DOI:
10.1039/C4RA00618F
(Paper)
RSC Adv., 2014,
4, 16362-16367
Aligned carbon nanotube/copper sheets: a new electrocatalyst for CO2 reduction to hydrocarbons†
Received
21st January 2014
, Accepted 3rd March 2014
First published on 13th March 2014
Abstract
We controlled the morphologies of copper (Cu) nanostructure on aligned carbon nanotube (CNT) sheets, influencing the efficiency of the electrocatalytic reduction of CO2. Functionalized CNT sheets affected the pulsed electrodeposition of copper in terms of 3D growth, bonding, and electrochemical activity. CNT/Cu sheet electrocatalyst shows high performance in electrochemical reduction of CO2 to hydrocarbons at room temperature and atmospheric pressure. Reduction products were carbon monoxide (CO), methane (CH4), and ethylene (C2H4) gases. Carbon monoxide yields (178 μmol cm2 mA−1 h−1) and methane yields (346 μmol cm2 mA−1 h−1) at oxygen-plasma-treated CNT/Cu sheet electrodes were remarkably higher than other CNT/Cu and CNT sheets. Experimental results also show 3D morphology of copper growth on CNT sheets may play a critical role in hydrocarbon products from CO2.
Introduction
Extrapolating efficient macroscopic materials from individual carbon nanotubes (CNTs) can maintain and/or enhance individual properties such as high specific conductivity,1 chemical inertness,2 high surface area,3 extremely high mechanical strength, and high Young's modulus.4 However, extrapolating these properties to macroscopic scale is still challenging. One of the promising scale-up methods was recently established by multilayers of dry-spun CNT sheets.5 A super-aligned CNT array, created via chemical vapor deposition (CVD), was pulled6,7 into highly aligned free-standing sheets of multi-walled CNTs.8,9 This process does not require any polymer binder and results in a flat, flexible film with low density and aligned graphitic structure. Transparency and electrical conductivity of CNT sheets10 has opened new applications including capacitors,11 batteries,12 and solar cells.13–15
CNT sheets are electrocatalytically stable, sp3-functionalizable, physically flexible/bendable, stackable with multilayers, much lighter than metal, self-supportable, and scalable. Van der Waals forces on CNT sheets can attract CO2 through physisorption.16 Thus, deposition of copper on highly aligned CNT sheets can be an effective way to utilize the desirable traits of both CNT sheets and copper for electrochemical reduction of CO2.
Metal and semi-conducting metals are well studied as electrocatalytic/photocatalytic materials for carbon dioxide reduction to hydrocarbons.17–21 For example, copper, iron, tin, gold, and platinum as a cathode are effective electrocatalysts to reduce CO2 to hydrocarbons and alcohols. In particular, copper is considered the most appropriate candidate for electrocatalytic processes due to its CO2 reduction properties. Copper can electrocatalytically reduce CO2 to hydrocarbons (mainly methane and ethylene) with reasonable current density (i.e. 5–10 mA cm−2) and current efficiency (i.e. >69% at 0 °C) in aqueous electrolyte.17,22 Different atomic lattice configurations of the copper provide controllable selectivity of reduction products with higher activity.23 Metal and semi-conducting metal-coated CNT particles have recently been studied for CO2 reduction.24,25 However, synthesizing metal–CNT composite particles requires complex steps26,27 including dispersion in solvent, reaction, and drying, in order to bond to a conducting substrate. These synthesized particles can break from the substrate electrode and float around (known as pulverization), so that electrocatalytic efficiency eventually decreases.
Here, we report, for the first time, a CO2 reduction using electrodeposited copper nanoparticles on highly aligned CNT sheets. Multi-walled carbon nanotube (MWCNT) arrays were grown using CVD, spun, and stacked into sheets. CNT sheets were pretreated through various functionalization methods and copper was electrochemically deposited. The efficiency of CO2 reduction to hydrocarbons was investigated with CNT/Cu sheets.
Results and discussion
Assembling of CNT/Cu sheets
The CNT sheets were produced from MWCNT arrays using a setup built at the University of Cincinatti,8,28 as shown in Fig. 1a. The sheets were drawn from 0.5 mm high CNT arrays, synthesized by a water-assisted chemical vapor deposition (CVD) process.6,29,30 Ethylene (C2H4) gas was used as a carbon precursor in order to obtain spinnable CNT arrays. The synthesized CNT arrays are aligned perpendicularly to the substrate, which consisted of several layers (Si/SiO2/Al2O3/Fe alloy catalyst). A free-standing sheet of MWCNTs was made by pulling a bundle of nanotubes from one side of the aligned CNT array. The sheet was then attached to a Teflon™ belt and drawn at a rate of 17 mm s−1. The rotation of the belt causes accumulation of the formed CNT ribbon on the Teflon™ and formation of a multi-layered sheet with controllable dimensions and thickness. The array on the platform of the setup was linearly translated by one half of its width per revolution of the belt, thus, allowing overlapping of the previously laid ribbon and securing lateral uniformity within the sheet. The fabricated 200-layer CNT sheets were densified layer by layer using acetone while located on the belt, and exposed to tension caused by the drawing procedure. This helped to maintain the longitudinal nanotube orientation and the original dimensions obtained during densification (Fig. 1b). The 200-layer CNT sheets were characterized by scanning electron microscopy (SEM). The obtained images revealed good tube alignment and a cross-section thickness of about 10 μm (Fig. 1b and c). The CNT sheets were electrically connected to a 2 cm × 2 cm × 10 μm PCB (printed circuit board) and the measured end-to-end resistance was typically <1 Ω (Fig. 1d). Thus, the CNT sheets can be directly used as a conductive layer of electrocatalyst for CO2 reduction, which can simplify the fabricating process of the CNT/Cu sheet electrocatalyst. The detailed fabrications are discussed in the Methods section.
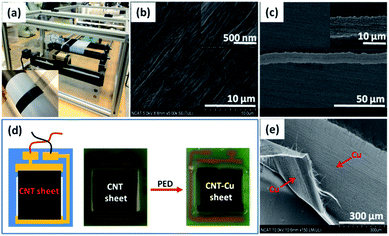 |
| Fig. 1 Fabrication of a highly aligned CNT/Cu sheet electrode. (a) Manufacturing facility for drawing of flexible CNT sheets from a vertically aligned CNT array, (b) SEM image of P-CNT sheet alignment, (c) cross section thickness of P-CNT sheets consisting of 200 layers, (d) schematic and pictures of O-CNT/Cu sheet electrode before and after Cu deposition, and (e) deposited Cu electrode on both sides of an O-CNT sheet. | |
The 200-layer CNT sheets were studied in three ways: (1) pristine CNT (P-CNT), (2) O2 plasma treatment (O-CNT), and (3) O2 plasma pretreatment and electrochemical redox cycling (OE-CNT). Raman spectra (Fig. S1†) show that defect/graphite ratios (ID/IG ratios) of P-CNT sheets, O-CNT sheets, and OE-CNT sheets were 0.83, 1.15, and 1.25, respectively.31 The O-CNT sheets exhibit electrochemical activity of high current due to the removal of amorphous carbon and organic contaminants and the reactivity of sp3-defected sites by O2 plasma (Fig. S2†). Copper(II) sulfate (CuSO4·5H2O) solution (0.1 mM, pH 2.8) was used as the Cu precursor and the three different CNT sheets were pulse-electrodeposited for 5 min to obtain the CNT/Cu sheets. Fig. 1d schematically shows a CNT/Cu sheet electrocatalyst device. The Cu particles were deposited on both sides of the CNT sheets (Fig. 1e). In this work, CNT/Cu sheets were used directly without using any binder or metal current layer as an electrocatalyst.
Surface morphology of the three different CNT/Cu sheets
Fig. 2 shows morphological differences of Cu structure between the three CNT/Cu sheets in terms of size, shape and distribution.
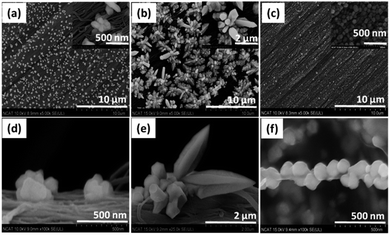 |
| Fig. 2 Scanning electron microscopy images of Cu modified on the three different CNT sheets. (a) P-CNT/Cu sheets, (b) O-CNT/Cu sheets, (c) OE-CNT/Cu sheets, and (d–f) side views of P-CNT/Cu sheets, O-CNT/Cu sheets, and OE-CNT/Cu sheets. | |
The Cu was deposited, coalesced, and formed crystalline islands (grain formation and anisotropic growth) on P-CNT sheets and O-CNT sheets. However, the Cu of O-CNT/Cu sheets was larger and more homogeneous. Cu2+ ions were reduced and deposited quickly on O-CNT sheets. The presence of weakly bound oxygen atoms and oxidized defects at the CNT sheets surface created by O2 plasma induces the formation of Cu–O bonds32 and further promotes Cu nucleation for strong Cu pinning.33 The electrons in the CNT sheet were localized to Cu, which continue to grow Cu cluster.34 The OE-CNT sheets were completely covered with Cu as agglomerated spheres (Fig. 2c). Electrochemical redox cycling preceded by O2 plasma pretreatment might increase the defect area of CNT sheets and further chemically activate the sites for Cu deposition.33,35 From the population density, surface area and diameter estimation from SEM images36 (top view in Fig. S3b† (25
000
:
1), side view in Fig. 2d–f), the Cu of P-CNT/Cu sheets shows a spherical bundle shape with an average diameter of about 130 nm for spheres in each bundle. The Cu of O-CNT/Cu sheets is of cylindrical shape of different sizes, with an average diameter of about 400 nm and height 1 μm. The Cu of OE-CNT/Cu sheets shows hemispherical shapes with an average diameter of about 80 nm on the CNT sheets. Assuming these shapes and considering that Cu particles were present on both sides of the three different CNT sheets, surface areas of Cu particles were calculated to be approximately 5, 25, and 12 cm2, respectively. The CNT sheet coverage ratios of Cu particles of P-CNT/Cu, O-CNT/Cu, and OE-CNT/Cu were about 0.2, 0.5, and 1, respectively. We define the coverage ratio as the ratio of the Cu area which has adhered to a CNT sheet (covered area) to the area of the CNT sheet (Fig. S3†). This ratio was calculated from the same SEM images. The average grain size for the Cu deposited on CNT sheets is about 37.8 nm, calculated using the Debye–Scherrer equation on (111), (200) reflections37–40 from X-ray diffraction (XRD) patterns (Fig. S4†). XRD patterns show that only Cu peaks were observed. The calculated deposited levels of Cu using energy dispersive X-ray spectroscopy (EDS) are presented in Table S3.† The Cu contents are 2.72% for P-CNT/Cu, 3.0% for O-CNT/Cu, and 30.8% for OE-CNT/Cu sheets. The OE-CNT/Cu sheets which were completely covered by Cu particles showed the largest relative Cu content. Though the detailed mechanism of the electrochemical formation of the Cu particles on the CNT sheets is not clear, the surface of the three different CNT sheets is believed to play an important role under identical electrochemical deposition conditions. The functionalization methods of the CNT sheets contribute to the formation of the Cu nanoparticles because the 3D network on the surface provides a large surface area as well as many electroactive sites for spatial distribution of nucleation and anisotropic growth.
Electroactivity of electrocatalyst surfaces
Fig. 3 presents cyclic voltammograms of P-CNT sheets and the three different CNT/Cu sheets, recorded between +1.3 and −1.5 V without stirring, in N2- and CO2-saturated 0.1 M NaHCO3 solutions.19,41–44 The cyclic voltammograms for CNT/Cu sheets show several features including one anodic peak (O-CNT/Cu sheets in CO2: +0.03 V), which is an indication of Cu oxide or hydroxide film formation, as well as two large cathodic peaks (O-CNT/Cu sheets in CO2: −0.175 V and −0.36 V). These two peaks are attributed to the reduction of the layer formed during the anodic sweep voltammogram, which results in the re-deposition of Cu.45,46
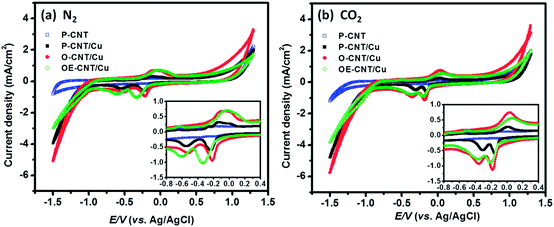 |
| Fig. 3 Cyclic voltammograms of the P-CNT sheets and the three different CNT/Cu sheets in 0.1 M NaHCO3 solution at a scan rate of 10 m V−1 s−1. (a) N2-saturated solution and (b) CO2-saturated solution. | |
The voltammograms also shifted all the current–potential response in the positive direction because of the pH decrease in CO2 saturated solution (pH = 7.6 to 6.7).19,47,48 The results show that the current density of the O-CNT/Cu sheets was the highest. The O-CNT/Cu sheets with large activated surface area and 3D Cu clusters might provide sufficient spatial contact with large diffusion area for electrocatalytic reduction of CO2.
Electrocatalytic reduction of CO2
CO2 reduction products of P-CNT sheets and three different CNT/Cu sheets were determined by the application of various currents in CO2 saturated 0.1 M NaHCO3 (380 mL) solution (pH 6.7). The gas products from the P-CNT sheets and three different CNT/Cu sheets are summarized in Fig. 4 and Tables S4–S7.† As shown in Fig. 4a, a small amount of hydrogen was observed for P-CNT sheets. Hydrogen evolution was not affected by either total surface area of Cu particles or CNT sheet coverage ratios (Fig. S3†). CO2 from deposited Cu particles on the CNT sheets was electrocatalytically reduced to CO, CH4, and C2H4 in aqueous solution. CH4 and CO were major products and C2H4 a minor product in all CNT/Cu sheets. These observations are in good agreement with results of Hori et al.49 as well as many other authors,19,50,51 who compared the electrochemical reduction of CO2 to hydrocarbons at Cu electrodes. According to the literature,23 the CO2 reduction depends on Cu's atomic configuration. On Cu (111), CH4 formation was favored and CO formation was reduced. The C2H4 formation was more favorable on Cu (100). Carbon monoxide (CO) and methane (CH4) were found to be the major products using a Cu (poly) metal foil electrode as an electrocatalyst.52–54 As shown in the XRD results (Fig. S4†), Cu particles have a dominant Cu (111) orientation in the three different CNT/Cu sheets. However, the ratio of CO2 reduction products for these CNT/Cu sheets is very different for the various structures of Cu particles on CNT sheets.
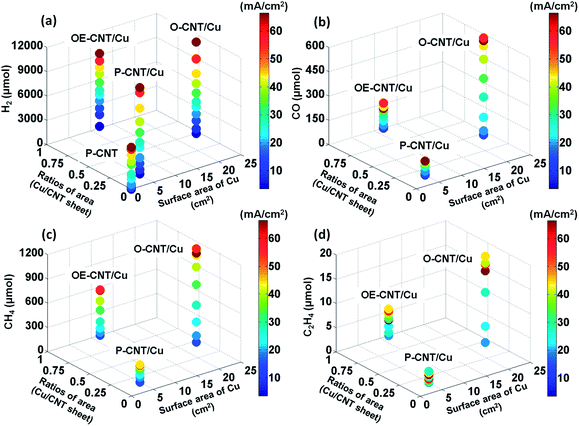 |
| Fig. 4 Electrocatalytic reaction efficiency and correlation between surface area of the deposited Cu particles and the CNT sheet coverage ratios. (a) H2 evolution, (b) CO, (c) CH4, and (d) C2H4 formation of P-CNT sheets and the three different CNT/Cu sheets in CO2 saturated 0.1 M NaHCO3 solution. | |
The highest amounts of reduction products were acquired from the O-CNT/Cu sheets in the range 50–60 mA cm−2. The smallest amounts of reduction products were acquired from the P-CNT/Cu electrode because of the low surface area of Cu particles and coverage ratios of Cu particles on CNT sheets. Even though OE-CNT/Cu sheets have a large density of Cu agglomerated spheres and surface coverage (Fig. 2c), the amounts of reduction products of the CO2 were much smaller than those of O-CNT/Cu sheets. We found that the surface area of the Cu particles was a more important factor in CO2 reduction than the coverage area of Cu particles on CNT sheets. Further, the 3D structure of Cu particles on CNT sheets might have better diffusion behavior of CO2 to Cu/CNT sheet electrode. CNT/Cu sheets could be engineered into flexible, light, stackable and scalable electrocatalytic devices for commercialization.
Conclusions
We have developed high-efficiency electrocatalysts using highly aligned CNT/Cu sheets. Cu morphology on the CNT sheets was controlled through the functionalization for the electrocatalytic reduction of CO2 to hydrocarbons. Reduction products were carbon monoxide (CO), methane (CH4), and ethylene (C2H4) gases. CNT/Cu sheets are flexible/bendable, stackable in multilayers, reusable, much lighter than metal and scalable, and can be applied to various electrocatalytic systems and devices for environmental and energy applications.
Methods
Pretreatment
The O2 plasma treatment of CNT sheets (O–CNT) was performed in a discharge chamber (M4L™ RF gas plasma system, PVA TePla America). The 200-layer pristine CNT sheets were exposed to the plasma produced from O2 (50 sccm) and Ar (50 sccm) gas with a nominal power of about 35 W. The pressure was fixed at 500 mTorr. Typically, the exposure lasted for 5 min. The other pre-treatmented electrode (OE-CNT) has been activated by cyclic voltammetry (CV) of 10 cycles under ±1.5 V after O2 plasma treatment under the same conditions.
Pulsed electrochemical deposition
To perform pulsed electrochemical deposition of Cu on functionalized CNT sheet electrodes, 0.1 mM CuSO4·5H2O was used at a pH of 2.8 and adjusted by 0.1 M H2SO4 with KCl. Electrodeposition was performed using a potentiostat (Gamry Instruments, USA). A pristine CNT sheet of size 2 cm × 2 cm × 10 μm served as the working electrode. The counter electrode was a platinum plate of 2 cm × 2 cm, and a Ag/AgCl electrode was used as a reference electrode. The experiments were conducted at atmospheric pressure and room temperature (25 ± 1 °C) and solutions were pre-purged with N2 for 15 min to remove O2. Each CNT sheet electrode was immersed in the solution for 5 min to keep stable. The pulsed electrochemical deposition of the CNT sheets was performed at −0.8 V and 5 Hz for 5 min, which was previously determined to be the duration to achieve the best electrocatalytic performance. Finally, the CNT/Cu sheets were rinsed with DI water and ethanol.
Electrochemical reduction
The electrochemical reduction experiments were conducted in a three-electrode electrochemical cell. A 0.1 M NaHCO3 (380 mL) electrolyte was saturated with ultra-pure CO2 (99.9999%, Airgas, USA) for 1 h (298 K, pH 6.7). During the CO2 reduction, the electrolyte was constantly stirred. At the end of the electrolysis, after 15 min reaction, the gaseous products were immediately sampled and analyzed by gas chromatography. The gas chromatograph was equipped with a Hayesep-D and MS13X column and HID (helium ionization detector). Experiments for each electrocatalyst were performed after a purge of 1 h under a continuous flow rate of 10 mL min−1 CO2 with electrochemical reduction conducted for 15 min. Aqueous products were not considered in this work. Data were plotted using MATLAB.
Characterization
Photographs. All photographs in this work were taken with a digital camera (Nikon, D3100).
SEM. Scanning electron microscope (SEM) measurements were performed on a Hitachi 8000 instrument. The images were taken in secondary electron mode with an accelerating voltage of 10 kV and working distance of 8 mm. Samples were coated with Au to prevent charging and were mounted on Al stages with conductive carbon tape.
EDS. Energy-dispersive X-ray spectroscopy (EDS) measurements were performed with a Bruker AXS (XFlash detector 5030) attachment on a Hitachi 8000 instrument. The images were taken in secondary electron mode, with an accelerating voltage of 10 kV, current of 10 mA, and working distance of 15 mm. Samples were coated with Au to prevent charging and were mounted on Al stages with conductive carbon tape.
XRD. The X-ray diffraction (XRD) pattern was recorded using an X-ray diffractometer (Bruker, Discover D8) with Cu Kα radiation (λ = 1.5418 Å). The measurement parameters were set in the 20°–80° 2θ range, 0.014° sampling frequency, 1 s per step scanning speed, 30 kV voltage, and 40 mA current.
Raman. Raman spectra were collected using a LabRAM ARAMIS (HORIBA Scientific). A 633 nm (1.96 eV) He–Ne laser was used as the light source and optical filters were used to adjust the power of the laser. The area of interest on the sample surface was focused to about 2 mm.
CV. Cyclic voltammetric (CV) measurements were performed using a potentiostat (Gamry Instruments, USA). A platinum plate was used as the counter electrode, and an Ag/AgCl electrode served as the reference electrode in N2− and CO2−saturated solution. Cyclic voltammetric behavior was obtained using a 10 mV s−1 scan rate and a −1.5 to +1.3 V voltage range scan limit.
Acknowledgements
This research was supported by the Office of Naval Research (N0001411103151) and the NSF Engineering Research Center (ERC) at North Carolina A&T State University. NSF funding at the University of Cincinnati through grant SNM-1120382 is gratefully acknowledged.
Notes and references
- T. W. Ebbesen, H. J. Lezec, H. Hiura, J. W. Bennett, H. F. Ghaemi and T. Thio, Nature, 1996, 382, 54–56 CrossRef CAS.
- A. V. V. Ellis, K. Goswami, R. N. Chakrapani, L. S. Ramanathan, P. M. Ajayan and G. Ramanath, Nano Lett., 2003, 3, 279–282 CrossRef CAS.
- A. Peigney, C. Laurent, E. Flahaut, R. R. Bacsa and A. Rousset, Carbon, 2001, 39, 507–514 CrossRef CAS.
- R. S. Ruoff, D. Qian and W. K. Liu, C. R. Phys., 2003, 4, 993–1008 CrossRef CAS PubMed.
- M. r. D. L. Xavier Lepró and R. H. Baughman, Carbon, 2010, 48, 3621–3627 CrossRef PubMed.
- M. B. Jakubinek, M. B. Johnson, M. A. White, C. Jayasinghe, G. Li, W. Cho, M. J. Schulz and V. Shanov, Carbon, 2012, 50, 244–248 CrossRef CAS PubMed.
- W. Li, C. Jayasinghe, V. Shanov and M. Schulz, Materials, 2011, 4, 1519–1527 CrossRef CAS.
- J. H. Pöhls, M. B. Johnson, M. A. White, R. Malik, B. Ruff, C. Jayasinghe, M. J. Schulz and V. Shanov, Carbon, 2012, 50, 4175–4183 CrossRef PubMed.
- H. Chen, A. Roy, J.-B. Baek, L. Zhud, J. Qua and L. Dai, Mater. Sci. Eng., R, 2010, 70, 63–91 CrossRef PubMed.
- S. F. Mei Zhang, A. A. Zakhidov, S. B. Lee, A. E. Aliev, K. R. A. Christopher, D. Williams and R. H. Baughman, Science, 2005, 19, 1215–1219 CrossRef PubMed.
- Y. Jin, H. Chen, M. Chen, N. Liu and Q. Li, ACS Appl. Mater. Interfaces, 2013, 5, 3408–3416 CAS.
- K. Fu, O. Yildiz, H. Bhanushali, Y. Wang, K. Stano, L. Xue, X. Zhang and P. D. Bradford, Adv. Mater., 2013, 25, 5109–5114 CrossRef CAS PubMed.
- J. Wei, Y. Jia, Q. Shu, Z. Gu, K. Wang, D. Zhuang, G. Zhang, Z. Wang, J. Luo, A. Cao and D. Wu, Nano Lett., 2007, 7, 2317–2321 CrossRef CAS PubMed.
- H. Sun, X. You, Z. Yang, J. Deng and H. Peng, J. Mater. Chem. A, 2013, 1, 12422–12425 CAS.
- Z. Yand, T. Chen, R. He, G. Guan, H. Li, L. Qiu and H. Peng, Adv. Mater., 2011, 23, 5436–5439 CrossRef PubMed.
- F. Su, C. Lu, W. Cnen, H. Bai and J. F. Hwang, Sci. Total Environ., 2009, 407, 3017–3023 CrossRef CAS PubMed.
- Y. Hori, K. Kikuchi and S. Suzuki, Chem. Lett., 1985, 14, 1695–1698 CrossRef.
- M. R. Gonçalves, A. Gomes, J. Condeço, R. Fernandes, T. Pardal, C. A. C. Sequeira and J. B. Branco, Energy Convers. Manage., 2010, 51, 30–32 CrossRef PubMed.
- J. Christophe, T. Doneux and C. Buess-Herman, Electrocatal, 2012, 3, 139–146 CrossRef CAS PubMed.
- J. Wu, F. Risalvato and X.-D. Zhoua, ECS Trans., 2012, 41, 49–60 CAS.
- C. W. Li and M. W. Kanan, J. Am. Chem. Soc., 2012, 134, 7231–7234 CrossRef CAS PubMed.
- Y. Hori, Mod. Aspects Electrochem., 2008, 42, 89–189 CAS.
- K. J. P. Schouten, E. Pérez Gallent and M. T. M. Koper, ACS Catal., 2013, 3, 1292–1295 CrossRef CAS.
- J. P. O'Byrne, R. E. Owen, D. R. Minett, S. I. Pascu, P. K. Plucinski, D. Mattia and M. D. Jones, Catal. Sci. Technol., 2013, 5, 1202–1207 Search PubMed.
- C. Genovese, C. Ampelli, S. Perathoner and G. Centi, J. Catal., 2013, 308, 237–249 CrossRef CAS PubMed.
- F. Valentini, A. Amine, S. Orlanducci, M. L. Terranova and G. Palleschi, Anal. Chem., 2003, 75, 5413–5421 CrossRef CAS.
- S. R. Bakshi, D. Lahiri and A. Agarwal, Int. Mater. Rev., 2010, 55, 41–64 CrossRef CAS PubMed.
- V. Shanov, US Pat. PCT/US2011/036190, 2011.
- L. W. C. Jayasinghe, Y. Song, J. L. Abot, V. N. Shanov, S. Fialkova, S. Yarmolenko, S. Sundaramurthy, Y. Chen, W. Cho, S. Chakrabarti, G. Li, Y. Yun and M. J. Schulz, MRS Bull., 2010, 35, 682–692 CrossRef.
- W. Li, C. Jayasinghe, V. N. Shanov and M. J. Schulz, Materials, 2011, 4, 1519–1527 CrossRef CAS.
- G. C. Chen, X. Q. Shan, Y. Q. Zhou, X. E. Shen, H. L. Huang and S. U. Khan, J. Hazard. Mater., 2009, 169, 912–918 CrossRef CAS PubMed.
- C. Bittencourt, X. Ke, G. Van Tendeloo, S. Thiess, W. Drube, J. Ghijsen and C. P. Ewels, Chem. Phys. Lett., 2012, 535, 80–83 CrossRef CAS PubMed.
- M. Park, B.-H. Kim, S. Kim, D.-S. Han, G. Kim and K.-R. Lee, Carbon, 2011, 49, 811–818 CrossRef CAS PubMed.
- A. Felten, J. Ghijsen, J.-J. Pireaux, R. Johnson, C. M. Whelan, D. Liang, G. V. Tendeloo and C. Bittencourt, J. Phys. D: Appl. Phys., 2007, 40, 7379–7382 CrossRef CAS.
- K. T. Kim, S. I. Cha, T. Gemming, J. Eckert and S. H. Hong, Small, 2008, 4, 1936–1940 CrossRef CAS PubMed.
- Y. Yun, V. Shanov, Y. Tu, M. J. Schulz, S. Yarmolenko, S. Neralla, J. Sankar and S. Subramaniam, Nano Lett., 2006, 6, 689–693 CrossRef CAS PubMed.
- S. Wang, X. Huang, Y. He, H. Huang, Y. Wu, X. L. Lizhen Hou, T. Yang, J. Zou and B. Huang, Carbon, 2012, 50, 2119–2125 CrossRef CAS PubMed.
- V. K. Gupta, S. Agarwal and T. A. Saleh, Water Res., 2011, 45, 2207–2212 CrossRef CAS PubMed.
- S. Khabazian and S. Sanjabi, Appl. Surf. Sci., 2011, 257, 5850–5856 CrossRef CAS PubMed.
- C. Suryanarayana, and M. G. Norton, X-Ray Diffraction:
A Practical Approach, Plenum Publishing Corporation, New York, 1998 Search PubMed.
- H. De Jesús-Cardona, C. del Moral and C. R. Cabrera, J. Electroanal. Chem., 2001, 513, 45–51 CrossRef.
- G. Keerthiga, B. Viswanathan, C. Alex Pulikottil and R. Chetty, Bonfring International Journal of Industrial Engineering and Management Science, 2012, 2, 41–43 Search PubMed.
- H. Yano, T. Akiyama, P. Bele, H. Uchida and M. Watanabe, Phys. Chem. Chem. Phys., 2010, 12, 3806–3814 RSC.
- L.-X. W. Huan Wang, Y.-C. Lan, J.-Q. Zhao and J.-X. Lu, Int. J. Electrochem. Sci., 2011, 6, 4218–4227 Search PubMed.
- M. Jayalakshmi and K. Balasubramanian, Int. J. Electrochem. Sci., 2008, 3, 1277–1287 CAS.
- B. D. Smith, D. E. Irish, P. Kedzierzawski and J. Augustynski, J. Electrochem. Soc., 1997, 144, 4288–4296 CrossRef CAS PubMed.
- Y. Hori, A. Murata and R. Takahashi, J. Chem. Soc., Faraday Trans., 1989, 1, 2309–2326 RSC.
- S. K. Shaw, A. Berna, J. M. Feliu, R. J. Nichols, T. Jacobc and D. J. Schiffrin, Phys. Chem. Chem. Phys., 2011, 13, 5242–5251 RSC.
- Y. Hori, H. Wakebe, T. Tsukamoto and O. Koga, Surf. Sci., 1995, 335, 258–263 CrossRef CAS.
- J. J. Kim, D. P. Summers and K. W. Frese Jr, J. Electroanal. Chem. Interfacial Electrochem., 1988, 245, 223–244 CrossRef CAS.
- J. Lee and Y. Tak, Electrochim. Acta, 2001, 46, 3015–3022 CrossRef CAS.
- K. Ogura, H. Yano and F. Shirai, Prepr. Symp. - Am. Chem. Soc., Div. Fuel Chem., 2003, 48, 264–265 CAS.
- W. Li, ACS Symp. Ser., 2010, 5, 55–76 CrossRef PubMed.
- M. Gattrell, N. Gupta and A. Co, J. Electroanal. Chem., 2006, 594, 1–19 CrossRef CAS PubMed.
Footnote |
† Electronic supplementary information (ESI) available. See DOI: 10.1039/c4ra00618f |
|
This journal is © The Royal Society of Chemistry 2014 |