DOI:
10.1039/C4RA00417E
(Paper)
RSC Adv., 2014,
4, 11136-11141
Solvothermal synthesis of FeCo nanoparticles for magneto-controllable biocatalysis†
Received
15th January 2014
, Accepted 11th February 2014
First published on 12th February 2014
Abstract
A facile and simple one-step solvothermal method has been developed to synthesize FeCo nanoparticles with well-controlled composition, desired morphology and high saturation magnetization, which exhibit component-dependent magnetic behaviors: the increase of Fe content leads to the increase of saturation magnetization and the decrease of coercivity. Furthermore, due to the availability of a coarse surface for the stable adsorption of targeted cargo, the high saturation magnetization for efficient control and directional separation from a reaction mixture by an external magnetic field, the as-prepared magnetic FeCo nanoparticles could work as a fuel-free magneto-controllable carrier to load biocatalytically-active cargo lipase for magneto-controllable and recyclable biocatalytic synthesis of lauryl laurate under an external magnetic field. By alternate positioning of the FeCo carrier with loaded cargo in and out of the substrate solution, the biocatalysis for esterification from lauric acid and lauryl alcohol to lauryl laurate could be switched between “On” and “Off” states.
Introduction
Magnetic nanoparticles (MNPs) with well-defined nanoscale structures and unique properties have attracted tremendous research efforts because of the potential applications in many fields,1,2 such as magnetic resonance imaging,3,4 drug delivery,5–7 environmental remediation,8 chemical sensors,9 bioseparation10,11 and biocatalysis.12 Among various magnetic nanomaterials, FeCo nanoparticles (FeCo NPs) exhibit exceptional magnetic properties, including high Curie temperature, the highest saturation magnetization and high permeability.13,14 Several attempts have been made to synthesize FeCo NPs with tunable composition and shape. For example, FeCo NPs with controllable size and composition were obtained by dynamically controlled co-decompositon.15,16 And the interfacial diffusion method has been utilized to prepare FeCo NPs with high magnetic moment.17 Dai et al. have reported the preparation of ultra small FeCo–graphitic carbon shell nanocrystals through adopting chemical vapor deposition, which significantly improved the biocompatibility and stability of the MNPs.18–20 However, to the best of our knowledge, rare research works reported the synthesis of composition- and size-controlled FeCo NPs by solvothermal method, which allows the synthesis of high quality nanostructured materials by solubilizing almost all materials upon heating and pressurizing the solvent system to its critical point.
In addition, effective immobilization of enzymes on surfaces without conformation change and denaturation is crucial for potential applications in the areas of bioelectrochemistry,21 biocatalysis,22–24 bioassays25,26 and bioengineering.27,28 In order to maintain the natural biocatalytic activity and stability, and improve the reusability of the enzymes from the reaction systems, a large number of functional carriers have been employed to immobilize enzymes.29,30 For instance, immobilizing enzymes on MNPs has received considerable attention not only due to the availability of large specific surface areas and stability of the adsorption of targeted cargos, but also because of their efficient control and directional separation from a reaction mixture by an external magnetic field. This makes MNPs as ideal building blocks and suitable support materials for the development of fuel-free directional locomotion of motors/carriers. Enzymes with biocatalytic activity loaded on such magnetically-driven fuel-free nano/microscale carriers can effectively make specific chemical reactions switchable in vivo and in vitro, which presents high catalytic efficiency and selectivity with functional various regulation and control, and meets the modern requirements of developing biosciences and nanomaterials towards facilitating the biocatalysis with high standards of recovery, reusability and catalytic activity under diverse control and regulation.
Herein, we report the synthesis of FeCo NPs with well-controlled composition, desired morphology and high saturation magnetization (Ms) by a facile and simple one-step solvothermal method, which demonstrate component-dependent magnetic behaviors: increase of Fe content leads to the increase of Ms and the decrease of coercivity (Hc) (Scheme 1). Furthermore, the as-prepared magnetic FeCo NPs could be tentatively employed to work as a fuel-free magneto-controllable carrier to load bio-active cargo lipase for magneto-controllable and recyclable biocatalysis of lauryl laurate under external magnetic field because of the coarse surface for the stable adsorption of targeted cargos, the high saturation magnetization for efficient control and directional separation from a reaction mixture under external magnetic field. By alternate positioning of FeCo carrier with loaded cargo in and away from the substrate solution, the biocatalysis for esterification from lauric acid and lauryl alcohol to lauryl laurate could be switched between “On” and “Off” states (Scheme 1B), respectively.
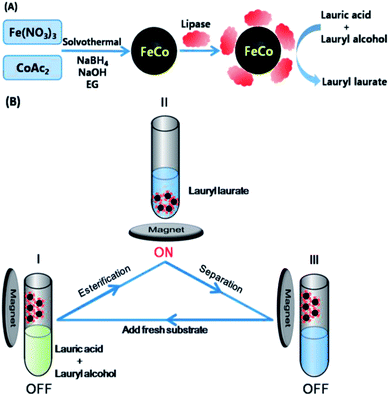 |
| Scheme 1 (A) Synthesis of the magnetic FeCo carrier and loading of biocatalytic-active cargo lipase onto the magnetic carrier for controllable biocatalysis of the esterification from lauric acid and lauryl alcohol to lauryl laurate. (B) Magnetically driven FeCo carrier with loaded lipase for switching biocatalytic synthesis of lauryl laurate between “On” and “Off” states under alternate positioning of the carrier in (from I to II) and out (from II to III) of the substrate solution. Then, after removing the biocatalytically esterificated substrate solution to determine the conversion rate of esterification via titration and adding the same volume of fresh substrate solution (from III to I), the magneto-controllable and recyclable biocatalytic synthesis of lauryl laurate could be restarted. | |
Experimental
Materials
All the reagents used in this work, including ferric nitrate (Fe(NO3)3·9H2O, 99%), cobalt(II) acetate hydrate (Co(Ac)2·4H2O, 99%), sodium hydroxide (NaOH, 99%), sodium borohydride (NaBH4, 99%), ethanediamine (C2H8N2, 99%), ethylene glycol (EG, 99%) were purchased from Beijing Chemical Reagent Factory and were used without further purification. Lipase Candida sp. LS-20 powder (39 kDa, ∼8 nm) with a hydrolytic activity of 10
000 U g−1 was prepared according to our previously published procedures.31
Synthesis of FeCo alloys
A typical synthetic process of FeCo alloy was described as follows.32 0.8 mmol Fe(NO3)3·9H2O and 0.8 mmol Co(Ac)2·4H2O were added into 20 mL EG under ultrasonication at room temperature to form a clear solution, then 0.5 g NaOH was poured into the mixture with magnetic stirring to form an uniform suspension followed by adding 5 mL ethanediamine into it. Next, 1 g of NaBH4 was added into this mixture, which was dissolved by ultrasonication. Then the above solution was transferred to the autoclave at room temperature. The autoclave was sealed and maintained at 180 °C for 24 h. After the autoclave cooled down to room temperature, the FeCo NPs were collected at the bottom of the container. The products were alternately washed by ethanol and distilled water with a permanent magnet for three times, followed by oven-dried at 60 °C for more than 4 h. In addition, the FeCo NPs with different compositions were prepared using the initial molar ratios of Fe3+
:
Co2+ at 0, 3
:
7, 5
:
5, 6
:
4 and 8
:
2, respectively.
Immobilization of lipase on magnetic carrier
The obtained FeCo alloy was used as magnetic carrier for the immobilization of lipase. 10 mL lipase solution with the concentration of 100 mg mL−1 in 0.1 mol L−1 pH 7.0 phosphate buffer solution was mixed with 100 mg MNPs at 25 °C for the physical immobilization of lipase on the rough surface of FeCo carrier. 36 h later, the immobilized lipase was separated from the solution under external magnetic field and washed twice with 0.1 mol L−1 pH 7.0 phosphate buffer solution to remove the free lipase. After that, the immobilized enzymes on magnetic carriers were freeze-dried for the following experiments.
Determination of the loading amount of lipase
The amount of lipase immobilized on the carrier was determined by measuring initial and final concentrations of lipase in the lipase solution using the Bradford protein assay.36
Determination of the esterification conversion rate
The esterification reactions were carried out in a 50 mL round bottom flask, containing lauric acid (0.10 g), lauryl alcohol (0.09 g), hexane (5 mL) and immobilized lipase on magnetic FeCo carrier (20 mg). The reaction temperature was maintained at 40 °C with shaking at 180 rpm for 12 h. As control, the same procedure was conducted with FeCo alloy without loading lipase for the esterification reaction.
The esterification conversion rate was determined by measuring the lauric acid conversion rate upon titration with 0.05 M NaOH according to previously published standard assay conditions.34 First, the FeCo carrier with loaded lipase was collected from the reaction solution after esterification for 12 h under external magnetic field, and the reaction solution was removed and quenched by the addition of 10 mL ethanol for further titration. Then, the FeCo carrier with loaded lipase was washed twice by hexane for the next esterification. To determine the esterification conversion rate, two drops of phenolphthalein and a sufficient amount of 0.05 M NaOH was added to the above reaction solution to give a permanent pink tinge. The consumed volume of NaOH solution was recorded, and the conversion rate of lauric acid was calculated based on eqn (1):
|
A = (V0 − V1)/V0 × 100
| (1) |
where
A is the conversion rate (%) catalyzed by immobilized lipase,
V0 is the consumed volume of NaOH in control experiment (mL), and
V1 is the consumed volume of NaOH in experimental group (mL).
Characterization
The morphology and size of the synthesized samples were determined by using scanning electron microscope measurement (SEM). Specimens were air-dried and imaged using a Zeiss SUPRA 55. The compositions of the samples were measured by energy dispersive spectroscopy (EDS). Powder X-ray diffraction (XRD) data was taken on a D/max-Ultima III (Rigaku) with a Cu Kα X-ray radiation source (λ = 0.15418 nm). The samples were step-scanned in steps of 0.02° (2θ) in the range 5–90° with a speed of 10° per minute. The magnetizations of the FeCo NPs were tested on a JSM-13 vibrating sample magnetometer (VSM) at 298 K and under the applied magnetic field at ±20 kOe.
Results and discussion
Structure characterization
In a typical experiment, FeCo carrier for loading enzymes was prepared through a one-pot solvothermal way using CoAc2 and Fe(NO3)3 as metal precursors, NaBH4 as reductant, C2H8N2 for kinetic control and EG as solvent (Scheme 1). As shown in Fig. 1A, three characteristic peaks consistent with the standard data of FeCo (JCPDS no. 65-4131) were observed for sample b–d. And the characteristic peaks of sample e were consistent with standard data of Co with fcc structure (JCPDS no. 15-0806). Sample a turned out to be a mixture of FeCo and Co, as indicated in Fig. 1A-a. The average diameters of primary nanocrystals estimated from Scherrer equation were 14.53, 15.87, 15.62 and 11.86 nm for sample b–e, showing similar trend with size evolution of the obtained nanoparticles. With the increased content of Fe in the nanocrystals, the spacing of lattice became bigger, leading to the shift of the XRD peaks to small angles, as shown in Fig. 1B.
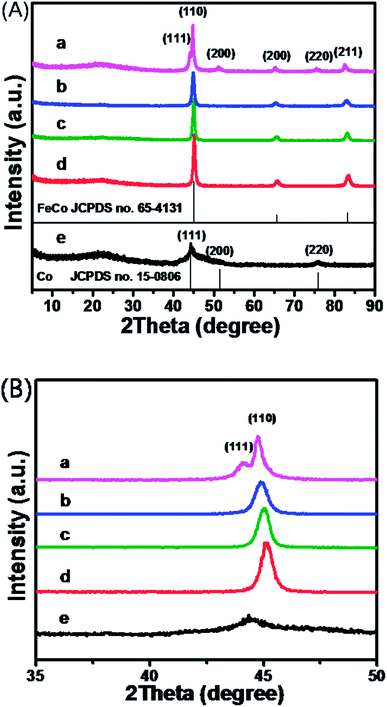 |
| Fig. 1 (A) XRD patterns of the resulted samples with different Fe3+ : Co2+ feeding ratios at (a) 8 : 2, (b) 6 : 4, (c) 5 : 5, (d) 3 : 7, (e) 0, respectively; (B) detailed XRD patterns of (A) from 35° to 50°. | |
Meanwhile, EDS measurements were conducted for the resulted products with different feeding ratios (Fig. S1†). As implied from EDS measurements, sample b–e are Fe50Co50, Fe41Co59, Fe24Co76, and Co, respectively. Compared to the initial feeding ratios, the ratio of Co in the final products was always higher, and the maximum content of Fe in the final products was 50%. And pure alloy could not be obtained when adding large amounts of excessive Fe precursor (Fig. 1A-a). Therefore, Co is easier to be reduced than Fe, which could be further confirmed by the higher electrode potential of Co(OH)2/Co than that of Fe(OH)3/Fe.
On the basis of the Nernst function, the reducibility of NaBH4 could be improved by increasing the concentration of NaOH and the reduction reaction could be carried out easier. With higher basicity, pure Co nanoparticles could be obtained (Fig. S2†), whereas Fe nanoparticles couldn't be prepared (Fig. S3†). In another case, with more excessive Fe precursor than Co precursor added, the resulted product turned out to be the mixture of FeCo and Co (Fig. 1A-a and S4†). Thus, it could be seen that Co was reduced first, after which, the synthesized Fe entered the crystal lattice of Co to form alloy while the majority of Fe precursor couldn't be reduced, confirming that Fe is more difficult to be reduced than Co.
Surface morphology characterization
To characterize the morphology and microstructure of synthesized FeCo alloy, SEM studies were performed. It could be seen that spherical nanoparticles with coarse surfaces were obtained (Fig. 2). As indicated in Fig. 2, spherical FeCo NPs possess heterostructures and secondary structure consisting of tens or hundreds of primary crystals according to the calculation in XRD characterization, making them paramagnetic. The average size of FeCo alloys largely depends on the components. With varied ratios of Fe to Co, different particle sizes could be obtained. The average diameters of Co metal and Fe50Co50 were 600 nm and 500 nm, respectively. But for Fe24Co76 and Fe41Co59, the particle sizes became much bigger, up to 1.5 μm and 1.6 μm, respectively. The differences in sizes may be caused by the nucleation and growth progress of NPs. Co is easier to be reduced, so it will be nucleated first. When the content of Co precursor added became less, the number of nucleuses in solution would be smaller, resulting in bigger particle size. Therefore, the order of average sizes is as follows: Fe41Co59 > Fe24Co76 > Co. However, beyond the corresponding range, the particles became much smaller, such as Fe50Co50, due to the incomplete reduction of Fe. The prepared FeCo NPs with coarse surfaces and paramagnetism may benefit the developing biosciences and nanomaterials towards protein adsorption and bioseparation for facilitating the biocatalysis with high levels of recovery, reusability and catalytic activity under diverse control and regulation.
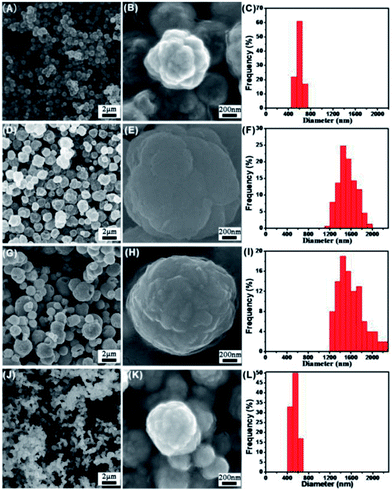 |
| Fig. 2 SEM images of FeCo NPs in different compositions: (A) Co, (D) Fe24Co76, (G) Fe41Co59, (J) Fe50Co50 (low magnification) and (B) Co, (E) Fe24Co76, (H) Fe41Co59, (K) Fe50Co50 (high magnification). Size distributions of (C) Co, (F) Fe24Co76, (I) Fe41Co59, (L) Fe50Co50 after counting more than 200 particles for each sample. | |
Magnetic properties
The magnetic properties of the obtained samples were investigated by VSM at room temperature. Fig. 3 reveals the magnetization curves of different kinds of MNPs measured at room temperature, in which steeply increased magnetization with increasing field could be observed. It could be seen that FeCo NPs possess much higher saturation magnetization than the Co metal or Fe3O4. Furthermore, magnetic properties of the FeCo NPs depend on the ratio of Fe and Co atoms. As summarized in Table S1† for detailed saturation magnetization and coercivity of FeCo NPs with different compositions, an increase in saturation magnetization was observed with increasing Fe content among FeCo alloys with different Fe3+
:
Co2+ ratios, which may be obviously due to relatively higher contribution of high magnetic moment metallic Fe compared to that of low magnetic moment metallic Co. And the maximum magnetization 186.83 emu g−1 was achieved for Fe41Co59, which was lower than that of bulk FeCo alloy (245 emu g−1), probably because of the polycrystalline nature of these NPs and NP surface oxidation.17 When Fe content further increased, maximum magnetization decreased, such as 180.48 emu g−1 for Fe50Co50. Hysteresis measurements display that Fe41Co59 and Fe50Co50 possess excellent soft-magnetic properties at room temperature with smaller coercivity around 70 Oe. However, with higher Co content, coercivity becomes much bigger for Fe24Co76 at 231.08 Oe, and this may be ascribed to higher anisotropy energy of Co.33
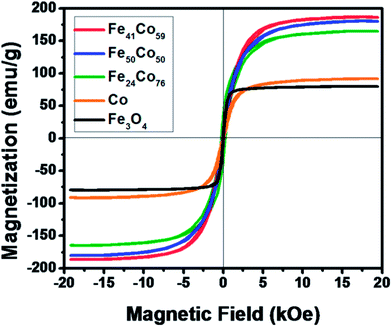 |
| Fig. 3 Magnetic behavior of FeCo NPs with different compositions measured at 298 K. | |
Magneto-controllable and recyclable biocatalysis
The as-prepared magnetic Fe41Co59 NPs with highest Ms were chosen to work as a fuel-free magneto-controllable carrier to load bio-active cargo lipase to its coarse surface through physical adsorption35,36 for magneto-controllable and recyclable biocatalytic synthesis of lauryl laurate under external magnetic field. Compared with Fe41Co59 before lipase immobilization, no obvious increase in size but a smoother surface of Fe41Co59 with loaded lipase was observed in Fig. S5,† maybe due to filling the interspaces on the coarse surface with lipase. And the loading amount of lipase was determined to be 0.04 g g−1, similar to our previous report.29 The activation and deactivation of biocatalytic cargo's catalytic activity by magnetically driven positioning of the magnetic FeCo carrier with loaded lipase near and away from the substrate solution can be employed for magneto-switchable biocatalysis of lauric acid and lauryl alcohol to lauryl laurate between active and inactive biocatalytic states (Scheme 1B), respectively. After the dispersion in solution, the carrier could be collected completely and quickly with an outer magnet (Fig. S6†). Directionally positioning the magneto-responsive FeCo carrier with loaded lipase into the substrate solution by external magnetic field, enables the biocatalytic synthesis of lauryl laurate (from Scheme 1B-I to II). After the biocatalytic synthesis of lauryl laurate was carried out at 40 °C for 12 h, magnetically-driven position of FeCo carrier with loaded lipase away from substrate solution blocks the biocatalytic reaction due to the lack of lipase (from Scheme 1B-II to III). Then, the substrate solution was removed for determining the conversion rate of esterification by titration, confirming that the biocatalytic synthesis of lauryl laurate was efficiently catalyzed by the loaded lipase. Adding the same volume of fresh substrate solution (from Scheme 1B-III to I), the magneto-controllable and recyclable biocatalytic synthesis of lauryl laurate can be restarted. By alternate positioning of FeCo carrier with loaded cargo in and out of the substrate solution, the biocatalysis of lauric acid and lauryl alcohol to lauryl laurate could be switched between “On” and “Off” states (Scheme 1B), respectively. As demonstrated in Fig. 4, the immobilized lipase possesses the good performance for esterification with conversion rates in the first four cycles around 85%. From the fifth cycle, the conversion rate dropped slightly maybe due to the desorption of lipase from the surface of FeCo carrier. As reported previously by others that the esterification conversion rate for lipase immobilized on amino-functionalized mesoporous silica in the first cycle was lower than 70% and it decreased quickly from the second cycle.34 Lipase immobilized on nonwoven fabric membranes for the esterification of oleic acid and ethanol has been studied with a conversion rate lower than 80%.30 And the synthesis of glycerol carbonate could also be catalyzed by cross-linked lipase aggregates onto magnetic particles with a yield of around 60%.35 Therefore, our as-prepared FeCo carrier could be a great candidate for the immobilization of lipase and the subsequent biocatalysis.
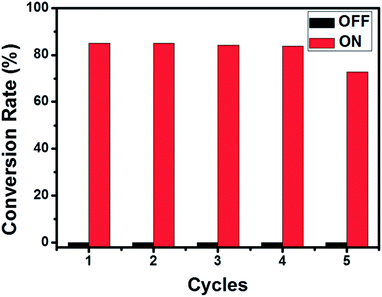 |
| Fig. 4 Magneto-controllable and recyclable conversion rate of esterification (T = 40 °C). | |
Conclusions
In conclusion, we have developed a facile and simple one-step solvothermal method to synthesize FeCo NPs with well-controlled composition, desired morphology, high saturation magentization, and exceptionally component-dependent magnetic behavior. Because of the coarse surface for adsorption of targeted cargos and the high saturation magnetization for efficient control and directional separation from a reaction mixture by an external magnetic field, the as-prepared FeCo carrier could be employed to work as a fuel-free magneto-controllable carrier to effectively load bio-active cargo lipase for magneto-controllable and recyclable biocatalytic synthesis of lauryl laurate. By alternate positioning of FeCo carrier with loaded cargo in and out from the substrate solution, the biocatalysis for esterification from lauric acid and lauryl alcohol to lauryl laurate could be switched between “On” and “Off” states, respectively. It is anticipated to provide an excellent platform for fabricating functional biocarriers with potentially wide-ranging applications in fuel-free magneto-bioelectronics and bioisolation, magneto-switchable fuel cells and bioreactors, controlled biomedical drug delivery and information storage, controllable cell enrichment and detection.
Acknowledgements
This work was supported by NSFC, the National Basic Research Program of China (973 program) (2013CB733600, 2012CB725200, 2011CBA00503) and the Fundamental Research Funds for the Central Universities.
Notes and references
- H. Deng, X. L. Li, Q. Peng, X. Wang, J. P. Chen and Y. D. Li, Angew. Chem., 2005, 117, 2842–2845 CrossRef.
- X. Wang, J. Zhuang, Q. Peng and Y. D. Li, Nature, 2005, 437, 121–124 CrossRef CAS PubMed.
- K. Yang, L. Hu, X. Ma, S. Ye, L. Cheng, X. Shi, C. Li, Y. Li and Z. Liu, Adv. Mater., 2012, 24, 1868–1872 CrossRef CAS PubMed.
- L. Gao, L. Xie, X. Long, Z. Wang, C. Y. He, Z. Y. Chen and B. Qiu, Biomaterials, 2013, 34, 3688–3696 CrossRef CAS PubMed.
- S. Huang, C. Li, Z. Cheng, Y. Fan, P. Yang, C. Zhang, K. Yang and J. Lin, J. Colloid Interface Sci., 2012, 376, 312–321 CrossRef CAS PubMed.
- N. Wang, Y. Guan, L. Yang, L. Jia, X. Wei, H. Liu and C. Guo, J. Colloid Interface Sci., 2013, 395, 50–57 CrossRef CAS PubMed.
- C. H. Fan, C. Y. Ting, H. J. Lin, C. H. Wang, H. L. Liu, T. C. Yen and C. K. Yeh, Biomaterials, 2013, 34, 3706–3715 CrossRef CAS PubMed.
- B. I. Kharisov, H. R. Dias, O. V. Kharissova, V. M. Jiménez-Pérez, B. O. Pérez and B. M. Flores, RSC Adv., 2012, 2(25), 9325–9358 RSC.
- P. B. Wan, S. Y. Yin, L. L. Liu, Y. G. Li, Y. J. Liu, X. T. Wang, W. Leow, B. Ma and X. D. Chen, Small, 2013 DOI:10.1002/smll.201302014.
- F. X. Qie, G. X. Zhang, J. X. Hou, X. M. Sun, S. Z. Luo and T. W. Tan, Talanta, 2012, 93, 166–171 CrossRef CAS PubMed.
- G. X. Zhang, F. X. Qie, J. X. Hou, S. Z. Luo, L. Luo and X. M. Sun, J. Mater. Res., 2012, 27, 1006–1013 CrossRef CAS.
- O. Gutfleisch, M. A. Willard, E. Brück, C. H. Chen, S. G. Sankar and J. P. Liu, Adv. Mater., 2011, 23, 821–842 CrossRef CAS PubMed.
- X. Sun, S. M. Tabakman, W. S. Seo, L. Zhang, G. Zhang, S. Sherlock, L. Bai and H. Dai, Angew. Chem., Int. Ed., 2009, 48, 939–942 CrossRef CAS PubMed.
- T. Sourmail, Prog. Mater. Sci., 2005, 50, 816–880 CrossRef CAS PubMed.
- C. Desvaux, C. Amiens, P. Fejes, P. Renaud, M. Respaud, P. Lecante, E. Snoeck and B. Chaudret, Nat. Mater., 2005, 4, 750–753 CrossRef CAS PubMed.
- G. S. Chaubey, C. Barcena, N. Poudyal, C. B. Rong, J. Gao, S. H. Sun and J. P. Liu, J. Am. Chem. Soc., 2007, 129, 7214–7215 CrossRef CAS PubMed.
- C. Wang, S. Peng, L. M. Lacroix and S. H. Sun, Nano Res., 2009, 2, 380–385 CrossRef CAS.
- Z. Chen, G. Hong, H. Wang, K. Welsher, S. M. Tabakman, S. P. Sherlock, J. T. Robinson, Y. Liang and H. Dai, ACS Nano, 2012, 6, 1094–1101 CrossRef CAS PubMed.
- W. S. Seo, J. H. Lee, X. Sun, Y. Suzuki, D. Mann, Z. Liu, M. Terashima, P. C. Yang, M. V. McConnell, D. G. Nishimura and H. Dai, Nat. Mater., 2006, 5, 971–976 CrossRef CAS PubMed.
- S. P. Sherlock and H. J. Dai, Nano Res., 2011, 4, 1248–1260 CrossRef CAS.
- E. Katz and I. Willner, J. Am. Chem. Soc., 2003, 125, 6803–6813 CrossRef CAS PubMed.
- D. F. Izquierdo, J. M. Bernal, M. I. Burguete, E. Garcia-Verdugo, P. Lozano and S. V. Luis, RSC Adv., 2013, 3, 13123–13126 RSC.
- Z. Y. Zhao, J. Liu, M. Hahn, S. Qiao, A. P. Middelberg and L. He, RSC Adv., 2013, 3, 22008–22013 RSC.
- D. Moelans, P. Cool, J. Baeyens and E. F. Vansant, Catal. Commun., 2005, 6, 307–311 CrossRef CAS PubMed.
- H. C. Wu and H. Bayley, J. Am. Chem. Soc., 2008, 130, 6813–6819 CrossRef CAS PubMed.
- R. Dronov, D. G. Kurth, H. Möhwald, R. Spricigo, S. Leimkühler, U. Wollenberger, K. V. Rajagopalan, F. W. Scheller and F. Lisdat, J. Am. Chem. Soc., 2008, 130, 1122–1123 CrossRef CAS PubMed.
- T. Sun, D. Han, K. Rhemann, L. Chi and H. Fuchs, J. Am. Chem. Soc., 2007, 129, 1496–1497 CrossRef CAS PubMed.
- P. B. Wan, Y. Y. Chen, Y. B. Xing, L. F. Chi and X. Zhang, Langmuir, 2010, 26, 12515–12517 CrossRef CAS PubMed.
- C. X. Cui, Y. F. Tao, L. L. Li, B. Q. Chen and T. W. Tan, J. Mol. Catal. B: Enzym., 2013, 91, 59–66 CrossRef CAS PubMed.
- W. N. Li, B. Q. Chen and T. W. Tan, Process Biochem., 2011, 46, 1358–1365 CrossRef CAS PubMed.
- T. W. Tan, M. Zhang, B. W. Wang, C. H. Ying and L. Deng, Process Biochem., 2003, 39, 459–465 CrossRef CAS.
- M. Z. Cheng, M. Wen, S. Q. Zhou, Q. S. Wu and B. Sun, Inorg. Chem., 2012, 51, 1495–1500 CrossRef CAS PubMed.
- M. Abbas, M. N. Islam, B. Parvatheeswara Rao, T. Ogawa, M. Takahashi and C. G. Kim, Mater. Lett., 2013, 91, 326–329 CrossRef CAS PubMed.
- C. F. Wang, G. W. Zhou, Y. J. Li, N. Lu, H. B. Song and L. Zhang, Colloids Surf., A, 2012, 406, 75–83 CrossRef CAS PubMed.
- M. Tudorache, A. Nae, S. Coman and V. I. Parvulescu, RSC Adv., 2013, 3, 4052–4058 RSC.
- M. M. Bradford, Anal. Biochem., 1976, 72, 248–254 CrossRef CAS.
Footnote |
† Electronic supplementary information (ESI) available: EDS results of the as-obtained samples and XRD characterization under different conditions. See DOI: 10.1039/c4ra00417e |
|
This journal is © The Royal Society of Chemistry 2014 |