DOI:
10.1039/C3RA47530A
(Paper)
RSC Adv., 2014,
4, 8764-8771
Reversible response of poly(aspartic acid) hydrogels to external redox and pH stimuli†
Received
11th December 2013
, Accepted 15th January 2014
First published on 16th January 2014
Abstract
A redox- and pH-responsive poly(aspartic acid) (PASP) hydrogel is synthesised by cross-linking cysteamine modified polysuccinimide (PSI) with non-cleavable cross-linker 1,4-diaminobutane followed by the hydrolysis of the resultant PSI gel to a PASP hydrogel. The hydrogel showed a remarkable change in its degree of swelling as a function of external pH because of the polyelectrolyte character of the network. In addition to pH-dependent swelling, the thiolation of the polymer rendered redox responsive behaviour to poly(aspartic acid). The degree of swelling could be tuned in a reversible manner by immersing the hydrogel in reducing or oxidising media. The elastic modulus of the hydrogel was also strongly affected by the presence of reducing or oxidising agents. Moreover, the gel strength could be improved via oxidation. The reversible response of the degree of swelling and the elastic modulus was induced through the thiol–disulphide transformation inside the hydrogel proven using a new analytical method. Consequently, a dual responsive hydrogel is obtained with redox-tunable cross-link density.
Introduction
Responsive hydrogels have attracted particular attention over the past few years due to their potential application in regenerative medicine and targeted delivery of therapeutic agents.1–6 These hydrogels can undergo abrupt changes when exposed to physical changes (temperature,7–9 light,10–12 electric13 or magnetic fields14) or chemical stimuli (ionic strength, pH15–17 or enzymatic conditions18). The responses can be very diverse, such as degradation, sol–gel transition or swelling or shrinking of the hydrogel.19
Temperature and pH are the most appealing stimuli for the development of hydrogels, either for injectable systems with sol–gel transition20 or macro/nanogels with swelling-shrinking responses to environmental triggers.19 These materials are often utilised in the targeted delivery of drugs, proteins or genes with a degradation- or diffusion-controlled release.19 The delivery of drugs through the complete degradation of the vehicle is not always satisfactory when a more complex release profile is needed, e.g., in the case of diabetic disorders. A typical solution to this challenge is on-demand, diffusion-controlled release of insulin performed using a pH-sensitive delivery matrix with response to the change in pH triggered by the glucose oxidase enzyme entrapped in the hydrogel.21,22 The reversible swelling and shrinking of the hydrogel allow for a long-term insulin release which is controlled by the current health state of the patient.
Specificity of the response can be improved through the design of multiresponsive materials.1 Injectable hydrogels with a sol–gel transition triggered by both pH and temperature are widely known representatives of this class. These hydrogels have the advantage that, contrary to temperature responsive materials, premature gelation can be avoided.23
In addition to pH and temperature, redox potential has earned the greatest interest among external triggers and a number of redox-responsive vehicles have been designed recently.24 These delivery systems are intended to degrade or disassemble in the cytosol where glutathione is present in a concentration of ca. 0.5–10 mM, contrary to the small glutathione concentration (2–20 μm) in the extracellular matrix.25,26 The presence of this redox gradient as an internal stimulus of the mammalian cells inspired the design of several bioreducible drug and gene delivery systems.24,27 However, most of the reported gels exhibited only a one-way response and disintegrated upon reduction. Redox-responsive vehicles with a reversible macroscopic change in the degree of swelling via a redox reaction would have a large advantage over hydrogels exhibiting reduction induced disintegration: a modulated release that is controlled by the pathological state of the patient can be achieved.
Hydrogel matrices with a reversible response to external redox stimulus were reported only in a few papers. A large variety of chemical moieties, e.g., ferrocenyl,28 nitroxyl, phenoxyl, carbazol, quinones, viologens or hydrazyl groups29 were utilised to synthesise redox-responsive polymer networks; however, their application in biomedical fields is rather limited because of their composition. Yoshida et al.30 synthesised a poly(N-isopropylacrylamide) gel with Ru-containing side-chain, which was coupled with an oscillatory reaction inside the gel. The hydrogel was capable of converting chemical energy into mechanical work; thus, it is a feasible pre-cursor material for artificial muscles and actuators. Allcock et al.31 developed phosphor containing polymer networks cross-linked with multivalent metal cations. A remarkable and reversible bending response to external electrochemical stimuli was obtained. However, biomedical application of the above-mentioned hydrogels is strongly limited. The rational design of redox-responsive hydrogels that are applicable in biological environments demands the incorporation of thiol groups or disulphide linkages into the polymer network.32
Poly(aspartic acid) (PASP) is biodegradable and biocompatible and already has several uses as a superhydrophilic and pH-responsive hydrogel.33,34 The precursor polymer of PASP, polysuccinimide (PSI), can be readily modified.35,36 Redox responsive features can be incorporated into the polymer network via the thiolation of the polymer, resulting in a dual responsive hydrogel matrix.37 The preparation of cross-linked PASP hydrogels and particles with one-way reduction sensitive response was reported in the literature,38–40 but a PASP hydrogel with reversible redox-induced swelling and shrinking has not been introduced until now. Consequently, our main goal was to prepare a redox and pH responsive hydrogel of thiolated PASP in which the cross-linking density, and thus the degree of swelling, is regulated in a reversible manner by changing the external redox potential.
Experimental section
Materials
1,4-Diaminobutane (DAB, 99%), imidazole (Puriss p.a.) and Ellman's reagent [5,5′-dithiobis(2-nitrobenzoic acid)] (98%) were obtained from Sigma-Aldrich. S-Aspartic acid (99%), dibutylamine (DBA, 99%), methanol (MeOH, 99.9%), cysteamine hydrochloride (97%), potassium chloride (99.5%), sodium tetraborate decahydrate (a. r.), sodium bromate (99%), dithiothreitol (DTT, for biochem.), mesitylene (for synthesis) and sulfolane (for synthesis) were acquired from Merck. Phosphoric acid (cc. 85%), hydrochloric acid (HCl, 35%) and dimethylformamide (DMF, pure) were purchased from Lach Ner. Citric acid monohydrate (99%) and sodium hydroxide (NaOH, a. r.) were purchased from Reanal (Hungary). All reagents and solvents were used without further purification. The synthesis of the modified and cross-linked polymers and all of the measurements were carried out at 25 °C unless otherwise indicated.
Synthesis of polysuccinimide
Polysuccinimide (PSI) was prepared through acid-catalysed thermal polycondensation of aspartic acid in a mixture of mesitylene and sulfolane at 160 °C (7 h) according the reaction shown in Scheme 1.37
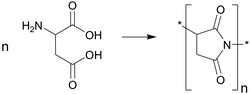 |
| Scheme 1 Polycondensation reaction of aspartic acid resulting in polysuccinimide (PSI). | |
PSI was purified by precipitation in methanol and dried in vacuum at 25 °C. Its chemical structure was confirmed by 1H NMR (300 MHz, DMSO-d6, δ): 5.10 (d, 1H, CH); 3.20 and 2.75 (ss, 2H, CH2). PSI was hydrolysed to PASP in mildly alkaline solution (pH = 8) and the average molecular weight of the resultant PASP was determined with HPLC size exclusion chromatography (SEC). A Nucleogel GFC-300 column was used (molecular weight range of 1–100 kDa) with PBS eluent. The average molecular weight (Mw) of PASP was 56.1 kDa with polydispersity index (PDI) = 1.07.
Synthesis of cysteamine-modified polysuccinimides
PSI was modified with cysteamine side chains to incorporate redox sensitive moieties into the polymer.37 The feed compositions of cysteamine modified polymers are listed in Table 1. Cysteamine hydrochloride and PSI was dissolved in DMF at room temperature. Dibutylamine was added to the mixture drop wise to deprotonate the amine groups of cysteamine hydrochloride. The mixture was stirred at ambient temperature for 6 h to synthesise cysteamine modified polymers (Scheme 2(I)). A nitrogen atmosphere was applied during the synthesis of thiolated polymers to hinder the oxidation of thiol side chains by air.
Table 1 Composition (polymer concentration, molar ratio of thiol side chains and permanent cross-linkers to repeating units) and gelation time of 1,4-diaminobutane cross-linked thiolated polysuccinimide gels; degree of swelling of poly(aspartic acid) hydrogels after hydrolysis at pH = 8
Sample |
Polysuccinimide |
Poly(aspartic acid) |
cPSIa (wt%) |
XCYSb (%) |
XDABc (%) |
tgeld (min) |
Qme (—) |
cPSI: feed wt% of polysuccinimide in DMF. XCYS: feed ratio of cysteamine hydrochloride to succinimide repeating units during preparation. XDAB: feed ratio of 1,4-diaminobutane to succinimide repeating units during preparation. tgel: gelation time of thiolated polysuccinimide after adding 1,4-diaminobutane as a permanent cross-linker in DMF. Qm: the degree of swelling of poly(aspartic acid) hydrogels in aqueous solution at pH = 8; *not measured because of the dissolution of the hydrogels at pH = 8. |
Gel-A |
6.80 |
20 |
5 |
100 |
—* |
Gel-B |
6.80 |
20 |
10 |
40 |
4.7 ± 0.3 |
Gel-C |
6.80 |
40 |
5 |
180 |
—* |
Gel-D |
6.80 |
40 |
10 |
100 |
—* |
Gel-E |
9.70 |
20 |
5 |
80 |
—* |
Gel-F |
9.70 |
20 |
10 |
65 |
8.5 ± 0.2 |
Gel-G |
9.70 |
40 |
5 |
170 |
—* |
Gel-H |
9.70 |
40 |
10 |
100 |
—* |
Gel-I |
13.60 |
20 |
5 |
60 |
9.8 ± 0.4 |
Gel-J |
13.60 |
20 |
10 |
40 |
9.1 ± 1.5 |
Gel-K |
13.60 |
40 |
5 |
80 |
10.3 ± 0.1 |
Gel-L |
13.60 |
40 |
10 |
75 |
8.9 ± 0.5 |
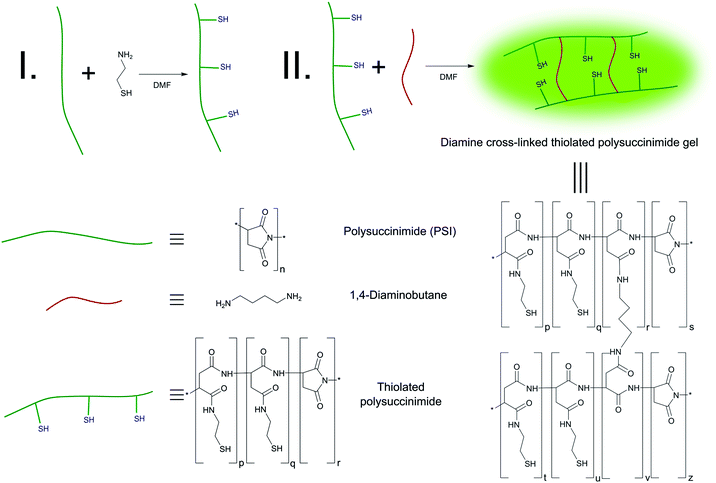 |
| Scheme 2 Synthesis of thiolated polysuccinimide (I) and 1,4-diaminobutane cross-linked thiolated polysuccinimide gels (II). | |
Synthesis of 1,4-diaminobutane cross-linked, cysteamine-modified polysuccinimide gels
Cysteamine-modified polymers could be cross-linked with 1,4-diaminobutane in the same reaction mixture without extracting thiolated PSI in solid form to simplify the synthesis because the presence of dibutylamine and unreacted cysteamine does not interfere with the cross-linking reaction (Scheme 2(II)). Polymer gels were moulded into sheets (10 × 10 × 0.2 cm) between two glass plates separated with a silicone spacer.
Synthesis of 1,4-diaminobutane cross-linked, cysteamine-modified poly(aspartic acid) gels
1,4-Diaminobutane cross-linked, cysteamine-modified polysuccinimide gels were swollen in aqueous buffer solution of pH = 8. The swelling medium was replaced several times to remove DMF and residues of unreacted cross-linkers, and water-swellable PASP gels were obtained as a result (Scheme 3).
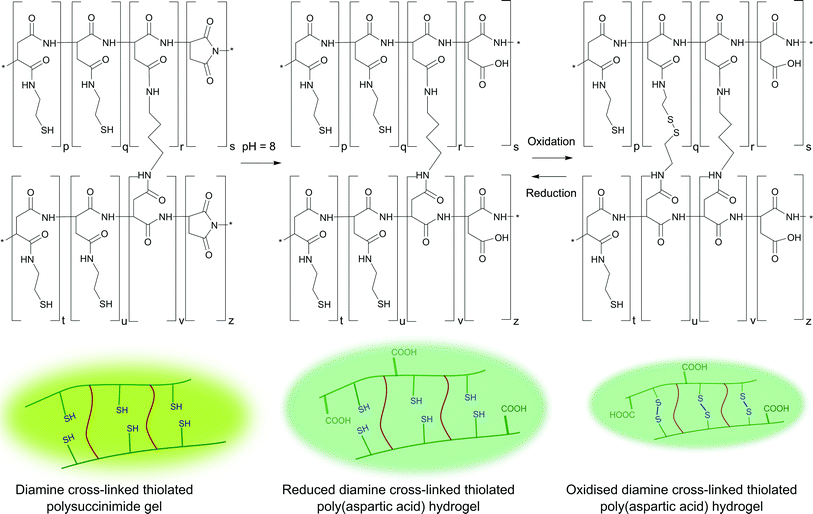 |
| Scheme 3 Synthesis of 1,4-diaminobutane cross-linked, cysteamine-modified poly(aspartic acid) gels and thiol–disulphide transformation in poly(aspartic acid) hydrogels. | |
Measurement of degree of swelling
Swelling experiments were performed in different media to demonstrate the reversible dual-response of the hydrogels. Hydrogel discs with a diameter of 10 mm and thickness of 2 mm were used in each case. The degree of swelling (Qm) was defined as the weight ratio of the swollen (ms) to the dried polymer gel (md):
The weight of the swollen polymer gel (ms) was measured after removing the excess swelling solution from the surface of the gel. The weight of the dried polymer gel (md) was measured after removing the buffer components by washing the gels with deionised water several times and drying them for two days in vacuum at ambient temperature.
The pH-dependent degree of swelling was measured by immersing the gels into aqueous buffer solutions of citric acid (from pH = 2 to pH = 6), imidazole (from pH = 6 to pH = 8) and sodium tetraborate (from pH = 8 to pH = 12). The pH values were adjusted by adding 1 M HCl or 1 M NaOH. The pH of the buffer solutions was checked with a pH/ion analyser (Radelkis OP-271/1, Hungary).
The redox-sensitive degree of swelling was measured at constant pH (pH = 8) in the presence of the reducing agent dithiothreitol (DTT, c = 10 mM) or the oxidising agent sodium bromate (c = 10 mM).
The ionic strength of buffer solutions was adjusted to different values by adding proper amounts of KCl (0.10, 0.15, 0.20 or 0.25 M).
Determination of the elastic modulus
The elastic modulus of the 1,4-diaminobutane cross-linked thiolated PASP hydrogels was determined by uniaxial stress–strain measurements using cylindrical samples (diameter ≈ height ≈ 1.5 cm) (Instron 5543 mechanical tester, USA). The compressive modulus (G) was determined using the elasticity model of regular networks:
where σn represents the nominal stress and λ is the relative deformation.
Ellman's assay of poly(aspartic acid) hydrogels
Ellman's assay was used for the determination of the thiol content of PASP hydrogels cross-linked with 1,4-diaminobutane. The hydrogels were carefully washed with the buffer solution used in the Ellman's assay before each measurement to remove any traces of oxidising or reducing reagents.† Hydrogel pieces with masses of approximately 100 mg were put into the solution of Ellman's reagent (V = 5 mL, creagent = 0.5 mM, pH = 8) after the careful removal of oxygen by bubbling nitrogen through the solution. The reaction was performed for 6 h with the gentle stirring of the solution. The hydrogel pieces were removed and washed with buffer solutions to remove the reagent. The solutions were collected and the thiolated form of Ellman's reagent was measured with an Analytic Jena Specord 200 spectrophotometer (Germany).†37
Results and discussion
Characterisation of 1,4-diaminobutane cross-linked, cysteamine-modified polysuccinimide and poly(aspartic acid) gels
Cysteamine modified polysuccinimide (PSI) and its corresponding poly(aspartic acid) derivative (PASP) exhibited a reversible sol–gel transition induced by atmospheric or chemical oxidation and reduction by dithiothreitol as reported in our previous paper.37 The cross-linking of the thiolated PSI with 1,4-diaminobutane as permanent cross-linker resulted in homogeneous transparent gels independently of the feed composition of the polymers. The gelation time increased with increasing thiol content (Table 1), which is explained by the presence of hydrogen bonds between the thiol side chains, resulting in loose physical cross-links that hinder the formation of chemical cross-links. Increasing the polymer concentration and increasing the molar ratio of the permanent cross-linker to the repeating units accelerated the gelation process. The effect of the cross-linker concentration is more pronounced at lower polymer concentrations. It is worth mentioning that gelation occurred within a reasonable gelation time over a wide range of network compositions (Table 1). The effect of the polymer concentration, the molar ratio of cysteamine to repeating units and the molar ratio of 1,4-diaminobutane to repeating units on properties of PASP hydrogels was studied and reported in the subsequent paragraphs.
Cysteamine modified polysuccinimide gels cross-linked with 1,4-diaminobutane were hydrolysed in alkaline aqueous solution (pH = 8). The equilibrium degree of swelling of the resultant PASP hydrogels (Qm) was determined as a function of feed composition (Table 1). The correlation between the composition of the hydrogels and their equilibrium degree of swelling is rather complex. Gels with lower polymer concentrations (6.80 or 9.70 wt%) disintegrated in alkaline solution, except Gel-B and Gel-F, which were densely cross-linked with 1,4-diaminobutane. Their thiolation degree was 20%. Gels with the highest polymer concentration (13.60%) were stable in alkaline medium independent of their thiolation degree and the concentration of the permanent cross-linker. However, the latter gels exhibited considerably larger degrees of swelling in aqueous solution than Gel-B and Gel-F. The observed swelling behaviour could be explained by the steric hindrance of the side chains of the aspartamide repeating units as well as by the presence of chemical cross-links and second-order interactions (hydrogen bonds and chain entanglements). We assume that the high polymer concentration during the synthesis resulted in considerable entanglement of the polymer chains, resulting in a large net point density in DMF. Permanent cross-links prevent the disentanglement of chains; thus, the total number of physical and chemical net points is large at high polymer concentrations, and the gels do not dissolve. In addition to chain entanglement and chemical cross-links, hydrogen bonds formed between thiol groups must also be taken into account. The concentration of hydrogen bonds decrease in aqueous solution (pH = 8) because thiol groups are partially deprotonated and solvated by water. This latter effect might be the reason for the dissolution of hydrogels with low polymer concentration, i.e., the limited number of chain entanglement and the presence of chemical net points is not sufficient to prevent the dissolution of the hydrogels. Increasing the polymer concentration results in higher thiol concentrations further hindering the cross-linking reaction with 1,4-diaminobutane because of the limited reactivity of the physically cross-linked network. The decreased reactivity results in a lower concentration of chemical net points in the PASP gels and an increase in the degree of swelling with increasing polymer concentration in aqueous media. The hydrogels with the highest initial polymer concentration (13.6 wt%) were chosen for further experiments to study the effect of the thiolation degree and the molar ratio of 1,4-diaminobutane to repeating units on redox response.
Redox response of the degree of swelling of poly(aspartic acid) gels
The thiol–disulphide transformation is hindered at pH values below 6 because of the protonation of the thiol groups (the pKa value of cysteamine is approximately 8.35). Disulphide formation is preferred only in the presence of the thiolate anion; thus, it became favoured above pH = 6.41 Accordingly, the redox response of the hydrogels was studied at pH = 8. The hydrogels showed environmental response in their degree of swelling when immersed in aqueous oxidising (10 mM NaBrO3) and then reducing (10 mM DTT) solutions (Fig. 1). The degree of swelling decreased in oxidising medium due to the formation of disulphide linkages from thiol groups, while it increased in reducing medium because of the cleavage of the disulphide linkages. The change in degree of swelling was more significant in the case of a smaller number of permanent cross-linkers; thus, the magnitude of the redox response could be varied with composition.
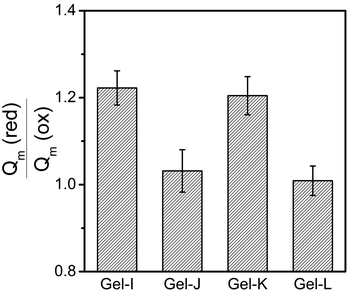 |
| Fig. 1 Ratio of the degree of swelling of PASP gels in reducing medium (10 mM DTT) to oxidising medium (10 mM NaBrO3) for different compositions of the polymer network. | |
The reversibility of the redox-response is of great interest for potential applications, and it is demonstrated in the case of Gel-K. Several redox-induced swelling-shrinking cycles could be performed in a reversible manner as shown in Fig. 2a. The swelling response could be adjusted by varying the ionic strength of the solution which extends even further the application possibilities of our redox-responsive hydrogel. As shown in Fig. 2b, the equilibrium degree of swelling of the hydrogel could be tuned between 24 and 40 in reducing medium and between 17 and 34 in oxidising medium by changing the ionic strength of the solution. Ionic strength was varied between 0.10 and 0.20 M during these experiments. The magnitude of the response, which is defined as the ratio of degree of swelling in reducing and oxidising media, is compared in Fig. 2c. The redox-response is expressed more at lower ionic strengths, and the response is the weakest at the largest ionic strength. The phenomenon may be explained by the polyelectrolyte character of the polymer network. The dissociation of carboxyl groups in repeating units is impeded at large salt concentrations, leading to decreased swelling; thus, a stronger response needs a lower concentration of ions. The ionic strength varies over a wide range at physiological sites (between ca. 40 to 120 mM); thus, the importance of the ionic strength dependent response is clear, and the extent of swelling needs to be tuned by adjusting the composition according to the ionic strength of the site of the future application.
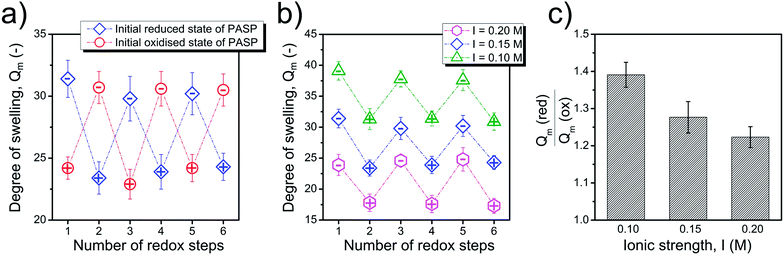 |
| Fig. 2 Reversible changes in the degree of swelling of PASP gels (Gel-K) in reducing and oxidising media (a) with reduction and oxidation as the initial redox step, respectively, at constant ionic strength (0.15 M); (b) at different ionic strengths. The oxidised state is denoted by + signs, reduced state by − signs. (c) The magnitude of the redox response at different ionic strengths. | |
pH-dependent degree of swelling of poly(aspartic acid) gels
PASP hydrogels offer a reversible pH-responsive behaviour as reported earlier.17,35,37 pH-sensitivity is explained by the polyelectrolyte character of the main polymer chain. A large number of carboxyl groups are present on the polymer chain even after modification and/or cross-linking. The environmental pH determines the ionisation degree of these dissociative groups, and the presence of ionised groups affects the equilibrium degree of swelling of the hydrogels. The degree of swelling of thiol-grafted, diamine cross-linked hydrogels was measured over a wide pH range (pH = 2 to 9) to determine whether the pH-sensitive character was preserved in the polymer network after modification. A significant response was observed as a function of pH (Fig. 3). The correlations of both the reduced and oxidised gels showed that the degree of swelling is as small as approximately 3 at pH < 3. The limited swelling can be explained by the fact that the limited solubility of the polymer determines the degree of swelling in acidic medium, and the effect of cross-linking density is not significant. A sharp increase is induced at approximately pH = 4 as a result of the gradual deprotonation of the carboxyl groups on the PASP chains. The degree of swelling reaches its final constant value at about pH = 6 in both cases. This pH-dependent behaviour is typical for PASP gels independently of the type of cross-linkers.17 A significant difference exists in the degree of swelling of the hydrogels in the reduced and oxidised state, respectively, at alkaline pH values. A larger degree of swelling was achieved in the reduced form caused by the decreased number of chemical cross-links. This observation is in agreement with the prediction of the modified Peppas—Brannon-Peppas model,17 namely, a smaller concentration of net points represents smaller elastic contribution in the total free energy of the polymer network-swelling agent system, and the relative increase in mixing and ionic terms results in an increased degree of swelling of the hydrogel.
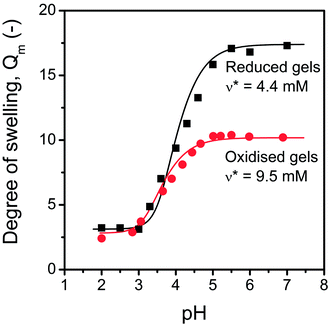 |
| Fig. 3 pH-dependent swelling of poly(aspartic acid) gels (Gel-K) in reduced and oxidised states, respectively (ionic strength was 0.25 M); the calculated cross-link density (ν*) values are indicated. | |
The cross-linking density (ν*) was determined using the model (Fig. 3), and it shows that the cross-linking density can be controlled by the addition of oxidising or reducing agent to the hydrogels. This result confirms the assumed redox induced thiol–disulphide transformation inside the hydrogel. The dual-responsive – pH- and redox-sensitive – behaviour of the proposed hydrogel may find various applications in biomedical areas considering the wide variety of pH- and redox-environments of human tissues.
The redox-response of the elastic modulus of the poly(aspartic acid) gels
The redox stimulus affects not only the degree of swelling but also the mechanical properties of the hydrogels. The stiffness of the hydrogels decreases in the reduced state due to the cleavage of disulphide linkages, while it increases in the oxidised state because of the re-formation of chemical cross-links (Fig. 4).
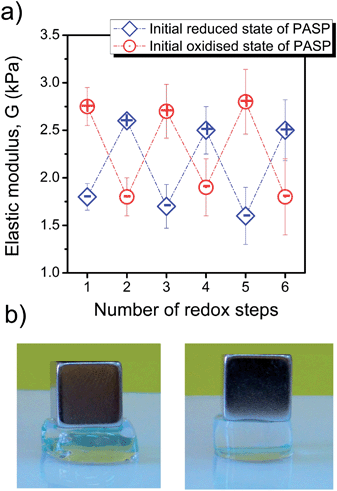 |
| Fig. 4 (a) Reversible redox-response in the elastic modulus of PASP gels (Gel-K) with reduction and oxidation as the initial redox step, respectively, at constant ionic strength (0.15 M). The oxidised state is denoted by + signs and the reduced state by − signs. (b) Photographs of cylindrical PASP gels under the load of a 15 g weight in reduced (left) and in oxidised state (right), respectively. | |
Repeated redox cycles showed reversible response and the gels preserved their mechanical stability for at least three cycles. The reversibility of the process proved to be independent of the type of initial redox state of PASP (reduced or oxidised). A change in stiffness could be easily observed even without an exact measurement of the modulus because the hydrogels became very soft in a reducing medium, and they became stiffer in the presence of an oxidising agent, i.e., oxidation enhanced the mechanical stability of hydrogels (Fig. 4b). It is worth noting that the standard deviation in the elastic modulus increased slightly with the number of cycles due to small cracks developing due to repeated redox reactions. However, the mechanical properties may be improved in the future by the application of long-chained cross-linkers or the homogeneous distribution of chain lengths. Such modifications may enhance the resilience of the hydrogels and increase the number of redox cycles without failure, which is absolutely necessary for uses as sensors and actuators.
Characterisation of thiol–disulphide transformation in poly(aspartic acid) gels
The redox-tunable reversible swelling-shrinking response, the variation of elastic modulus and the difference in pH-dependent swelling of reduced and oxidised hydrogels proved unambiguously that a reversible thiol–disulphide transformation occurs in reducing and oxidising media. Nevertheless, to obtain further direct proof, we intended to develop an analytical method to characterise the conversion of thiol groups to disulphide linkages and vice versa. Ellman's assay is widely used to determine the thiol content in modified polymers; however, the quantification of thiol groups in hydrogels has not been reported in the literature before most likely because of the difficulties in performing the assay. Ellman's assay must be carried out in the absence of redox agents; thus, special care was taken to exclude oxygen during the analysis, and EDTA was used to inhibit the catalytic effect of traces of metal ions, which might have caused the partial oxidation of the reagent. A prolonged reaction time was necessary to determine the concentration of thiol groups in the side chains of the polymer network because of the diffusion-limited, decreased reaction rate inside the gels. However, swollen hydrogels permit the diffusion of the solution of the analyte, and a reaction time of 6 h was sufficient to determine the thiol content accurately as shown in Fig. 5.
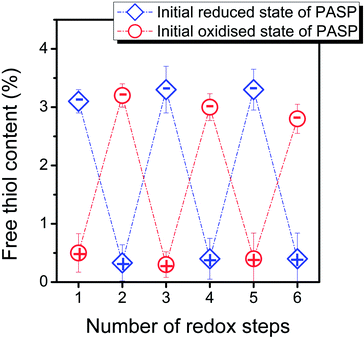 |
| Fig. 5 Cyclic change in free thiol content of PASP gels in reducing and oxidising media, respectively, determined using Ellman's assay. The oxidised state is denoted by + signs and reduced state by − signs. | |
The standard deviation in the thiol content is small, proving the reliability of the measurement. The results of Ellman's assay confirmed that a reversible thiol–disulphide transformation occurs, which led to reversible macroscopic responses in swelling and elastic properties. As a consequence, grafting thiol groups onto PASP rendered redox controllable cross-linking density to our pH-responsive polymer hydrogels.
Conclusions
Poly(aspartic acid) based polymeric matrices have been studied in detail in several recent papers; however, to the best of our knowledge, this is the first report on PASP hydrogels with a reversible response due to external redox stimuli without the dissolution of the gel. A unique synthetic method was developed and is presented in the article. The precursor polymer of poly(aspartic acid), polysuccinimide, was modified with thiol-containing side-groups, then permanently cross-linked with a redox-inert cross-linker 1,4-diaminobutane. Poly(aspartic acid) hydrogels were obtained after subsequent alkaline hydrolysis. PASP hydrogels showed reversible pH-response in their degree of swelling because of the polyelectrolyte character of the network. Moreover, a reversible redox response was also obtained when the hydrogels were immersed in reducing and oxidising media. The response was shown by the swelling behaviour and the elastic modulus of the hydrogels. Gel strength could be improved via oxidation as a consequence. We developed a new analytical method and proved that the macroscopic changes observed were induced by a thiol–disulphide transformation. The redox reaction could be repeated several times. The tunable dual-responsive character of the gels makes them suitable for various future applications, such as sensors, actuators and various biomedical devices.
Acknowledgements
The research was supported by the OTKA Foundation (PD76401), by the NKTH A*STAR Hungarian Singaporean Bilateral S&T International Co-operation (BIOSPONA) and by the János Bolyai Research Scholarship of HAS. The authors appreciate the help of Prof. Tibor Kremmer from the Research Centre for Natural Sciences in the HPLC SEC measurements.
Notes and references
- G. Pasparakis and M. Vamvakaki, Polym. Chem., 2011, 2, 1234–1248 RSC.
- N. A. Peppas, J. Z. Hilt, A. Khademhosseini and R. Langer, Adv. Mater., 2006, 18, 1345–1360 CrossRef CAS.
- S. W. Shalaby, Designed-to-degrade systems, Hanser Publishers, Munich, Vienna, New York, 1994 Search PubMed.
- I. Tokarev and S. Minko, Soft Matter, 2009, 5, 511–524 RSC.
- M. Motornov, Y. Roiter, I. Tokarev and S. Minko, Prog. Polym. Sci., 2010, 35, 174–211 CrossRef CAS PubMed.
- D. Roy, J. N. Cambre and B. S. Sumerlin, Prog. Polym. Sci., 2010, 35, 278–301 CrossRef CAS PubMed.
- C. Zhao, X. Zhuang, P. He, C. Xiao, C. He, J. Sun, X. Chen and X. Jing, Polymer, 2009, 50, 4308–4316 CrossRef CAS PubMed.
- A. Szilágyi and M. Zrínyi, Polymer, 2005, 46, 10011–10016 CrossRef PubMed.
- K. László, K. Kosik and E. Geissler, Macromolecules, 2004, 37, 10067–10072 CrossRef.
- S. Sugiura, A. Szilágyi, K. Sumaru, K. Hattori, T. Takagi, G. Filipcsei, M. Zrínyi and T. Kanamori, Lab Chip, 2009, 9, 196–198 RSC.
- I. Tomatsu, K. Peng and A. Kros, Adv. Drug Delivery Rev., 2011, 63, 1257–1266 CrossRef CAS PubMed.
- A. Szilágyi, K. Sumaru, S. Sugiura, T. Takagi, T. Shinbo, M. Zrínyi and T. Kanamori, Chem. Mater., 2007, 11, 2730–2732 CrossRef.
- T. Shiga, Adv. Polym. Sci., 1997, 134, 131–163 CrossRef CAS.
- G. Filipcsei, I. Csetneki, A. Szilágyi and M. Zrínyi, Adv. Polym. Sci., 2007, 206, 137–189 CrossRef CAS.
- R. París and I. Quijada-Garrido, Eur. Polym. J., 2010, 46, 2156–2163 CrossRef PubMed.
- Y. Wang, Z. C. Yuan and D. J. Chen, J. Mater. Sci., 2012, 47, 1280–1288 CrossRef CAS.
- T. Gyenes, V. Torma, B. Gyarmati and M. Zrínyi, Acta Biomater., 2008, 4, 733–744 CrossRef CAS PubMed.
- P. D. Thornton, R. J. Mart and R. V. Ulijn, Adv. Mater., 2007, 19, 1252–1256 CrossRef.
- T. Vermonden, R. Censi and W. E. Hennink, Chem. Rev., 2012, 112, 2853–2888 CrossRef CAS PubMed.
- Y. Li, J. Rodrigues and H. Tomas, Chem. Soc. Rev., 2012, 41, 2193–2221 RSC.
- T. Traitel, Y. Cohen and J. Kost, Biomaterials, 2000, 21, 1679–1687 CrossRef CAS.
- S. I. Kang and Y. H. Bae, J. Controlled Release, 2003, 86, 115–121 CrossRef CAS.
- L. Yu and J. Ding, Chem. Soc. Rev., 2008, 37, 1473–1481 RSC.
- F. H. Meng, W. E. Hennink and Z. Zhong, Biomaterials, 2009, 30, 2180–2198 CrossRef CAS PubMed.
- F. Q. Schafer and G. R. Buettner, Free Radical Biol. Med., 2001, 30, 1191–1212 CrossRef CAS.
- R. Cheng, F. Feng, F. Meng, C. Deng, J. Feijen and Z. Zhong, J. Controlled Release, 2011, 152, 2–12 CrossRef CAS PubMed.
- C. Legros, M.-C. De Pauw-Gillet, K. C. Tam, S. Lecommmandoux and D. Taton, Polym. Chem., 2013, 4, 4801–4808 RSC.
- M. A. Hempenius, C. Cirmi, J. Song and G. J. Vancso, Macromolecules, 2009, 42, 2324–2326 CrossRef CAS.
- R. Gracia and D. Mecerreyes, Polym. Chem., 2013, 4, 2206–2214 RSC.
- S. Sasaki, S. Koga, R. Yoshida and T. Yamaguchi, Langmuir, 2003, 19, 5595–5600 CrossRef CAS.
- S.-T. Fei, M. V. B. Phelps, Y. Wang, E. Barrett, F. Gandhi and H. R. Allcock, Soft Matter, 2006, 2, 397–401 RSC.
- B. Gyarmati, Á. Némethy and A. Szilágyi, Eur. Polym. J., 2013, 49, 1268–1286 CrossRef CAS PubMed.
- W. Joentgen, N. Müller, A. Mitschker and H. Schmidt, in Biopolymers Online, Wiley-VCH Verlag GmbH & Co. KGaA, 2005 Search PubMed.
- S. M. Thombre and B. D. Sarwade, J. Macromol. Sci., Part A: Pure Appl.Chem., 2005, 42, 1299–1315 CrossRef.
- Á. Némethy, K. Solti, L. Kiss, B. Gyarmati, M. A. Deli, E. Csányi and A. Szilágyi, Eur. Polym. J., 2013, 49, 2392–2403 CrossRef PubMed.
- V. Torma, T. Gyenes, Z. Szakács, B. Noszál, Á. Némethy and M. Zrínyi, Polym. Bull., 2007, 59, 311–318 CrossRef CAS.
- B. Gyarmati, B. Vajna, Á. Némethy, K. László and A. Szilágyi, Macromol. Biosci., 2013, 13, 633–640 CrossRef CAS PubMed.
- M. Zrínyi, T. Gyenes, D. Juriga and J. H. Kim, Acta Biomater., 2013, 9, 5122–5131 CrossRef PubMed.
- S. Shu, X. Wang, X. Zhang, X. Zhang, Z. Wang and C. Li, New J. Chem., 2009, 33, 1882–1887 RSC.
- C. Zheng, X. G. Zhang, L. Sun, Z. P. Zhang and C. X. Li, J. Mater. Sci.: Mater. Med., 2013, 1–9 Search PubMed.
- A. Bernkop-Schnürch, A. E. Clausen and M. Hnatyszyn, Int. J. Pharm., 2001, 226, 185–194 CrossRef.
Footnote |
† Electronic supplementary information (ESI) available. See DOI: 10.1039/c3ra47530a |
|
This journal is © The Royal Society of Chemistry 2014 |
Click here to see how this site uses Cookies. View our privacy policy here.