tetramethylsilane was the internal standard (0 ppm). The FT-IR spectrum of each poly(esters-sulfone) in a KBr disk was obtained using a JASCO/IR-430 spectrometer. Number average molecular weights (Mn) and polydispersity indexes (Mw/Mn) for the polyesters were measured using a size exclusion chromatography (SEC) system consisting of a Tosoh DP8020 pump system, a Tosoh RI-8020 differential refractometer, and Tosoh TSK-gel α-3000 and α-5000 columns (Tosoh, Tokyo, Japan). The eluent was 0.05% (w/v) LiBr, 100 mM tetramethylethylenediamine in N,N-dimethylformamide (DMF). The flow rate was 0.5 mL min−1, and the temperature was 40 °C. Dynamic light scattering (DLS) measurements were performed using a system equipped with an ALV/SO-SIPD detector and an ALV-7002 correlator. Particle size was measured at a 90°-scattering angle and at 30 °C. A He–Ne laser (λ = 632.8 nm; NEOARK NEO-30MS2) was used as the light source. For the DLS measurements, 20 μL of each composite emulsion [poly(ester-sulfone) 7′ (in DMF–n-BuOH (2/2, v/v) emulsion)] was diluted with 10 mL H2O. The obtained auto-correlation function was analyzed by CONTIN program, and the second-order cumulant method was then used to evaluate the variance μ2/Γ2. Particle size distribution was defined as 1 + μ2/Γ2, which approximately corresponds to the Mw/Mn. The Γ value was transformed into the hydrodynamic radius (Rh) by the Einstein–Stoles equation. Zeta potential measurements were performed using a Zetasizer Nano ZS (Malvern Instruments, UK). Measurement principle was electrophoretic light scattering.
Preparation of bis(pentenyl)phthalate (BPPh)
Phthalic acid (2.5 g, 15 mmol), bis(nonafluorobutanesulfonyl)imide (87 mg, 0.15 mmol), and 4-penten-1-ol (5.167 g, 60 mmol) were added into a 50 mL round-bottom flask. The mixture was stirred at 90 °C for 18 h under reduced pressure (90 mmHg), then diluted with 30 mL chloroform, washed with 30 mL of saturated NaHCO3(aq), and dried over MgSO4. After filtration and solvent evaporation, bis(pentenyl)phthalate (BPPh) was obtained as a pale-yellow liquid in 78% yield (3.5 g). 1H-NMR (200 MHz, CDCl3, δ): 7.78–7.67, 7.59–7.48 (m, O
CC6H4C
O, 4H), 5.96–5.70 (m, C
CHCH2, 2H), 5.14–4.96 (m, CH2
C, 4H), 4.38–4.25 (t, CH2CH2OC
O, 4H, 6.5 Hz), 2.19 (m, C
CHCH2CH2, 4H), 1.84 (q, CH2CH2CH2OC
O, 4H, 6.9 Hz).
Synthesis of the aromatic poly(ester-sulfide)s 5–8 (Table 1, entry 5–8) by photo-polymerization
A series of 10 mL round-bottom flasks were prepared that each contained BPPh (1.0 mmol), 1-hydroxycyclohexyl phenyl ketone (Irgacure 184; 2.0 × 10−2 mmol), and 1,2-ethanedithiol (EtDt), 1,3-propanedithiol (PrDt), 1,4-butanedithiol (BuDt), or 1,5-pentanedithiol (PeDt) (1.0 mmol each) in 1 mL of distilled toluene. The mixtures were each stirred at room temperature under nitrogen and UV-irradiated using a Toshiba H-400P high-pressure mercury lamp (400 W; and −400 nm) for various times. Then the mixtures were individually diluted with CHCl3. The poly(ester-sulfide)s were individually precipitated twice from CHCl3 by addition of n-hexane and dried under vacuum. Pale-yellow syrups were obtained. Details for the syntheses are summarized in Table 1. The polymer preparations were characterized by 1H-NMR spectroscopy and SEC. 1H-NMR (200 MHz, CDCl3, δ): 5, 7.84–7.70, 7.66–7.53 (m, O
CC6H4C
O, 4H), 4.36 (t, CH2CH2OC
O, 4H, 6.5 Hz), 2.82–2.56 (m, SCH2CH2S, 4H), 2.62 (t, CH2CH2CH2S, 4H, 7.0 Hz), 1.95–1.25 (m, O
COCH2CH2CH2CH2 4H, O
COCH2CH2CH2CH2, 4H, O
COCH2CH2CH2CH2, 4H); 6, 7.77–7.65, 7.59–7.46 (m, O
CC6H4C
O, 4H), 4.30 (t, CH2CH2OC
O, 4H, 4.6 Hz), 2.60 (t, CH2CH2SCH2CH2S, 4H, 7.3 Hz), 2.52 (t, CH2CH2SCH2CH2S, 4H, 7.2 Hz), 1.92–1.40 (m, O
COCH2CH2CH2CH2, 4H, O
COCH2CH2CH2CH2, 4H, O
COCH2CH2CH2CH2, 4H, SCH2CH2CH2S, 2H); 7, 7.76–7.65, 7.59–7.46 (m, O
CC6H4C
O, 4H), 4.30 (t, CH2CH2OC
O, 4H, 6.6 Hz), 2.58–2.42 (m, CH2CH2SCH2CH2, 8H), 1.90–1.40 (m, O
COCH2CH2CH2CH2, 4H, O
COCH2CH2CH2CH2, 4H, O
COCH2CH2CH2CH2, 4H, SCH2CH2CH2CH2S, 4H); 8, 7.77–7.62, 7.59–7.44 (m, O
CC6H4C
O, 4H), 4.30 (t, CH2CH2OC
O, 4H, 6.6 Hz), 2.50 (m, CH2CH2SCH2CH2, 8H, 7.0 Hz), 1.84–1.34 (m, O
COCH2CH2CH2CH2, 4H, O
COCH2CH2CH2CH2, 4H, O
COCH2CH2CH2CH2, 4H, SCH2CH2CH2CH2CH2S, 4H, SCH2CH2CH2CH2CH2S, 2H).
Table 1 Poly(ester-sulfide)s and poly(ester-sulfone)s synthesized for this study
Entry |
Ester-containing dialkene |
Dithiol |
Poly(ester-sulfide)a |
Poly(ester-sulfone)c |
|
Mnb (×104) |
Mw/Mnb |
|
Conv.d (%) |
Mnb (×104) |
Mw/Mnb |
Prepared by thiol–ene click polymerization of ester-containing dialkenes (BPMSA and BPPh) and dithiols at room temperature (25 °C) in the presence of Irgacure using UV irradiation. Determined by SEC measurement in DMF relative to poly(styrene) standards. Prepared by Oxone oxidation of the parent poly(ester-sulfide) in DMF at 70 °C for 6 h. Determined by 1H-NMR. |
1 |
BPMSA |
EtDt |
1 |
5.2 |
1.6 |
1′ |
95 |
3.1 |
1.9 |
2 |
BPMSA |
PrDt |
2 |
2.9 |
2.4 |
2′ |
91 |
1.4 |
1.8 |
3 |
BPMSA |
BuDt |
3 |
3.4 |
2.6 |
3′ |
84 |
2.8 |
2.3 |
4 |
BPMSA |
PeDt |
4 |
1.0 |
2.0 |
4′ |
95 |
1.0 |
1.8 |
5 |
BPPh |
EtDt |
5 |
0.7 |
1.8 |
5′ |
>99 |
1.0 |
1.4 |
6 |
BPPh |
PrDt |
6 |
0.9 |
1.8 |
6′ |
>99 |
1.3 |
1.5 |
7 |
BPPh |
BuDt |
7 |
2.4 |
2.4 |
7′ |
90 |
3.0 |
1.8 |
8 |
BPPh |
PeDt |
8 |
1.0 |
1.8 |
8′ |
98 |
1.0 |
1.8 |
Oxone oxidation of the poly(ester-sulfide)s (1–8) to poly(ester-sulfone)s (1′–8′)
An example (Table 1, entry 5) of the oxidation of the poly(ester-sulfide) to the poly(ester-sulfone) follows. Aromatic poly(ester-sulfide) 5 (500 mg, 1.2 mmol repeating unit) and Oxone (1.4 g, 2.2 mmol) in DMF (8 mL) were added into a 30 mL round-bottom flask. After stirring at 70 °C for 6 h, the mixture was filtered, and the solvent was evaporated under reduced pressure to give a white solid that was then dissolved in 5 mL of DMF and precipitated by addition of 90 mL H2O. A total of 471 mg of the poly(ester-sulfone) was obtained as a white solid. The product was characterized by 1H-NMR spectroscopy and SEC. The conversion of the sulfide into the sulfone was calculated using the 1H-NMR intensity ratio of the signals at 4.23 ppm (CH2CH2OC
O) and 3.27 ppm (CH2CH2CH2SO2CH2) for 5′; or 3.31–3.06 ppm (m, CH2CH2SO2CH2CH2, 8H) for 6′; or 3.21–2.99 ppm (m, CH2CH2SO2CH2CH2, 8H) for 7′; or 3.10–2.95 ppm (m, CH2CH2SO2CH2CH2, 8H) for 8′. 1H-NMR (200 MHz, DMSO-d6, δ): 5′, 7.78–7.58 (m, O
CC6H4C
O, 4H), 4.23 (t, CH2CH2OC
O, 4H, 6.1 Hz), 3.51 (s, SO2CH2CH2SO2, 4H), 3.27 (t, CH2CH2CH2SO2, 4H, 7.4 Hz), 1.85–1.60 (m, O
COCH2CH2CH2CH2, 4H, O
COCH2CH2CH2CH2, 4H), 1.56–1.34 (m, O
COCH2CH2CH2CH2, 4H); 6′, 7.70–7.62 (m, O
CC6H4C
O, 4H), 4.23 (t, CH2CH2OC
O, 4H, 6.1 Hz), 3.24 (t, CH2CH2SO2CH2CH2SO2, 4H, 7.1 Hz), 3.15 (t, CH2CH2SO2CH2CH2SO2, 4H, 8.2 Hz), 2.21–1.98 (m, SO2CH2CH2CH2SO2, 2H), 1.84–1.60 (m, O
COCH2CH2CH2CH2, 4H, O
COCH2CH2CH2CH2, 4H), 1.58–1.36 (m, O
COCH2CH2CH2CH2, 4H); 7′, 7.79–7.61 (m, O
CC6H4C
O, 4H), 4.24 (t, CH2CH2OC
O, 4H, 5.5 Hz), 3.21–2.99 (m, CH2CH2SO2CH2CH2, 8H), 1.88–1.60 (m, O
COCH2CH2CH2CH2, 4H, O
COCH2CH2CH2CH2, 4H, SO2CH2CH2CH2CH2SO2, 4H) 1.57–1.36 (m, O
COCH2CH2CH2CH2, 4H); 8′, 7.74–7.53 (m, O
CC6H4C
O, 4H), 4.19 (t, CH2CH2OC
O, 4H, 5.9 Hz), 3.10–2.95 (m, CH2CH2SO2CH2CH2, 8H), 1.78–1.54 (m, O
COCH2CH2CH2CH2, 4H, O
COCH2CH2CH2CH2, 4H, SO2CH2CH2CH2CH2CH2SO2, 4H) 1.54–1.32 (m, O
COCH2CH2CH2CH2, 4H, SO2CH2CH2CH2CH2CH2SO2, 2H).
Preparation of stainless steel electrodes coated with TiO2/poly(ester-sulfone) composites
TiO2 (anatase crystal) powder of mean particle size <25 nm was purchased from Sigma-Aldrich and used without additional purification. A suspension of TiO2 in 7.5 mL of ethanol and 7.5 mL of H2O2 was agitated in an ultrasonicator (BRANSONIC® Yamato 2510) for 15 min and then stirred for 5 min. Each poly(ester-sulfone) was stirred in 15 mL of DMF for 15 min at 60 °C to obtain a homogeneous solution. Then the TiO2 and each poly(ester-sulfone) solution were individually mixed, ultrasonically agitated for 15 minutes, and then stirred for 5 min. For deposition of each TiO2–poly(ester-sulfone) composite onto a 0.2 × 15 × 25 mm stainless steel SUS 301 electrode (The Japan Metal Service Corporation, Saitama, Japan) by EPD, the distance between the electrodes was 7.5 mm and the deposition time was 30–90 s at a current of 2–6 mA. The poly(ester-sulfone)s, prior to EPD and recovered from the electrode after EPD, were characterized by 1H-NMR and FT-IR spectroscopy, and by scanning electron microscopy (SEM) (dual-stage JSM-6010LA microscope; JEOL Ltd., Tokyo, Japan).
Photo-catalytic activity of TiO2/poly(ester-sulfone) on composite films
The ability of the composites to photo-catalyse was investigated by their immersion of composite films in aqueous rhodamine-B (10−5 M). After holding each sample in the solution for 120 minutes under UV (250–400 nm) irradiation, each composite was removed and dried. The solution was characterized by UV, fluorescence measurement, additionally the composite film was characterized by 1H-NMR and SEC.
Results and discussion
Syntheses of sulfone-containing aromatic polyesters
According to our procedure for the synthesis of the poly(ester-sulfone),24c we first attempted to individually prepare sulfide-containing aliphatic polyesters (1–4) by thiol–ene photo-polymerization of BPMSA with different dithiols and with Irgacure 184 as the photo-initiator, under UV irradiation, at room temperature (Scheme 1 and Table 1, entries 1–4). Polymerization proceeded rapidly even under air. Polymerization at 25 °C proceeded and was complete within several hours. Within 15–30 min, each photo-polymerization gave an aliphatic poly(ester-sulfide) of large Mn in good to excellent yields (84–95%, Table 1, entry 1–4). The SEC data demonstrated that the poly(ester-sulfide)s have Mw/Mn values 1.6–2.4 that are commonly found for ordinary A–A- and B–B-type step-growth polymerizations.25
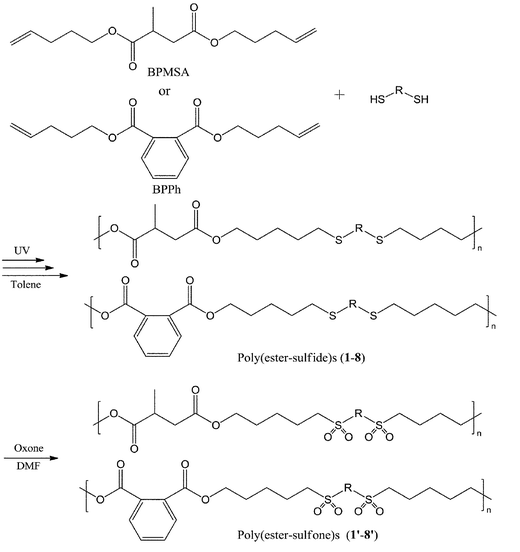 |
| Scheme 1 Synthesis of poly(ester-sulfide) (1–8) and subsequent Oxone oxidation to poly(ester-sulfone) (1′–8′). | |
Additionally, we conducted photo-initiated thiol–ene polymerizations of BPPh and dithiol monomers to produce aromatic poly(ester-sulfone)s (Scheme 1 and entries 5–8 in Table 1). When EtDt and PrDt were used as monomers, the reactions did not produce poly(ester-sulfide)s of large Mns and good yields (Table 1, entries 5 and 6). These poly(ester-sulfide)s would have a short methylene chain between the thiols, and we considered that the steric hindrance between the aromatic units restricted the molecular motion of the poly(ester-sulfide) main chain or that the aromatic ring that absorbs UV light, which inhibit the reactions.22 When we used BuDt as the dithiol, the corresponding poly(ester-sulfide), poly(BPPh-alt-BuDt) (7), with Mn of 2.4 × 104 was synthesized.
Next, we investigated the Oxone oxidation of the poly(ester-sulfide)s 1–8 to produce the corresponding poly(ester-sulfone)s (Table 1, entries 1–8). According to our reported procedure,24c 2 equiv. of Oxone per sulfide quantitatively converted the sulfides to the sulfones. IR absorbance bands (1070–1030 cm−1) ascribable to sulfoxides (the reaction intermediate) were not observed and neither were any 1H-NMR resonances that would be associated with a sulfoxide. However, IR absorbancies at 1271 cm−1 and 1124 cm−1, characteristic of sulfones, were present. The Mns of the poly(ester-sulfone)s 1′–8′ (sulfonation conversion = 100%) was similar to that of its parent poly(ester-sulfide)s 1–8. For example (run 4, Table 1), Mn of poly(ester-sulfone) 4′ (1.0 × 104) was similar to that (1.0 × 104) of the parent poly(ester-sulfide) 4. Moreover, the Mw/Mns for the two polyesters were similar (1.8 and 2.0 respectively), indicating that neither degradation nor side reactions occurred during the oxidation.
Electrophoretic deposition of TiO2/poly(ester-sulfone) coatings
In the case of a bioactive glass, ethanol, rather than n-butanol, more effectively controlled the stability of the suspension of aliphatic poly(ester-sulfone)s 1′–4′.24c Therefore, we selected a film prepared from the DMF–ethanol mixture and the results are summarized in Table 2. Mixtures of TiO2 and a poly(ester-sulfone)s 5′–8′ (Table 1, entries 5–8) actually formed colloidal suspensions in DMF–n-butanol as well as DMF–ethanol, and could be deposited onto a stainless-steel anode by EPD. Notably, virgin TiO2 particles moved towards the cathode (Table 2, entry 1) because these particles are positive charged in mild acidity solutions (pH, 6.2).14i,27 Conversely, we found that not the poly(ester-sulfide)s 1–8 but the poly(ester-sulfone)s 1′–8′ migrated to the anode by virtue of the partially negative charge on the sulfone oxygen, which suggests this charge polarized sulfones dominates the surface charge of the composite. The 1H-NMR spectrum (Fig. 4) of the poly(ester-sulfone) 3′ (Table 1, entry 3) before and after deposition onto the electrode indicates that EPD did not alter its structure/composition. Ordinary aliphatic polyesters, e.g., polycaprolactone (prepared in our laboratory, Mn = 7900, Mw/Mn = 1.30) or polylactic acid, are not charged either, so that when they are part of a TiO2 composite, they are EPD deposited onto the cathode (Fig. 5), which makes the deposition of the TiO2/poly(ester-sulfone)s (prepared in Table 1, entries 5–8) onto the anode an unusual phenomenon. Therefore, the electrophoretic behavior of a TiO2/polyester composite can probably be controlled by the type of polyester.
Table 2 TiO2/poly(ester-sulfone) coating on the stainless steel electrode by EPDa
Entry |
Content |
Poly(ester-sulfone) g L−1 |
Mnb (×104) |
Time (sec) |
Current (mA) |
Electrode |
Image of the electrode deposit |
At a current of 2–6 mA, 30–90 second. Determined by GPC measurement in DMF relative to poly(styrene)s. |
1 |
TiO2 |
0 |
— |
60 |
20 |
Cathode |
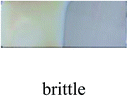 |
2 |
 |
12 |
1.1 |
90 |
4 |
Anode |
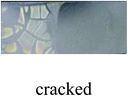 |
3 |
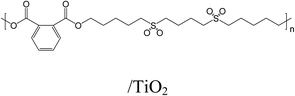 |
6 |
1.1 |
30 |
2 |
Anode |
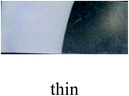 |
4 |
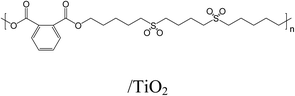 |
6 |
1.1 |
30 |
4 |
Anode |
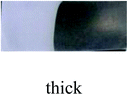 |
5 |
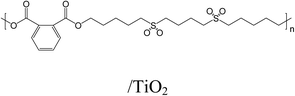 |
6 |
1.1 |
30 |
6 |
Anode |
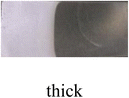 |
6 |
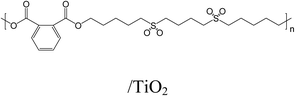 |
6 |
1.1 |
90 |
2 |
Anode |
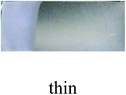 |
7 |
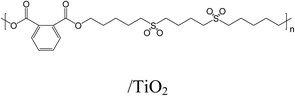 |
6 |
2.2 |
30 |
2 |
Anode |
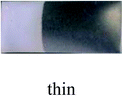 |
8 |
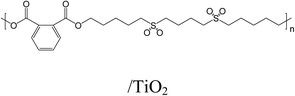 |
6 |
2.2 |
90 |
2 |
Anode |
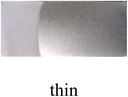 |
9 |
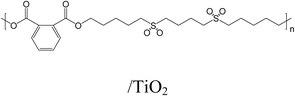 |
6 |
3.0 |
30 |
2 |
Anode |
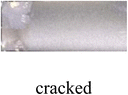 |
10 |
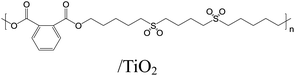 |
6 |
3.0 |
90 |
2 |
Anode |
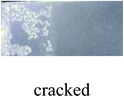 |
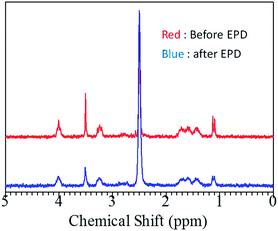 |
| Fig. 4 1H-NMR spectrum of the poly(ester-sulfone) 3′ (Table 1, entry 3) before EPD (red) and after EPD (blue). | |
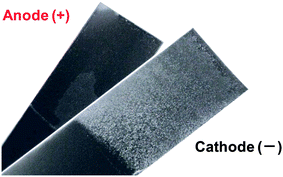 |
| Fig. 5 Cathodic electrophoretic deposition of the TiO2/polycaprolactone. | |
Upon examining the TiO2/poly(ester-sulfone)s 7′ deposited films associated with different EPD conditions, we found cracks in the films prepared from the pure poly(ester-sulfone) 7′ (Mn = 1.1 × 104) suspension (12 g L−1; Table 2, entry 2) and no cracks in the TiO2/poly(ester-sulfone) films prepared from the colloidal suspension that contained 6 g poly(ester-sulfone) per L solvent (Table 2, entries 3–8). When we used higher molecular weight poly(ester-sulfone)s 7′ (Mn = 3.0 × 104) using the optimized condition (polymer concentration, 6 g L−1), the composite films had cracked (Table 2, entries 9 and 10). These results indicated that the molecular weight of poly(ester-sulfone)s 7′ influenced on the EPD coating.
Zeta potential measurements of the poly(ester-sulfone) emulsions
To characterize the electrophoretic behavior of the TiO2/poly(ester-sulfone) 7′-covered anodes, its zeta-potential of poly(ester-sulfone) 7′ was measured in different mixtures of DMF and n-butanol (Table 3). The measured negative values (−33.27–18.3 mV) supported the anode selective deposition, and the larger negative values were, the more stable suspensions were obtained.
Table 3 Zeta-potential measurements of suspensions consisting of poly(ester-sulfone) 7′ in DMF–n-BuOH
Entry |
Content |
Suspension DMF–n-BuOH (v/v) |
Zeta potentiala (mV) |
EPD coating |
Determined by Zetasizer Nano ZS. |
1 |
TiO2 |
2 : 2 |
1.8 |
Cathode |
2 |
 |
2 : 2 |
−33.3 |
Anode |
3 |
 |
3 : 1 |
−18.3 |
Anode |
4 |
 |
1 : 3 |
0.4 |
No coating |
Dynamic light scattering of the poly(ester-sulfone) 7′ emulsion in the absence and presence of TiO2
DLS measurements are also carried out to investigate average particle size of poly(ester-sulfone) 7′ [in DMF–EtOH (2/2, v/v)] emulsions in the absence and presence of TiO2 and their size distributions (Fig. 6). From the DLS analysis, bimodal traces consisting of average size (hydrodynamic radius: Rh) of 2 μm and 200 μm were observed in the poly(ester-sulfone) emulsions in the absence of TiO2. In the presence of the TiO2, unimodal trace was observed with peak top of 2 μm, and the particle size distribution is much broader than that in the absence of TiO2 (Fig. 6). It seems that the particle size is influence by the TiO2. These results indicated that the emulsion is composed of the seed TiO2 coated with poly(ester-sulfone) as the shell.
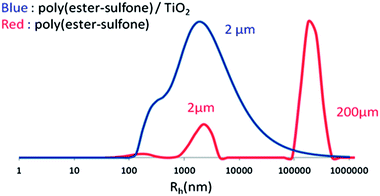 |
| Fig. 6 DLS measurements of poly(ester-sulfone) 7′ in DMF–EtOH (2/2, v/v) emulsions in the absence (red) and presence (blue) of the TiO2. | |
Characterization of TiO2/poly(ester-sulfone) 7′ film prepared by electrophoretic coating
We assessed the surface and cross-section morphology of the TiO2/poly(ester-sulfone) 7′ film by SEM and EDX. The SEM images of the film show that TiO2 particles are well dispersed in a matrix formed by the poly(ester-sulfone) (Fig. 7a and c). Fig. 7b and d show a SEM image and an EDX map for the film, respectively. The colors in the EDX map identify carbon (red), titanium (green), and sulfur (blue) and indicate that the TiO2 particles were homogeneously dispersed, i.e., the poly(ester-sulfone) surrounds and is in close contact with the TiO2 particles. Our results surely indicate that a TiO2/poly(ester-sulfone) colloidal diphasic suspension has formed (see Fig. 6) and that the sulfone moieties were responsible for anode-selective deposition. The composite thickness was 35 μm on average by cross-section SEM observation, and from the EDX mapping of the cross-section, it looks that population of TiO2 in the poly(ester-sulfone) 7′ matrix was uniform (data not shown). As to stability of the surface, the surfaces (Fig. 7e) and the elements population (Fig. 7f) are not changed for 1 month after photo irradiation.
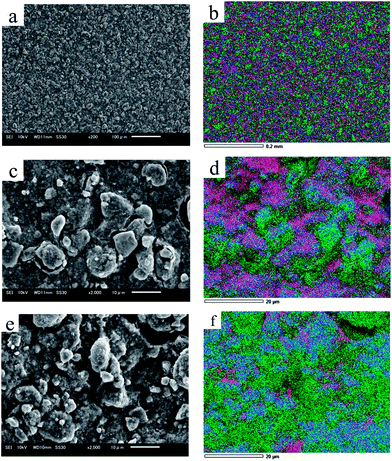 |
| Fig. 7 Scanning electron micrographs of TiO2/poly(ester-sulfone) 7′ films. Surfaces of the composite (a) at low magnification (×200) and (c) at high magnification (×2000). (b) and (d) EDX map, showing the distribution of carbon (red), titanium (green), and oxygen (blue), of the composite (×200, ×2000 magnification). (e) and (f) Same surface of upper ones for 1 month after photocatalytic activity test. | |
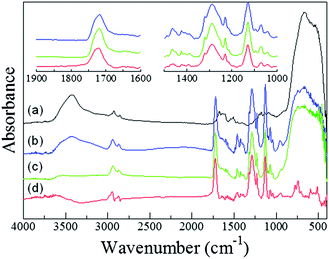 |
| Fig. 8 FT-IR spectra of (a) pure TiO2, (b) TiO2/poly(ester-sulfone) 7′ physical mixture, (c) TiO2/poly(ester-sulfone) EPD coating and (d) pure poly(ester-sulfone) 7′. | |
In the IR spectrum of TiO2/poly(ester-sulfone) after EPD coating (Fig. 8), signal ascribed to TiO2 appeared at 1600 cm−1 and 668 cm−1, which coincided with those of the parent TiO2 (at 1620 cm−1 and 671 cm−1) and broadening of the peak ascribed to poly(ester-sulfone) moiety at 1718 cm−1, 1288 cm−1 and 1129 cm−1 was not observed. The results indicated that the ligand exchange of TiO2 and strong coordination to poly(ester-sulfone) did not occur.
Photocatalytic activity on the surface of electrophoretically deposited TiO2/poly(ester-sulfone) composites
Photocatalytic activity was confirmed on the surface of electrophoretically deposited TiO2/aliphatic and aromatic poly(ester-sulfone)s (3′ and 7′) composites by immersing in 7 mL of 1.0 × 105 M rhodamine-B aqueous solution (Fig. 9). For 120 minutes by UV irradiation, fluorescence of the rhodamine-B aqueous solution disappeared (Fig. 9b–d), indicating self-cleaning ability on the both of aliphatic and aromatic surfaces. However, serious peeling was observed on the TiO2/aliphatic poly(ester-sulfone) 3′ (Fig. 9c and d). On the other hand, the surface of TiO2/aromatic poly(ester-sulfone) 7′ was not changed compared with that before UV irradiation. In the 1H-NMR spectra (Fig. 10, left) and SEC curves (Fig. 10, right) of the aromatic poly(ester-sulfone) 7′ before and after deposition on the electrode (Table 2, entry 8), remarkable changes were not observed either, indicating that photo-degradation of the aromatic poly(ester-sulfone) matrix was not serious during the photoirradiation and the 7′ composite was fixed on the stainless steel even in the aqueous solution.
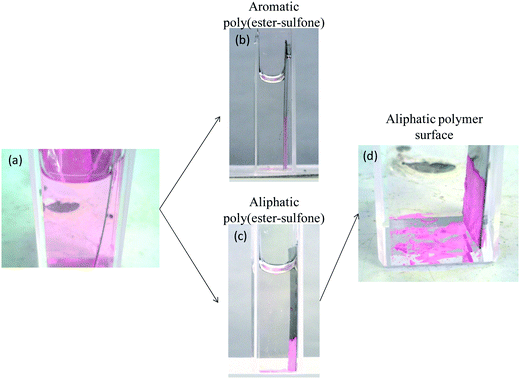 |
| Fig. 9 Photographs of TiO2/aromatic (b) and aliphatic (c) poly(ester-sulfone) (7′ and 3′) before (a) and after photo irradiation [(b–d)]. The poly(ester-sulfone)s were prepared from the parent poly(ester-sulfide)s 7 and 3, respectively, and used for the self-cleaning experiments. | |
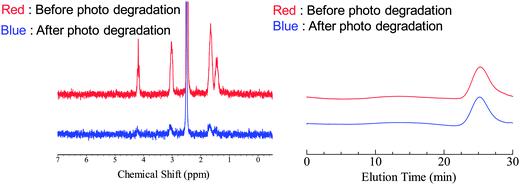 |
| Fig. 10 1H-NMR spectra and size exclusion chromatograms of TiO2/poly(ester-sulfone) 7′ composite before and after photo irradiation. The poly(ester-sulfone) 7′ was prepared from the parent poly(ester-sulfide) 7 used for the chromatography. | |
The photocatalytic activity using rhodamine-B aqueous solution was also shown in profile of fluorescence intensity ratios of initial fluorescence at 580 nm and that after UV irradiation [I(580)/I0(580)] as a function of time (Fig. 11). In both of the previous aliphatic and aromatic poly(ester-sulfone)s (3′ and 7′) composites, the I(580)/I0(580)s decreased and the degradation was completed within 120 min. Interestingly, the degradation rate was comparable for that in the presence of the same amount of virgin TiO2. In order to check the difference of the aliphatic and aromatic components, the degradations of rhodamine-B aqueous solution were also carried out in the presence of MSA and PA (see also Fig. 11), because the corresponding monomers BPMSA and BPPh are not soluble in the aqueous medium. Unexpectedly, the degradations of rhodamine-B aqueous solution were apparently inhibited in the presence of MSA [MSA/TiO2 = 1/1, mol mol−1; Fig. 12(3)] or PA [PA/TiO2 = 1/1, mol mol−1; Fig. 12(2)], in which the remarkable difference in the degradation behaviors between the aliphatic and aromatic carboxylic acid was not observed. Coordination of the carboxylic acid to TiO2 decreased the catalytic activity, which is well-coincided with reported statement that the treatment with organic modifiers having oxygenated functionalities (hydroxyl or carboxyl groups) in a vicinal position inhibits both reductive and oxidative photocatalytic activity of TiO2.28 The results strongly supported that it is essential to use nonionic coating materials for coating of TiO2 to fabricate self-cleaning surfaces.
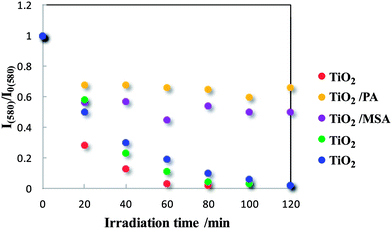 |
| Fig. 11 Photodegradation of rhodamine B on the several TiO2 composite, measured by fluorescence spectroscopy. | |
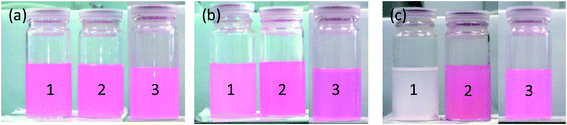 |
| Fig. 12 Investigation of influence of the monomer unit (aliphatic and aromatic carboxylic acids), (1) TiO2, (2) TiO2/phthalic acid (PA), (3) TiO2/methylsuccinic acid (MSA). UV irradiated (a) 0 min, (b) 60 min, (c) 120 min. | |
After the degradation test, the surfaces of both of aliphatic and aromatic poly(ester-sulfone) (3′ and 7′)/TiO2 composites looks red due to the adsorption of rhodamine-B. To the surface, UV irradiation directly performed and after 480 min, the color of the surface turned to be white (Fig. 13). The results revealed that the degradation of rhodamine-B surely occurred on the composite surface.
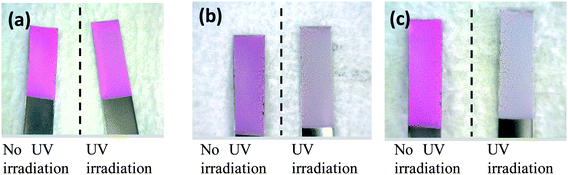 |
| Fig. 13 UV irradiated aromatic poly(ester-sulfone) 7′/TiO2 composites surface (right) in the air for (a) 120 min, (b) 240 min and (c) 480 min. | |
Conclusion
In this study, we prepared a TiO2/click poly(ester-sulfone) colloid, which could be deposited onto a stainless steel surface by EPD to form a soft composite coating. The TiO2/click poly(ester-sulfone) film developed a surface aming at self-cleaning when immersed in rhodamine-B aqueous solution confirming the photocatalytic activity of the new composite coating. The synthesis of the aromatic poly(ester-sulfone) was facile, and the aromatic poly(ester-sulfone)s (5′–8′) were stable in the photo-irradiation as well as the EPD condition, e.g. under electric fields, compared with aliphatic poly(ester-sulfone)s (1′–4′). The results reported herein therefore provide a new guideline of smart coating of TiO2 using electrophoretic coating, aiming at foundation of self-cleaning system.
Acknowledgements
This work was funded by the Ministry of Education, Science, and Culture of Japan (Grant-in-Aid for Development Scientific Research, no. 24550132). The authors are grateful to Dr Kenji Nagata of the Nagoya Institute of Technology for valuable advice and fruitful discussions.
References
-
(a) P. Sarker and P. S. Nicholson, J. Am. Ceram. Soc., 1996, 79, 1987–2002 CrossRef;
(b) J. A. Roether, A. R. Boccaccini, L. L. Hench, V. Maquet, S. Gautier and R. Jérôme, Biomaterials, 2002, 23, 3871–3878 CrossRef CAS PubMed;
(c) I. Corni, N. Neumann, S. Novak, K. König, P. Veronesi, Q. Chen, M. P. Ryan and A. R. Boccaccini, Surf. Coat. Technol., 2009, 203, 1349–1359 CrossRef CAS;
(d) F. Pishbin, A. Simchi, M. P. Ryan and A. R. Boccaccini, Surf. Coat. Technol., 2011, 205, 5260–5268 CrossRef CAS;
(e) H. H. Rodriguez, G. Vargas and D. A. Cortés, Ceram. Int., 2008, 34, 1303 CrossRef CAS;
(f) O. O. Van der Biest and L. J. Vandeperre, Annu. Rev. Mater. Sci., 1999, 29, 327–352 CrossRef CAS;
(g) A. R. Boccaccini and I. Zhitomirsky, Curr. Opin. Solid State Mater. Sci., 2002, 6, 251–260 CrossRef CAS;
(h) R. Ma and I. Zhitomirsky, J. Alloys Compd., 2011, 509, S510–S513 CrossRef CAS;
(i) Y. Wang, I. Deen and I. Zhitomirsky, J. Colloid Interface Sci., 2011, 362, 367–374 CrossRef CAS PubMed;
(j) A. R. Boccaccini, C. Peters, J. A. Roether, D. Eifler, S. K. Misra and E. J. Minay, J. Mater. Sci., 2006, 41, 8152–8159 CrossRef CAS.
- A. Fujishima and K. Honda, Nature, 1972, 238, 37–38 CrossRef CAS PubMed.
- B. O. Regan and M. Grätzel, Nature, 1991, 353, 737–740 CrossRef.
- A. Mills and S. L. Hunte, J. Photochem. Photobiol., A, 1997, 108, 1–35 CrossRef CAS.
- P. D. Cozzoli, A. Kornowski and H. Weller, J. Am. Chem. Soc., 2003, 125, 14539–14548 CrossRef CAS PubMed.
- X. Chen and S. S. Mao, Chem. Rev., 2007, 107, 2891–2959 CrossRef CAS PubMed.
- D. M. Antonelli and Y. J. Ying, Angew. Chem., Int. Ed. Engl., 1995, 34, 2014–2017 CrossRef CAS.
- Y. J. Ying, AIChE J., 2000, 46, 1902–1906 CrossRef.
- J. C. Yu, L. Zhang, Z. Zheng and J. Zhao, Chem. Mater., 2003, 15, 2280–2286 CrossRef CAS.
- M. R. Hoffmann, S. T. Martin, W. Choi and D. W. Bahnemann, Chem. Rev., 1995, 95, 69–96 CrossRef CAS.
- Z. Ding, G. Q. Lu and P. F. Greenfield, J. Phys. Chem. B, 2000, 104, 4815–4820 CrossRef CAS.
- T. V. D. Meulen, A. Mattson and L. Österlund, J. Catal., 2007, 251, 131–144 CrossRef.
- J. Wang, D. N. Tafen, J. P. Lewis, Z. Hong, A. Manivannan, M. Zhi, M. Li and N. Wu, J. Am. Chem. Soc., 2009, 131, 12290–12297 CrossRef CAS PubMed.
-
(a) M. J. Santillan, F. Membrives, N. Quaranta and A. R. Boccaccini, J. Nanopart. Res., 2008, 10, 787–793 CrossRef CAS;
(b) C. K. Lin, T. J. Yang, Y. C. Feng, T. T. Tsung and C. Y. Su, Surf. Coat. Technol., 2006, 200, 3184–3189 CrossRef CAS;
(c) S. Dor, S. Ruhle, A. Ofir, M. Adler, L. Grinis and A. Zaban, Colloids Surf., A, 2009, 342(1–3), 70–75 CrossRef CAS;
(d) W. Tan, X. Yin, X. Zhou, J. Zhang, X. Xiao and Y. Lin, Electrochim. Acta, 2009, 54(19), 4467–4472 CrossRef CAS;
(e) M. J. Santillan, N. E. Quaranta and A. R. Boccaccini, Surf. Coat. Technol., 2010, 205, 2562–2571 CrossRef CAS;
(f) S. Lebrette, C. Pagnoux and P. Abelard, J. Colloid Interface Sci., 2004, 280, 400–408 CrossRef CAS PubMed;
(g) S. Lebrette, C. Pagnoux and P. Abelard, J. Eur. Ceram. Soc., 2006, 26, 2727–2734 CrossRef CAS;
(h) D. Hanaor, M. Michelazzi, P. Veronesi, C. Leonelli, M. Romagnoli and C. Sorrell, J. Eur. Ceram. Soc., 2011, 31, 1041–1047 CrossRef CAS;
(i) A. Chavez-Valdez, M. Herrmann and A. R. Boccaccini, J. Colloid Interface Sci., 2012, 375, 102–105 CrossRef CAS PubMed;
(j) K. Suttiponparnit, J. Jiang, M. Sahu, S. Suvachittanont, T. Charinpanitkul and P. Biswa, Nanoscale Res. Lett., 2011, 6, 27 Search PubMed.
-
(a) R. W. Lenz, Adv. Polym. Sci., 1993, 107, 1–40 CrossRef CAS;
(b) V. V. Koeshak and S. V. Vinogradora, Polyester, Pergamon Press, New York, 1995 Search PubMed.
-
(a) W. H. Carothers and G. L. Dorough, J. Am. Chem. Soc., 1930, 52, 711–721 CrossRef CAS;
(b) E. Takiyama, I. Niikura and Y. Hatano, Japan Pat., 189,823, 1995;
(c) M. Miura, H. Watanabe and M. Fujiwara, Japan Pat., 53, 695, 1995;
(d) P. Buzin, M. Lahcini, G. Schwarz and H. R. Kricheldord, Macromolecules, 2008, 41, 8491–8495 CrossRef CAS;
(e) M. Garaleh, M. Lahcini, H. R. Kricheldorf and S. M. Weidner, J. Polym. Sci., Part A: Polym. Chem., 2009, 47, 170–177 CrossRef CAS.
-
(a) H. R. Kricheldorf and I. Kreiser-Saunders, Macromol. Chem. Phys., 1990, 191, 1057–1066 CrossRef CAS;
(b) A. Lofgren, A. C. Albertsson, P. Dubois and R. Jerome, J. Macromol. Sci., Rev. Macromol. Chem. Phys., 1995, 35, 379–418 CrossRef;
(c) S. Kim, Y. K. Han, Y. H. Kim and S. I. Hong, Macromol. Chem. Phys., 1992, 193, 1623–1631 CrossRef CAS.
-
(a) M. Ajioka, K. Enomoto, K. Suzuki and A. Yamaguchi, Bull. Chem. Soc. Jpn., 1995, 68, 2125–2131 CrossRef CAS;
(b) Ni: M. Mochizuki, K. Mukai, K. Yamada, N. Ichise, S. Murase and Y. Iwaya, Macromolecules, 1997, 30, 7403–7407 CrossRef CAS;
(c) HfCl4(THF2): K. Ishihara, S. Ohara and H. Yamamoto, Science, 2000, 290, 1140–1142 CrossRef CAS PubMed;
(d) Sn distannoxane: M. Ishii, M. Okazaki, Y. Shibasaki and M. Ueda, Biomacromolecules, 2001, 2, 1267–1270 CrossRef CAS PubMed.
-
(a) A. Takasu, Y. Oishi, Y. Iio, Y. Inai and T. Hirabayashi, Macromolecules, 2003, 36, 1772–1774 CrossRef CAS;
(b) A. Takasu, Y. Iio, Y. Oishi, Y. Narukawa and T. Hirabayashi, Macromolecules, 2005, 38, 1048–1050 CrossRef CAS;
(c) A. Takasu, Y. Iio, T. Mimura and T. Hirabayashi, Polym. J., 2005, 37, 946–953 CrossRef CAS;
(d) In water: A. Takasu, A. Takemoto and T. Hirabayashi, Biomacromolecules, 2006, 7, 6–9 CrossRef CAS PubMed;
(e) PLA: A. Takasu, Y. Narukawa and T. Hirabayashi, J. Polym. Sci., Part A: Polym. Chem., 2006, 44, 5247–5253 CrossRef CAS;
(f) Combination with ROP: A. Takasu, H. Tsuruta, Y. Narukawa, Y. Shibata, M. Oshimura and T. Hirabayashi, Macromolecules, 2008, 41, 4688–4693 CrossRef CAS;
(g) Customized catalyst: A. Takasu, T. Makino and S. Yamada, Macromolecules., 2010, 43, 144–149 CrossRef CAS;
(h) Microwave-assisted: S. Yamada and A. Takasu, Polym. J., 2011, 43, 1003–1007 CrossRef CAS.
-
(a) Hydroxyl: A. Takasu, Y. Shibata, Y. Narukawa and T. Hirabayashi, Macromolecules, 2007, 40, 151–153 CrossRef CAS;
(b) Hydroxyl: Y. Shibata, J. Polym. Sci., Part A: Polym. Chem., 2009, 47, 5747–5759 CrossRef CAS;
(c) Mercapto: K. Yamamoto and A. Takasu, Macromolecules, 2010, 43, 8519–8523 CrossRef CAS;
(d) Disulfide: Y. Sato and A. Takasu, Polym. J., 2010, 42, 956–959 CrossRef CAS.
- H. C. Kolb, M. G. Finn and K. B. Sharpless, Angew. Chem., Int. Ed., 2001, 40, 2004–2021 CrossRef CAS.
-
(a) V. V. Rostovtsev, L. G. Green, V. V. Fokin and K. B. Sharpless, Angew. Chem., Int. Ed., 2002, 41, 2596–2599 CrossRef CAS;
(b) T. R. Chan, R. Hilgraf, K. B. Sharpless and V. V. Fokin, Org. Lett., 2004, 17, 2853 CrossRef PubMed.
-
(a) Adhesive polymer: D. D. Diaz, S. Punna, P. Holzer, A. K. Mcpherson, K. B. Sharpless, V. V. Fokin and M. G. Finn, J. Polym. Sci., Part A: Polym. Chem., 2004, 42, 4392 CrossRef CAS;
(b) Polyketone: B. Z. Tang, A. Qin, C. K. W. Jim, W. Lu, J. W. Y. Lam, M. Häussler, Y. Dong, H. H. Y. Sung, I. D. Williams and G. K. L. Wong, Macromolecules, 2007, 40, 2308–2317 CrossRef;
(c) Helical polymer: S. Kobayashi, K. Itomi, K. Morino, H. Iida and E. Yashima, Chem. Commun., 2008, 3019–3021 RSC;
(d) Poly(ethylene glycol): X. M. Liu, A. Thakur and D. Wang, Biomacromolecules, 2007, 8, 2653–2658 CrossRef CAS PubMed;
(e) Biomass-based polymer: T. Michinobu, Y. Inazawa, K. Hiraki, Y. Katayama, E. Masai, M. Nakamura, S. Ohara and K. Shigehara, Chem. Lett., 2008, 154–155 CrossRef CAS;
(f) T. Michinobu, K. Hirai, Y. Inazawa, Y. Katayama, E. Masai, M. Nakayama, S. Ohara and K. Shigehara, Polym. J., 2011, 43, 648–653 CrossRef CAS;
(g) Review: W. H. Binder and R. Sachsenhofer, Macromol. Rapid Commun., 2007, 28, 15–54 CrossRef CAS;
(h) Review: W. H. Binder and R. Sachsenhofer, Macromol. Rapid Commun., 2008, 29, 952–981 CrossRef CAS;
(i) Review: J. A. Johnson, M. G. Finn, J. T. Koberstein and N. J. Turro, Macromol. Rapid Commun., 2008, 29, 1052–1072 CrossRef CAS;
(j) New click chemistry: Y. Koyama, M. Yonekawa and T. Takata, Chem. Lett., 2008, 37, 918–919 CrossRef CAS;
(k) Y. G. Lee, Y. Koyama, M. Yonekawa and T. Takata, Macromolecules, 2009, 42, 7709–7717 CrossRef CAS;
(l) α-Azide-ω-alkyne monomers: S. Binauld, D. Damiron, T. Hamaide, J. P. Pascault, E. Fleury and E. Drockenmuller, Chem. Commun., 2008, 4138–4140 RSC;
(m) D. Binauld, E. Fleury, E. Drockenmuller and F. Huang, J. Polym. Sci., Part A: Polym. Chem., 2010, 48, 2470–2476 CrossRef;
(n) Functional polymer: E. Schwartz, K. Breitenkamp and V. V. Fokin, Macromolecules, 2011, 44, 4735–4741 CrossRef CAS;
(o) Polytriazoleimide: X. Zhou, L. Wan, Y. Hu, Y. E, F. Huang and L. Du, Polymer J., 2010, 42, 216–222 CrossRef CAS.
-
(a) Y. Nagao and A. Takasu, Macromol. Rapid Commun., 2009, 30, 199–203 CrossRef CAS PubMed;
(b) Y. Nagao and A. Takasu, J. Polym. Sci., Part A: Polym. Chem., 2011, 48, 4207–4218 CrossRef;
(c) Y. Nagao, A. Takasu and A. R. Boccaccini, Macromolecules, 2012, 45, 3326–3334 CrossRef CAS.
-
(a) H. T. A. Wilderbeek, J. G. P. Goossens, C. W. M. Bastiaansen and D. J. Broer, Macromolecules, 2002, 35, 8962–8968 CrossRef CAS;
(b) H. T. A. Wilderbeek, M. G. M. Meer, C. W. M. Bastiaansen and D. J. Broer, J. Phys. Chem. B, 2002, 106, 12874–12883 CrossRef CAS;
(c) K. L. Killops, L. M. Campos and C. J. Hawker, J. Am. Chem. Soc., 2008, 130, 5062–5064 CrossRef CAS PubMed;
(d) L. Kwinsnek, S. Nazarenko and C. E. Hoyle, Macromolecules, 2009, 42, 7031–7041 CrossRef;
(e) C. J. Kloxin, T. F. Scott and C. N. Bowman, Macromolecules, 2009, 42, 2551–2556 CrossRef CAS PubMed;
(f) J. Shin, H. Matsushima, J. W. Chan and C. E. Hoyle, Macromolecules, 2009, 42, 3294–3301 CrossRef CAS;
(g) C. Lluch, J. C. Ronda, M. Galia, G. Lligadas and V. Cádiz, Biomacromolecules, 2010, 11, 1646–1653 CrossRef CAS PubMed.
-
(a) P. Sarker and P. S. Nicholson, J. Am. Ceram. Soc., 1996, 79, 1987–2002 CrossRef;
(b) J. A. Roether, A. R. Boccaccini, L. L. Hench, V. Maquet, S. Gautier and R. Jérôme, Biomaterials, 2002, 23, 3871–3878 CrossRef CAS PubMed;
(c) I. Corni, N. Neumann, S. Novak, K. König, P. Veronesi, Q. Chen, M. P. Ryan and A. R. Boccaccini, Surf. Coat. Technol., 2009, 203, 1349–1359 CrossRef CAS;
(d) F. Pishbin, A. Simchi, M. P. Ryan and A. R. Boccaccini, Surf. Coat. Technol., 2011, 205, 5260–5268 CrossRef CAS;
(e) H. H. Rodriguez, G. Vargas and D. A. Cortés, Ceram. Int., 2008, 34, 1303 CrossRef CAS;
(f) O. O. Van der Biest and L. J. Vandeperre, Annu. Rev. Mater. Sci., 1999, 29, 327–352 CrossRef CAS;
(g) A. R. Boccaccini and I. Zhitomirsky, Curr. Opin. Solid State Mater.
Sci., 2002, 6, 251–260 CAS;
(h) R. Ma and I. Zhitomirsky, J. Alloys Compd., 2011, 509, S510–S513 CrossRef CAS;
(i) Y. Wang, I. Deen and I. Zhitomirsky, J. Colloid Interface Sci., 2011, 362, 367–374 CrossRef CAS PubMed;
(j) A. R. Boccaccini, C. Peters, J. A. Roether, D. Eifler, S. K. Misra and E. J. Minay, J. Mater. Sci., 2006, 41, 8152–8159 CrossRef CAS.
- H. Noguchi, A. Nakajima, T. Watanabe and K. Hashimoto, Environ. Sci. Technol., 2003, 37, 153–157 CrossRef CAS PubMed.
- I. Corazzari, S. Livraghi, S. Ferrero, E. Giamello, B. Fubini and I. Fenoglio, J. Mater. Chem., 2012, 22, 19105–19112 RSC.
|
This journal is © The Royal Society of Chemistry 2014 |
Click here to see how this site uses Cookies. View our privacy policy here.