DOI:
10.1039/C3RA45994B
(Paper)
RSC Adv., 2014,
4, 9830-9837
Supported CuBr on graphene oxide/Fe3O4: a highly efficient, magnetically separable catalyst for the multi-gram scale synthesis of 1,2,3-triazoles†
Received
21st October 2013
, Accepted 23rd January 2014
First published on 28th January 2014
Abstract
A superparamagnetic graphene oxide (GO)/Fe3O4–CuBr catalyst was prepared via a simple chemical method and characterized by Fourier transform infrared (FTIR), thermal gravimetric analysis (TGA), transmission electron microscopy (TEM), powder X-ray diffraction (XRD) and X-ray photoelectron spectroscopy (XPS). The GO/Fe3O4–CuBr catalyst could be dispersed homogeneously in water and further used as an excellent semi-heterogeneous catalyst for the one-pot multi-component Cu(I)-catalyzed azide-alkyne 1,3-dipolar cycloaddition (CuAAC) reaction. 1,4-Disubstituted mono/bis-1,2,3-triazoles are readily obtained on a multi-gram scale by the reaction of aryl/alkyl halides, alkynes and sodium azide under microwave irradiation conditions in good to excellent yields. Moreover, GO/Fe3O4–CuBr could be separated conveniently from the reaction mixtures by an external permanent magnet and reused in at least six consecutive runs without a noticeable drop in the product yield and its catalytic activity.
Introduction
In recent years, the Cu(I)-catalyzed azide-alkyne 1,3-dipolar cycloaddition (CuAAC) reaction,1–5 which was coined as “click reaction”, has attracted great attention not only from academia but also for applications.6–11 In this context, many efforts have been made to further develop CuAAC, which mainly focused on one-pot multicomponent CuAAC reaction.12–33 The one-pot multicomponent CuAAC reaction has been recognized as one of the most attractive synthetic tools due to the green, efficient and convenient preparation of 1,2,3-triazole derivatives, whose subunits are extensively used in medicinal chemistry as a pharmacophore to modify known bioactive molecules and to potentiate their biological activities.
Generally, copper salts and ligands have been used as homogeneous catalyst systems to catalyze one-pot synthesis of 1,2,3-triazoles from aryl/alkyl halides, alkynes and sodium azide. Although homogeneous copper catalysts have many advantages, the homogeneous catalysts are difficult to be recovered and reused, and the residual copper metal along with the products can cause serious problems in the synthesis of bioactive molecules. Moreover, it is difficult to be be used in large-scale syntheses particular on environmental and economic concerns.
In this regard, heterogenization of the copper catalysts can be an attractive solution to this problem since the copper salts immobilized on a support can be easily separated from the obtained products free of metal residues and recycled. There has been considerable interest in the development of heterogeneous CuAAC catalytic systems that can be efficiently reused whilst keeping the inherent activity of the catalytic center. Up to now, a lot of efforts have been made to design and synthesize recoverable catalysts. Immobilizing the CuAAC catalysts directly onto various inorganic and organic supports,34 such as magnetic nanoparticles (MNPs),35–38 zeolite,39,40 polymers41–50 and SiO251–55 have been explored. However, the preparation procedures of the catalysts supported on these materials were relatively cumbersome and require multiple steps.
In recent years, carbocatalysis has been paid much attention as it is cheap and easily obtained, and carbon materials have been widely used as heterogeneous catalysts. Catalysts based on carbon materials, such as activated carbon and carbon nanotubes, have also been studied as CuAAC catalyst supports.56,57 These catalysts showed high catalytic activity and could be reused for several cycles, but they were difficult to separate from the reaction mixtures, and filtration (or high speed centrifugation) was required.
Different from activated carbon and carbon nanotubes, graphene oxide (GO) has been demonstrated as a promising support for heterogeneous nanocatalysts due to their intrinsic properties such as high chemically stability, large surface area and good accessibility.58 Most importantly, GO has rich surface functional groups (mainly epoxide, hydroxyl and carboxyl), which made GO as a suitable support material for metal and metal oxide nanoparticles. Recently, it was reported that many types of inorganic nanomaterials could be deposited onto GO nanosheet surfaces to impart new functionality to this increasingly popular 2D nanomaterial in consideration of its large surface area. For example, Fe3O4 nanoparticles (Fe3O4 NPs) has been successfully introduced on the surface or inter-plane of GO.59–61 The unique properties of GO/Fe3O4 hybrids, combining effects from GO and Fe3O4 NPs have opened a new window for fabricating highly stable multifunctional nanomaterials by using these hybrids as support materials.
Unfortunately, as far as we know, in spite of the development of GO/Fe3O4 supported catalysts, GO/Fe3O4-supported CuAAC catalysts have still not been reported. In addition, the above noted papers reported only the synthesis of 1,4-disubstituted mono-1,2,3-triazoles by using heterogeneous catalysts. In order to further improve the efficiency and practicality of the CuAAC reaction, herein we develop a green, efficient and scale-up synthesis of 1,4-disubstituted mono/bis-1,2,3-triazoles from aryl/alkyl halides, alkynes and sodium azide in water, which catalyzed by magnetically recyclable GO/Fe3O4–CuBr via a combination of one-pot multi-component CuAAC reaction and microwave irradiation (Scheme 1).
 |
| Scheme 1 One-pot synthesis of 1,4-disubstituted mono/bis-1,2,3-triazoles catalyzed by GO/Fe3O4–CuBr in water under microwave irradiation condition. | |
Preparation and characterization of GO/Fe3O4–CuBr
The concise route for the preparation of GO/Fe3O4–CuBr is outlined in Scheme 2.
 |
| Scheme 2 Schematic representation of the preparation and magnetic separation of GO/Fe3O4–CuBr catalyst from water by an external magnet after 30 s. | |
GO and GO/Fe3O4 were prepared according to the modified Hummer's method62,63 and Song's method,64 respectively. The obtained GO/Fe3O4 was then treated with CuBr in ethanol at reflux temperature for 24 hours under a N2 atmosphere to generate GO/Fe3O4–CuBr catalyst (Scheme 2). The catalyst has been characterized by FTIR, TGA, atomic absorption spectroscopy (AAS), TEM, XRD and XPS.
As can be seen from Table 1, the peaks at near 1722 cm−1 were characteristic bands of C
O stretching vibration, indicating that the GO was successfully synthesized. However, the peak at 1722 cm−1 disappeared due to the formation of –COO− after coating with Fe3O4. In addition, Table 1 also showed the FTIR spectra of GO/Fe3O4 and GO/Fe3O4–CuBr before and after the complexing reaction with CuBr. Obviously, when GO/Fe3O4 complexed with CuBr, the –Fe–O– stretching peak of GO/Fe3O4 appeared at 589 cm−1 was shifted to 580 cm−1. The characteristic –C
C– bands appeared at 1644 cm−1 were shifted to 1642 cm−1. The lowering in frequency of the above peaks indicates the formation of the metal–ligand bond.
Table 1 FTIR and microanalysis of GO/Fe3O4–CuBr catalyst
|
FTIR (KBr, cm−1) |
Microanalysis (wt%) |
AAS analysis results of fresh catalysts. AAS analysis results of GO/Fe3O4–CuBr after six consecutive trials. |
Sample |
νC O |
νC C |
νFe–O |
Cua |
Cub |
GO |
1722 |
1634 |
— |
— |
— |
GO/Fe3O4 |
— |
1644 |
589 |
— |
— |
GO/Fe3O4–CuBr |
— |
1642 |
580 |
10.115 |
8.504 |
The percentage of copper contents of the fresh GO/Fe3O4–CuBr and the recycled GO/Fe3O4–CuBr after six consecutive trials were determined by AAS (Table 1). The catalysts were stirred in dil. HNO3 for 10 h and then subjected to AAS analysis. The percentage of copper contents of them were found to be 10.115 and 8.504 wt%, respectively.
The morphology GO/Fe3O4–CuBr and the reused catalyst was determined by TEM. As Fig. 1a showed, Fe3O4 nanoparticles are chemically deposited on GO with the aid of the –COOH on GO. Some Fe3O4 aggregation is observed. The results of TEM indicated the nano-sized organic–inorganic hybrid materials were prepared. The morphology of the used catalyst did not show any significant change even after six reaction cycles, which proved its robustness (Fig. 1b).
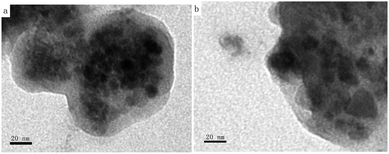 |
| Fig. 1 TEM images of GO/Fe3O4–CuBr (a) and the recycled catalyst after 6 consecutive trials (b). | |
Fe and Cu elements were detected on the whole GO surface by energy-dispersive X-ray analysis (EDX) analysis. Fig. 2 shows typical EDX mapping images of Fe (red) and Cu (green) elements, which are from Fe3O4 and CuBr of GO/Fe3O4–CuBr (a) and the recycled catalyst after 6 consecutive trials (b), respectively. Fig. 2a shows Fe3O4 and CuBr are uniformly coated onto GO. However, Fig. 2b shows the Cu of the recycled catalyst after six cycles was lower than the fresh catalyst.
 |
| Fig. 2 EDX elemental mapping images of the surface of GO/Fe3O4–CuBr (a) and the recycled catalyst after 6 consecutive trials (b). | |
The thermal behaviors of GO, GO/Fe3O4 and GO/Fe3O4–CuBr are further investigated by TGA. As shown in Fig. 3, the GO powders exhibit two steps of mass loss at 150 °C and 300 °C with 9% and 35% weight loss, which are attributed the removal of adsorbed water and oxygen-containing functional groups, respectively (Fig. 3a). An obvious weight loss (15 wt%) between 350 °C and 550 °C is observed, which can be assigned to the decomposition and vaporization of various oxygen-containing functional groups at different positions on the surface of the GO/Fe3O4 hybrid composite (Fig. 3b). However, GO/Fe3O4–CuBr exhibits a higher thermal stability rather than both GO and GO/Fe3O4 (Fig. 3c). A slow weight loss (about 1.5 wt%) at low temperature (<100 °C) was observed, which could be assigned to the loss of the residual or absorbed solvent. The GO/Fe3O4–CuBr catalyst possesses good thermal stabilities (up to 500 °C), which meets the demands for potential applications in catalysis.
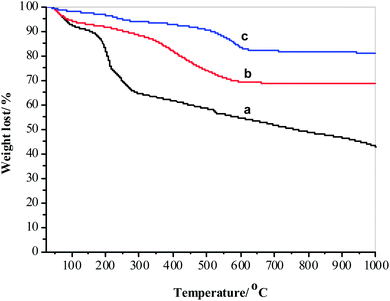 |
| Fig. 3 TGA curves of GO (a), GO/Fe3O4 (b), GO/Fe3O4–CuBr (c). | |
The XRD patterns of graphite, GO, GO/Fe3O4 and GO/Fe3O4–CuBr are presented in Fig. 4. The original graphite shows a sharp characteristic diffraction peak at 2θ = 26.5°, corresponding to the (002) crystal plane with a d-spacing of 0.34 nm. After the oxidation treatment, the (002) diffraction peak for graphite shifted to lower angle at 2θ = 12.5°, indicating the formation of GO. In comparison with the natural graphite, the enhancement of the interlayer spacing of GO is mainly due to the presence of oxygen-containing functional groups formed during oxidation on the graphite sheets. In addition, the characteristic peak of pristine graphite at 2θ = 26.5° disappears after strong oxidation. These data suggest that graphite is successfully converted to GO after oxidation by Hummer's method. The main peaks in Fig. 4c at 2θ = 30.1° (220), 35.6° (311), 43.6° (400), 53.6° (422), 57.5° (511), and 62.8° (440) show the characteristics of Fe3O4 (JCPDS no. 19-629). Three additional peaks at 2θ = 27.1°, 45.2°, and 53.2° corresponding to the (111), (220), and (311) planes of CuBr are observed in the pattern of the GO/Fe3O4–CuBr composite (Fig. 4d), indicating the existence of CuBr. Fig. 4d shows the diffraction peaks of Fe3O4 and CuBr all clearly broadened, which suggested that both of them were successfully grafted onto GO sheets. These results of XRD confirm the successful preparation of GO/Fe3O4–CuBr.
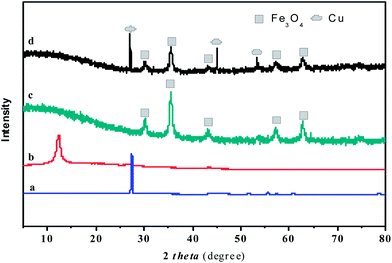 |
| Fig. 4 XRD Patterns of graphite (a), GO (b), GO/Fe3O4 (c), GO/Fe3O4–CuBr (d). | |
Magnetic properties of GO/Fe3O4–CuBr and the used GO/Fe3O4–CuBr after six recycling were investigated using VSM. Fig. 1 shows the magnetization curves of the original GO/Fe3O4–CuBr (Fig. 5a) and the used sample after 6 recycling (Fig. 5b) at room temperature. The obtained results revealed that the samples had a suitable property for magnetic actuation and manipulation. The values of saturation magnetization decreased from 17.632 to 9.8049 emu g−1 after six consecutive trials, which possibly originated from the decrease of loading amount of Fe3O4 nanoparticles on the GO surface during using under microwave heating condition.
 |
| Fig. 5 The magnetization curve of (a) GO/Fe3O4–CuBr and (b) GO/Fe3O4–CuBr after 6 recycling. | |
XPS was used to study surface elemental composition of GO/Fe3O4–CuBr and GO/Fe3O4–CuBr after 6 recycling. As shown in Fig. S13,† the C1s spectra can be deconvoluted into two main components corresponding to carbon atoms in different functional groups, which exhibits the presence of C–C (284.5 eV), C–O groups (286.5 eV). The binding energies of Cu2p3/2 in GO/Fe3O4–CuBr and the recycled GO/Fe3O4–CuBr catalyst were both found to be around 932.5 eV (Fig. S14†), suggesting no change in chemical valence of Cu(I) in the supported catalyst after 6 recycling. In addition, the concentrations of surface Cu and Fe atoms of them decreased from 3.54% and 4.79 to 2.76% and 4.39% after 6 recycling, respectively, confirming only a few Fe3O4 and CuBr from the GO/Fe3O4–CuBr catalyst were leached into the solution after 6 recycling. This may account for the slight decrease in catalytic activity.
Effect of catalysts on the one-pot CuAAC reaction under microwave assisted and conventional heating conditions
For the initial screening experiments, NaN3, benzyl chloride and phenyl acetylene were selected as the test substrates to examine the effects of various CuAAC catalysts in water at 80 °C under microwave irradiation and conventional heating conditions (Table 2).
Table 2 Effect of the Cu catalysts on the one-pot synthesis of 1-benzyl-4-phenyl-1H-1,2,3-triazole under microwave assisted and conventional heating conditionsa

|
Entry |
Cu catalyst |
Yieldb (%) |
Yieldc (%) |
Cu contentd (%) |
Reagents and conditions: BnCl (0.50 mmol), PhC CH (0.50 mmol), NaN3 (0.50 mmol), Cu catalyst (0.025 mmol Cu, 5 mol%), water (2.0 mL). Isolated yield under microwave irradiation condition, 10 min. Isolated yield by using conventional heating, 8 h. Cu content of the obtained 1,2,3-triazole under microwave irradiation condition. |
1 |
Cu(OAc)2 |
Trace |
Trace |
— |
2 |
CuBr |
65 |
96 |
6.540 |
3 |
GO/Fe3O4–CuBr |
98 |
98 |
0.012 |
Optimization studies revealed that CuAAC reaction could perform with low yield by using Cu(OAc)2 as catalyst (Table 2, entry 1). When CuBr was used as catalyst, CuAAC reaction could only give a moderate yield (Table 2, entry 2). Based on these frustrating results, we continued our research to improve the yield of the product by using other catalysts. To our delight, the reaction performed smoothly with the use of GO/Fe3O4–CuBr and could give an excellent yield (Table 2, entry 3).
Under conventional heating condition, we investigated the activity of the catalysts with respect to time for the formation of triazole and found 8 h is the optimized reaction time at 80 °C. When CuBr and GO/Fe3O4–CuBr was used as catalyst, the yields of the obtained 1,2,3-triazole were found to be 96% and 98%, respectively (Table 2). Comparing with the traditional heating method, the use of microwave irradiation could dramatically reduce the reaction time. As a result, GO/Fe3O4–CuBr was used in the subsequent investigations under microwave irradiation condition because of its high reactivity, high selectivity and easy separation.
Copper is an essential trace metal in man but it is toxic at higher concentrations. Accordingly, the percentage of copper contents of the obtained 1,2,3-triazole in the model reaction under heterogeneous and homogeneous catalytic conditions were determined by AAS (Table 2). The copper contents of the product prepared by CuBr and GO/Fe3O4–CuBr as catalyst were found to be 6.540 and 0.012 wt%, respectively. It could obviously reduce the level of copper metal in the final products by using GO/Fe3O4–CuBr as heterogeneous catalyst.
Synthesis of 1,4-disubstituted mono/bis-1,2,3-triazoles catalyzed by GO/Fe3O4–CuBr
Having determined the optimal conditions, we proceeded to investigate the scope of this one-pot CuAAC reaction of different halides and alkynes under the optimized reaction conditions in aqueous medium. The results are summarized in Table 3. The GO/Fe3O4–CuBr catalyzed CuAAC reaction of NaN3/halides with alkynes was performed under the standard condition: GO/Fe3O4–CuBr (5.0 mol%) as the catalyst and water as the solvent at 80 °C without exclusion of air (Table 3). As shown in Table 3, all the reactions were completed within a time period of 5–25 min with high selectivity and conversion, depending on the substrates. And the corresponding triazoles were obtained in good to excellent yields. In general, no significant difference in reactivity was observed for the examined reactants with varied electronic properties. Both electron-rich and electron-poor azides as reactants generated good to excellent yields of the products. Moreover, this cycloaddition also proceeded successfully in the case of diynes like 1,4-bis(prop-2-ynyloxy)benzene and 1,3-bis(prop-2-ynyloxy)benzene, although the reaction time was longer than other substrates (Table 3, entries 6–9). These results highlight the compatibility of this cascade process with a wide range of functionalities. Furthermore, it is important to note that the corresponding triazoles were obtained in high yields and regioselectivity. The structures of the obtained products were conformed by FTIR, 1H NMR, 13C NMR spectroscopy and mass spectrometry, respectively.
Table 3 GO/Fe3O4–CuBr used in the one-pot CuAAC reaction for the regiospecific formation of 1,4-disubstituted mono/bis-1,2,3-triazoles under microwave irradiation in watera
Entry |
Halide |
Alkyne |
Time (min) |
Yieldb (%) |
Reagents and conditions: R1X (0.50 mmol), R2CH2C CH (0.50 mmol) or R2CH(C CH)2 (0.25 mmol), NaN3 (0.50 mmol), GO/Fe3O4–CuBr (0.025 mmol Cu, 5.0 mol% of catalyst), water (2.0 mL), 480 W, 80 °C. Isolated yields. |
1 |
 |
 |
5 |
92 (ref. 65) |
2 |
 |
 |
10 |
98 (ref. 65) |
3 |
 |
 |
8 |
96 (ref. 42) |
4 |
 |
 |
5 |
90 |
5 |
 |
 |
15 |
90 |
6 |
 |
 |
15 |
89 |
7 |
 |
 |
18 |
91 |
8 |
 |
 |
20 |
88 |
9 |
 |
 |
25 |
89 |
Remarkably, compared with other similar magnetic nanocatalyst (such as MNPs–CuBr38), the GO/Fe3O4–CuBr catalyst showed higher catalytic activity during the one-pot multicomponent CuAAC reaction. For example, the one-pot reactions could proceed smoothly to completion within 10 min (Table 3, entries 1–3), and the products were isolated in excellent yields (92–98%). The intrinsic properties (large surface area and good accessibility) of GO might cause the extremely high catalytic activity of GO/Fe3O4–CuBr.
Reuse test of GO/Fe3O4–CuBr catalyst
For a true heterogeneous catalyst, the reusability is one of the important properties. In order to make our catalytic system greener and economical, we focused on reusability of GO/Fe3O4–CuBr catalyst on multicomponent reaction of phenyl acetylene, NaN3 and benzyl chloride. A series of catalytic cycles were run to investigate the constancy of the catalyst activity for this model reaction. After each run, the catalyst was quickly concentrated to the side wall of the reactor via an external permanent magnet. After a simple wash using ethanol and air drying the catalyst was used for the next cycle. The recovered catalyst could be added to fresh substrates under identical conditions for six runs without a noticeable drop in the product yield and its catalytic activity (Fig. 6). As shown in Table 1, the copper content of the recycled catalyst after six cycles was found to be 8.504 wt% detected by AAS, which lower than the flesh catalyst (10.115 wt%). The possible reason was the complexing ability between surface functional groups (mainly epoxide, hydroxyl and carboxyl) of GO and CuBr were relatively weak. It caused a slight decrease of catalytic activity of GO/Fe3O4–CuBr catalyst after several recycles (the yield was reduced by 8% after six recycles, Fig. 4).
 |
| Fig. 6 Reusability of the catalyst using sodium azide, benzyl chloride and phenyl acetylene as starting materials. | |
Scale-up synthesis of 1-benzyl-4-phenyl-1H-1,2,3-triazole
Prompted by the aforementioned interesting results like the high catalytic activity of GO/Fe3O4–CuBr, we set out to further clarify the practicality of the catalyst. To extend this strategy to the multi-gram scale synthesis of 1,2,3-triazole derivatives, we investigated the scale-up preparation of 1-benzyl-4-phenyl-1H-1,2,3-triazole as a model reaction. Encouraged by the above results, we increased the scale of the reaction to 5.0, 10.0, 20.0 and 40.0 mmol, keeping the reaction stoichiometry unchanged. The reactions were found to proceed in good to excellent yields without undesired side reactions. When the scale of the reaction was increased to 40.0 mmol, the reaction was still found to proceed successfully and the corresponding product was obtained in 75% yield. Most importantly, when the scale of the reaction was 10.0 mmol, the catalyst could be recycled and reused at least four times. The detailed results were summarized in the Table 4.
Table 4 Scale-up synthesis of 1-benzyl-4-phenyl-1H-1,2,3-triazole
Entry |
Scale (mmol) |
H2O (mL) |
Yielda (%) |
isolated yield. catalyst recycled and used for 4nd run. catalyst recycled and used for 4nd run. catalyst recycled and used for 4nd run. |
1 |
0.5 |
2 |
98 |
2b |
0.5 |
2 |
94 |
3 |
5 |
20 |
96 |
4c |
5 |
20 |
92 |
5 |
10 |
40 |
88 |
6d |
10 |
40 |
86 |
7 |
20 |
80 |
80 |
8 |
40 |
120 |
75 |
Conclusions
In summary, a new kind of GO/Fe3O4-supported semi-heterogeneous catalyst, namely GO/Fe3O4–CuBr, were firstly prepared. It shows high catalytic activity for three-component CuAAC reaction, and it has been used successfully for efficient, green and scale-up preparation of 1,4-disubstituted mono/bis-1,2,3-triazoles. More importantly, the magnetic GO/Fe3O4–CuBr catalyst can be quickly and completely recovered by simply applying an external magnet and the efficiency remains unaltered even after four recycles when the scale of the reaction is 10.0 mmol. Furthermore, when the reaction is performed in H2O on 40.0 mmol scale, the desired product was also obtained in 75% yield. The mild reaction condition, high yields of products, ease of work-up, ecologically clean procedure, and scale-up synthesis will make the present method a useful and important addition to the methodologies for the synthesis of mono/bis-1,2,3-triazoles.
Experimental section
The IR spectra were obtained using a FTIR (4000–400 cm−1) spectrometer (Nicolet Nexus FTIR spectrometer, USA) at 4 cm−1 resolution and 32 scans. Samples were prepared using the KBr disc method. The amount of copper in GO/Fe3O4–CuBr was determined by AAS using standard methods with a Varian AA275 atomic absorption spectrophotometer (USA). Size and morphology images of GO/Fe3O4–CuBr were obtained by TEM (Hitachi H-7650, Japan) operating at 100 KV after a drop of aqueous solution of the nanoparticles was deposited and dried on a copper grid. NMR spectra were acquired in CDCl3 on a Bruker DMX-400 spectrometer at 400 MHz for 1H NMR, 100 MHz for 13C NMR the chemical shifts are given in δ values from TMS as an internal standard. Powder XRD was used to characterize the crystalline structure of the nanoparticles on Bruker D8 Advance (Germany) using Cu Kα radiation. X-ray photoelectron spectroscopy (XPS) measurements were performed with ESCA 2000 (VG Microtech) system.
n-Butyl bromide (99%), benzyl chloride (99%), Phenylacetylene (97%), FeSO4·7H2O (99%), FeCl3 (99%), NH3·H2O (30%), hydrogen peroxide (30%), propargyl bromide (80% in toluene) and NaN3 (99%) were purchased from Shanghai Sinopharm Chemicals Co., Propargyl alcohol (97%) was purchased from Wuhan Hechang Chemicals Co., methyl 2-(prop-2-ynyloxy) benzoate (97%) and 1-isopropyl-4-methyl-2-(prop-2-ynyloxy)benzene (98%) were purchased from Xiamen Lvyin Chemicals Co. Compounds 5b, 6b and 7b (all of purity ≥ 98%) were synthesized according to the literature procedures, respectively.66–68 All other chemicals were used without further purification. All the one-pot CuAAC reactions were carried out in a professional microwave reactor equipped with a stirring bar.
Preparation of GO/Fe3O4–CuBr catalyst
GO was synthesized according to previous methods.62,63 The GO/Fe3O4 nanocomposite was prepared by the chemical co-precipitation method similar to that reported previously.64 GO (200 mg) was dispersed into deionized water (250 mL) under ultrasonication for 30 min. FeCl3 (1.0 mmol) was added into the aqueous dispersion solution. The mixture was stirred overnight at 65 °C. A NH3·H2O solution (30 mL, 25–28%) and FeSO4·7H2O (0. 5 mmol) were added, respectively. After the resulted mixture was stirred for another 2 h at 65 °C, the precipitate was separated by an external permanent magnet, and was washed with distilled water in turn until neutralization and dried under vacuum. The GO/Fe3O4–CuBr nanocomposite was prepared as follows: in a small Schlenk tube, the obtained GO/Fe3O4 (1.00 g) was then treated with CuBr (0.20 g) in ethanol (50 mL), the mixture was stirred under a N2 atmosphere at reflux temperature for 12 hours. The GO/Fe3O4–CuBr catalyst was separated by an external permanent magnet, wash with deionized water and ethanol followed by drying under vacuum at 60 °C overnight for further use.
General procedure for one-pot synthesis of the 1,2,3-triazoles
The primary halide, NaN3 and terminal alkyne in a 1
:
1
:
1 molar ratio followed by adding GO/Fe3O4–CuBr catalyst in a 10.0 mL round-bottomed flask (H2O, 2.0 mL). The mixture was heated and stirred at 80 °C under microwave irradiation (MW, 480 W) until TLC analysis showed that the reaction was complete. After completion of the reaction, the catalyst was separated conveniently from the reaction mixtures by an external permanent magnet. After a simple wash using ethanol and air drying the catalyst was used for the next cycle. the reaction mixture was extracted with ethyl acetate for three times. Then the combined organic layers were dried (MgSO4) and evaporated under reduced pressure. The residue was finally purified by flash chromatography on silica-gel to give the desired 1,2,3-triazoles in 88–98% yields (Table 3).
Analytical and spectroscopic data of the 1,2,3-triazoles
Methyl 2-((1-benzyl-1H-1,2,3-triazol-4-yl) methoxy) benzoate (Table 3 entry 4). M.p. (°C): 108–109. IR (KBr) (νmax, cm−1): 3151 (w), 3075 (w), 3034 (w), 2952 (s), 1722 (s), 1598 (m), 1432 (m), 1245 (s), 1083 (m), 815 (m), 765 (m), 704 (m); 1H NMR (400 MHz, CDCl3) δ: 7.82 (d, J = 7.7 Hz, 1H), 7.67 (s, 1H), 7.48 (t, J = 7.8 Hz, 1H), 7.38 (s, 3H), 7.29 (d, J = 4.9 Hz, 2H), 7.13 (d, J = 8.4 Hz, 1H), 7.02 (t, J = 7.5 Hz, 1H), 5.55 (s, 2H), 5.32 (s, 2H), 3.82 (s, 3H); 13C NMR (CDCl3) δ: 51.89 (1C, CH3), 54.29 (1C, CH2Ph), 63.60 (1C, OCH2), 114.18, 120.62, 121.00, 123.21, 128.08, 128.76, 129.12, 131.75, 133.64, 134.57, 157.78 (C–Ar), 166.43 (1C, CO); MS (ESI): m/z calc. for C18H17N3O3: 323.13, found: 324.04 [M + H]+, 346.02 [M + Na]+.
4-((2-Isopropyl-5-methylphenoxy)methyl)-1-benzyl-1H-1,2,3-triazole (Table 3 entry 5). M.p. (°C): 75–76. IR (KBr) (νmax, cm−1): 2969 (m), 2923 (m), 2867 (s), 1616 (s), 1503 (s), 1447 (m), 1253 (m), 1174 (m), 1105 (m), 1026 (m), 822 (m); 1H NMR (400 MHz, CDCl3) δ: 7.52 (s, 1H), 7.46–7.35 (m, 3H), 7.33–7.27 (m, 2H), 7.14–7.07 (m, 1H), 6.79 (d, J = 5.5 Hz, 2H), 5.57 (s, 2H), 5.23 (s, 2H), 3.25 (dt, J = 13.8, 6.9 Hz, 1H), 2.33 (s, 3H), 1.18 (d, J = 6.9 Hz, 6H); 13C NMR (CDCl3) δ: 155.31, 145.37, 136.45, 134.63, 134.33, 129.13, 128.76, 127.99, 126.02, 122.26, 112.96 (C–Ar), 62.62 (1C, OCH2), 54.20 (1C, CH2Ph), 26.54 (1C, C), 22.77 (1C, CH3), 21.32 (2C, CH3); MS (ESI): m/z calc. for C20H23N3O: 321.18, found: 322.08 [M + H]+.
4-((4-((1-Benzyl-1H-1,2,3-triazol-4-yl)methoxy)phenoxy)methyl)-1-benzyl-1H-1,2,3-triazole (Table 3 entry 6). M.p. (°C): 202–204. IR (KBr) (νmax, cm−1): 3122 (w), 3106 (w), 2958 (m), 2907 (m), 2864 (m), 1512 (s), 1443 (m), 1228 (m), 1124 (m), 1047 (m), 806 (m), 702 (m); 1H NMR (400 MHz, CDCl3) δ: 7.58 (s, 2H), 7.53–7.33 (m, 6H), 7.29 (d, J = 4.7 Hz, 4H), 6.90 (s, 4H), 5.55 (s, 4H), 5.14 (s, 4H). 13C NMR (CDCl3) δ: 54.32 (1C, CH2Ph), 62.74 (2C, OCH2), 115.86, 128.13, 128.83, 129.15, 134.46, 152.18 (C–Ar). MS (ESI): m/z calc. for C26H24N6O2: 452.20, found: 453.16 [M + H]+.
4-((4-((1-Butyl-1H-1,2,3-triazol-4-yl)methoxy)phenoxy)methyl)-1-butyl-1H-1,2,3-tria-zole (Table 3 entry 7). M.p. (°C): 125–127. IR (KBr) (νmax, cm−1): 3148 (w), 3096 (w), 2949 (s), 2881 (m), 1512 (s), 1452 (s), 1219 (s), 1107 (w), 1047 (s), 827 (s), 573 (m); 1H NMR (400 MHz, CDCl3) δ: 7.59 (s, 2H), 6.94 (s, 4H), 5.18 (s, 4H), 4.37 (t, J = 7.2 Hz, 4H), 1.98–1.82 (m, 4H), 1.44–1.32 (m, 4H), 0.97 (t, J = 7.3 Hz, 6H). 13C NMR (CDCl3) δ: 31.60 (2C, CH3), 32.23 (2C, CH3CH2), 50.15 (2C, CH3CH2CH2), 61.84 (2C, CH3CH2CH2CH2), 62.79 (1C, OCH2), 115.29, 115.86, 122.39, 144.31, 152.82 (C–Ar). MS (ESI): m/z calc. for C20H28N6O2: 384.23, found: 385.12 [M + H]+.
4-((3-((1-Benzyl-1H-1,2,3-triazol-4-yl)methoxy)phenoxy)methyl)-1-benzyl-1H-1,2,3-triazole (Table 3 entry 8). M.p. (°C): 171–172. IR (KBr) (νmax, cm−1): 3139 (w), 3088 (w), 3066 (w), 2924 (m), 2864 (m),1615 (s), 1494 (s), 1443 (m), 1382 (m), 1185 (s), 1021 (s), 831(m), 780 (m), 728 (m); 1H NMR (400 MHz, CDCl3) δ: 7.57 (s, 2H), 7.40 (dd, J = 9.7, 6.1 Hz, 6H), 7.32–7.28 (m, 4H), 7.17 (d, J = 8.2 Hz, 1H), 6.60 (d, J = 7.7 Hz, 3H), 5.55 (s, 4H), 5.16 (s, 4H). 13C NMR (CDCl3) δ: 54.31 (1C, CH2Ph), 62.12 (2C, OCH2), 102.12, 107.64, 128.15, 128.84, 129.17, 130.06, 134.44, 159.42 (C–Ar). MS (ESI): m/z calc. for C26H24N6O2: 452.20, found: 453.15 [M + H]+.
Methyl 3,5-bis((1-benzyl-1H-1,2,3-triazol-4-yl) methoxy) benzoate (Table 3 entry 9). M.p. (°C): 146–147. IR (KBr) (νmax, cm−1): 3146 (w), 3093 (w), 1720 (s), 1666 (s), 1596 (m), 1452 (m), 1299 (m), 1160 (s), 1049 (s), 817 (m), 721 (m); 1H NMR (400 MHz, CDCl3) δ: 7.56 (s, 2H), 7.38 (s, 6H), 7.30–7.25 (m, 6H), 6.80 (s, 1H), 5.55 (s, 4H), 5.19 (s, 4H), 3.90 (s, 3H). 13C NMR (CDCl3) δ: 52.31 (1C, OCH3), 54.29 (2C, CH2Ph), 62.31 (2C, OCH2), 106.95, 108.69, 127.79, 127.53, 128.14, 128.85, 129.18, 132.18, 134.42, 159.23, 166.53 (C–Ar). MS (ESI): m/z calc. for C28H26N6O4: 510.20, found: 511.13 [M + H]+.
Acknowledgements
National Natural Science Foundation of China (no. 21004024), Natural Science Foundation of Fujian province (no. 2011J01046) Program for New Century Excellent Talents in University of Fujian province (no. 2012FJ-NCET-ZR03) and Promotion Program for Young and Middle-aged Teacher in Science and Technology Research of Huaqiao University (ZQN-YX103) are appreciated.
Notes and references
- C. W. Tornøe, C. Christensen and M. Meldal, J. Org. Chem., 2002, 67, 3057 CrossRef.
- V. V. Rostovtsev, L. G. Green, V. V. Fokin and K. B. Sharpless, Angew. Chem., Int. Ed., 2002, 41, 2596 CrossRef CAS.
- M. Morten and W. T. Christian, Chem. Rev., 2008, 108, 2952 CrossRef PubMed.
- Q. Wang and C. Hawker, Chem.–Asian J., 2011, 6, 2568 CrossRef CAS PubMed.
- X. Q. Xiong, L. Cai and Z. K. Tang, Chin. J. Org. Chem., 2012, 32, 1410 CrossRef CAS.
- J. E. Moses and A. D. Moorhouse, Chem. Soc. Rev., 2007, 36, 1249 RSC.
- R. K. Iha, K. L. Wooley, A. M. Nystrom, D. J. Burke, M. J. Kade and C. J. Hawker, Chem. Rev., 2009, 109, 5620 CrossRef CAS PubMed.
- H. C. Kolb and K. B. Sharpless, Drug Discovery Today, 2003, 8, 1128 CrossRef CAS PubMed.
- J.-F. Lutz, Angew. Chem., Int. Ed., 2007, 46, 1018 CrossRef CAS PubMed.
- W. H. Binder and R. Sachsenhofer, Macromol. Rapid Commun., 2007, 28, 15 CrossRef CAS.
- G. C. Tron, T. Pirali, R. A. Billington, P. L. Canonico, G. Sorba and A. A. Genazzani, Med. Res. Rev., 2008, 28, 278 CrossRef CAS PubMed.
- A. K. Feldman, B. Colasson and V. V. Fokin, Org. Lett., 2004, 6, 3897 CrossRef CAS PubMed.
- P. Appukkuttan, W. Dehaen, V. V. Fokin and E. Van der Eycken, Org. Lett., 2004, 6, 4223 CrossRef CAS PubMed.
- S. Chittaboina, F. Xie and Q. Wang, Tetrahedron Lett., 2005, 46, 2331 CrossRef CAS.
- H. S. G. Beckmann and V. Wittmann, Org. Lett., 2007, 9, 1 CrossRef CAS PubMed.
- K. Odlo, E. A. Høydahl and T. V. Hansen, Tetrahedron Lett., 2007, 48, 2097 CrossRef CAS.
- C. T. Lee, S. L. Huang and B. H. Lipshutz, Adv. Synth. Catal., 2009, 351, 3139 CrossRef CAS.
- F. Friscourt and G. J. Boons, Org. Lett., 2010, 12, 4936 CrossRef CAS PubMed.
- J. D. Crowley and P. H. Bandeen, Dalton Trans., 2010, 39, 612 RSC.
- F. Alonso, Y. Moglie, G. Radivoy and M. Yus, Adv. Synth. Catal., 2010, 352, 3208 CrossRef CAS.
- B. Kaboudin, Y. Abedia and T. Yokomatsu, Org. Biomol. Chem., 2012, 10, 4543 CAS.
- A. Megia-Fernandez, M. Ortega-Muñoz, F. Hernandez-Mateo and F. Santoyo-Gonzalez, Adv. Synth. Catal., 2012, 354, 1797 CrossRef CAS.
- R. B. N. Baig and R. S. Varma, Chem. Commun., 2012, 48, 5853 RSC.
- A. N. Prasad, B. Thirupathi, G. Raju, R. Srinivas and B. M. Reddy, Catal. Sci. Technol., 2012, 2, 1264 CAS.
- S. Hwang, H. Bae, S. Kim and S. Kim, Tetrahedron, 2012, 68, 1460 CrossRef CAS.
- J. A. Shin, Y. G. Lim and K. H. Lee, J. Org. Chem., 2012, 77, 4117 CrossRef CAS PubMed.
- M. C. Hashjin, R. Ciyabi, M. Baharloui, G. Hosseini and H. Tavakoli, Chin. J. Chem., 2012, 30, 223 CrossRef.
- M. Driowya, A. Puissant, G. Robert, P. Auberger, R. Benhida and K. Bougrin, Ultrason. Sonochem., 2012, 19, 1132 CrossRef CAS PubMed.
- Y. J. Shang, L. B. Ren and D. M. Wang, Chin. J. Chem., 2007, 25, 1202 CrossRef CAS.
- S. Mohammed, A. K. Padala, B. A. Dar, B. Singh, B. Sreedhar, R. A. Vishwakarma and S. B. Bharate, Tetrahedron, 2012, 68, 8156 CrossRef CAS.
- Z. J. Quan, Q. Xu, Z. Zhang, Y. X. Da and X. C. Wang, Tetrahedron, 2013, 69, 881 CrossRef CAS.
- J. Zhang, G. Jin, S. Xiao, J. Wu and S. Cao, Tetrahedron, 2013, 69, 2352 CrossRef CAS.
- B. T. Ramanjaneyulu, V. Reddy, P. Arde, S. Mahesh and R. Vijaya Anand, Chem.–Asian J., 2013, 8, 1489 CrossRef CAS PubMed.
- B. Dervaux and F. E. Du Prez, Chem. Sci., 2012, 3, 959 RSC.
- A. Megia-Fernandez, M. Ortega-Muñoz, J. Lopez-Jaramillo, F. Hernandez-Mateo and F. Santoyo-Gonzalez, Adv. Synth. Catal., 2010, 352, 3306 CrossRef CAS.
- R. Hudson, C. J. Li and A. Moores, Green Chem., 2012, 14, 622 RSC.
- R. B. Nasir Baig and R. S. Varma, Green Chem., 2012, 14, 625 RSC.
- X. Q. Xiong and L. Cai, Catal. Sci. Technol., 2013, 3, 1301 CAS.
- S. Chassaing, A. S. S. Sido, A. Alix, M. Kumarraja, P. Pale and J. Sommer, Chem.–Eur. J., 2008, 14, 6713 CrossRef CAS PubMed.
- P. Kuhn, A. Alix, M. Kumarraja, B. Louis, P. Pale and J. Sommer, Eur. J. Org. Chem., 2009, 423 CrossRef CAS.
- D. E. Bergbreiter, P. N. Hamilton and N. M. Koshti, J. Am. Chem. Soc., 2007, 129, 10666 CrossRef CAS PubMed.
- C. Girard, E. Onen, M. Aufort, S. Beauviere, E. Samson and J. Herscovici, Org. Lett., 2006, 8, 1689 CrossRef CAS PubMed.
- T. R. Chan and V. V. Fokin, QSAR Comb. Sci., 2007, 26, 1274 CAS.
- L. Bonami, W. Van Camp, D. Van Rijckegem and F. E. Du Prez, Macromol. Rapid Commun., 2009, 30, 34 CrossRef CAS PubMed.
- M. Lammens, J. Skey, S. Wallyn, R. O'Reilly and F. D. Prez, Chem. Commun., 2010, 46, 8719 RSC.
- S. Wallyn, M. Lammens, R. K. O'reilly and F. D. Prez, J. Polym. Sci., Part A: Polym. Chem., 2011, 49, 2878 CrossRef CAS.
- J. Albadi, M. Keshavarz, F. Shirini and M. Vafaie-nezhad, Catal. Commun., 2012, 27, 17 CrossRef CAS.
- E. Ozkal, S. Özçubukçu, C. Jimenoa and M. A. Pericàs, Catal. Sci. Technol., 2012, 2, 195 CAS.
- R. B. Nasir Baig and R. S. Varma, Green Chem., 2013, 15, 1839 RSC.
- S. Roy, T. Chatterjee and Sk. M. Islam, Green Chem., 2013, 15, 2532 RSC.
- T. Shamim and S. Paul, Catal. Lett., 2010, 136, 260 CrossRef CAS.
- H. Sharghi, R. Khalifeh and M. M. Doroodmand, Adv. Synth. Catal., 2009, 351, 207 CrossRef CAS.
- T. Miao and L. Wang, Synthesis, 2008, 363 CAS.
- A. Coelho, P. Diz, O. Caamano and E. Sotelo, Adv. Synth. Catal., 2010, 352, 1179 CrossRef CAS.
- L. Wan and C. Cai, Catal. Lett., 2012, 142, 1134 CrossRef CAS.
- B. H. Lipshutz and B. R. Taft, Angew. Chem., Int. Ed., 2006, 45, 8235 CrossRef CAS PubMed.
- H. Sharghi, M. H. Beyzavi, A. Safavi, M. M. Doroodmand and R. Khalifeha, Adv. Synth. Catal., 2009, 351, 2391 CrossRef CAS.
- B. F. Machadoab and P. Serp, Catal. Sci. Technol., 2012, 2, 54 Search PubMed.
- W. H. Shi, J. X. Zhu, D. H. Sim, Y. Y. Tay, Z. Y. Lu, X. J. Sharma, Y. Zhang, M. Srinivasan, H. Hang, H. H. Hng and Q. Y. Yan, J. Mater. Chem., 2011, 21, 3422 RSC.
- X. Y. Yang, Y. S. Wang, X. Huang, Y. F. Ma, Y. Huang, R. C. Yang, H. Q. Duan and Y. S. Chen, J. Mater. Chem., 2011, 21, 3448 RSC.
- G. G. Zhou, D. W. Wang, F. Li, L. L. Zhang, N. Li, Z. S. Wu, L. Wen, G. Q. Lu and H. M. Cheng, Chem. Mater., 2010, 22, 5306 CrossRef CAS.
- W. Hummers and R. Offeman, J. Am. Chem. Soc., 1958, 80, 1339 CrossRef CAS.
- Y. X. Xu, H. Bai, G. W. Gewu Lu, C. Li and G. Q. Hi, J. Am. Chem. Soc., 2008, 130, 5856 CrossRef CAS PubMed.
- Y. H. Song, Z. F. He, H. Q. Hou, X. L. Wang and L. Wang, Electrochim. Acta, 2012, 71, 58 CrossRef CAS.
- Z. Gonda and Z. Novák, Dalton Trans., 2010, 39, 726 RSC.
- S. Ates, Y. Y. Durmaz, L. Torun and Y. Yagci, J. Macromol. Sci., Part A: Pure Appl.Chem., 2010, 47, 809 CrossRef CAS.
- E. Khosravi, Y. Yagci and Y. Savelyev. New smart materials via metal mediated macromolecular engineering, Springer, 2008, p. 93 Search PubMed.
- J. G. Ha, J. Song, J. K. Lee, B. K. Cho and W. C. Zin, Chem. Commun., 2012, 48, 3418 RSC.
Footnote |
† Electronic supplementary information (ESI) available: The characterization of the products 4–9 by 1H and 13C NMR spectra are given. See DOI: 10.1039/c3ra45994b |
|
This journal is © The Royal Society of Chemistry 2014 |
Click here to see how this site uses Cookies. View our privacy policy here.