DOI:
10.1039/C3RA45837G
(Paper)
RSC Adv., 2014,
4, 11714-11722
Selective “turn-off” fluorescent sensing of mercury ions using aminocyclodextrin:3-hydroxy-N-phenyl-2-naphthamide complex in aqueous solution†
Received
15th October 2013
, Accepted 7th January 2014
First published on 8th January 2014
Abstract
A sensitive and highly selective, fluorescent “turn-off” colorimetric sensor for Hg2+ ions is reported using an aminocyclodextrin:3-hydroxy-N-phenyl-2-naphthamide complex in a 5% CH3CN–water system. Six different aminocyclodextrins viz., per-6-amino-α-cyclodextrin, per-6-amino-β-cyclodextrin, per-6-amino-γ-cyclodextrin, mono-6-amino-α-cyclodextrin, mono-6-amino-β-cyclodextrin and mono-6-amino-γ-cyclodextrin were synthesized and used as hosts for complexing 3-hydroxy-N-phenyl-2-naphthamide. This complex can be used as a sensing system for Hg2+ ions. A blue shifted “turn-off” fluorescence quenching and color change from yellow to colorless in the presence of Hg2+ ions is observed which is attributed to suppression of excited-state intramolecular processes (ESIPT) upon Hg2+ ion complexation. Selectivity towards Hg2+ is found to depend upon cavity size/close proximity of the amino groups of aminocyclodextrins (L1–L6) to the metal ion. Probe L1 is selective and sensitive to Hg2+ with a detection limit as low as 1 × 10−12 M. The present sensor system can also be applied to detect the level of Hg2+ in real environmental water samples.
Introduction
Due to their potential for the easy, highly sensitive and selective detection and quantification of pollutant species, chemical sensors have attracted interest in fields such as waste management, environmental chemistry, clinical toxicology and bioremediation of radionuclides.1,2 Chemical sensors are molecular receptors that transform into analytically useful signals upon binding to specific guests. Over the past few decades, considerable attention has been devoted to the design and synthesis of abiotic receptors for cationic species, which has made cation recognition one of the most popular and fastest growing disciplines in the field of supramolecular chemistry to solve critical analytical problems of social and economical importance.3 Heavy-metal ion pollution is still a global problem due to geological events and the widespread use of heavy-metal ions in modern human activities.4 The detection of heavy metal ions, particularly mercury, lead and cadmium, is critical for environmental monitoring, as these metals are highly toxic and common pollutants. Therefore, developing fluorimetric/colorimetric heavy-metal ion-specific sensors for simple on-site real-time detection with high sensitivity and selectivity is very important and highly desirable.
Mercury is considered as the most toxic nonradioactive metal, and it causes adverse environmental and health problems such as bioaccumulation by numerous organisms and severe physiological problems including neurological, neuromuscular, or nephritic disorders originating from its presence in water resources.5 The Environmental Protection Agency of the USA has already set the upper limit of the Hg2+ ions level in drinking water to 2 ppb (10 nM).6 Among the different forms (including toxic and lipophilic organomercury derivatives typically methylmercury, CH3HgX, X = Cl, AcO−, etc.), Hg2+ ions are not only toxic but also highly water-soluble, making them bioavailable for humans and animals by ingestion of water.7 Therefore, a notable design for new fluorescent mercury sensors with water compatibility as well as with high selectivity and sensitivity is highly essential.5 Thus detecting and monitoring Hg2+ ions in environmental and biological systems presents a challenge.
Supramolecular chemistry is defined as an assembly of molecules bound by multiple weak noncovalent forces8 to form supramolecular structures, which are expected to produce novel functions that differ from those found in simple molecules.9 In this context, cyclodextrins (CDs) are attractive for the construction of supramolecular structures and are macrocyclic oligosaccharides possessing nanosized hydrophobic cavities that bind substrates selectively via noncovalent interactions,8 which enable them to be used in various applications such as catalysis and enzyme mimicry.10 Per-amino-CDs are homogeneous CD derivatives modified by persubstitution at the primary face with amino pendant groups, which display combined hydrophobic and electrostatic binding of guest molecules relative to native CDs. The salient features are (a) the hydrophobic cavity acts as a biomimetic receptor which binds substrates selectively via noncovalent interactions,8 (b) basic primary amino and acidic secondary hydroxyl groups simultaneously act as active/binding sites, which can be utilized in catalysis and to design a sensor, (c) the combination of amino and hydroxyl groups is well known as a (natural) anion/cation binding site for amino acids and peptides,11 and is extensively employed in developing receptors and sensors,12 and (d) it has been noted that the amino groups of amino-CDs as a universal connector for various recognition components and the amino groups as themselves can be recognition sites/efficient first sphere binding ligands for a range of metal ions.13 The presence of a chiral hydrophobic cavity with amino groups on the primary side makes this CD a unique medium for various chemical transformations,14 sensors15 and also form stable complexes with metal cations.16
Our ongoing efforts to realise suitable supramolecular sensing probes for metal ions has prompted us to develop an efficient and highly selective sensor for Hg2+ using aminocyclodextrin (Scheme 1) as the supramolecular host and 3-hydroxy-N-phenyl-2-naphthamide (2) as the fluorescent guest molecule. The latter was chosen as a fluorescent probe due to its desirable structure and unique photophysical properties such as good photostability, long-wavelength absorption and emission.17 Aminocyclodextrins were chosen as hosts due their strong binding ability towards neutral analytes/cations.15 Also, they are a convenient platform to construct colorimetric “naked eye” and/or fluorescence “turn-off” supramolecular probes (L1–L6) taking advantage of the binding sites. The presence of CDs as sensing probes also imparts high water solubility and potential utility in environmental sensing applications.
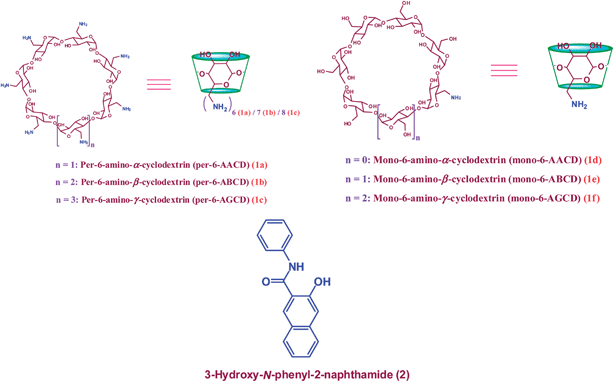 |
| Scheme 1 Aminocyclodextrin (1a–1f) hosts and the probe molecule 2 employed in this study. | |
Results and discussion
Preparation and characterization of probe L1
The fluorescent supramolecular complex probe L1 was prepared using per-6-amino-β-cyclodextrin (per-6-ABCD, 1b)18 as a binding host and 3-hydroxy-N-phenyl-2-naphthamide (2) as a fluorescent guest molecule (Scheme S1 in ESI†) in a 5% CH3CN–water medium and was characterized by UV-vis absorption, fluorescence, NMR, Job's plot and ESI-MS analysis (Fig. S2, S5 and S34 in ESI†). Upon equimolar addition of 1b to 2, the color of the solution turned from colorless to yellow (Fig. S2 in ESI†), the absorption maximum around 359 nm disappeared, three new peaks appeared at 208, 224 and 400 nm and two peaks red shifted from 239 and 300 nm to 255 and 312 nm, respectively, (Fig. 1(i)). Upon complexation with 1b, a new and substantially red-shifted enhanced fluorescence emission was observed at 577 nm (λexc = 400 nm) in aqueous solution (Fig. 1(ii)) in addition to another band at 450 nm. While the peaks at 533 and 577 nm are attributed to ESIPT processes, the peak at 450 nm is due to localized emission. The binding constant value of probe L1 (2166 M−1) (Fig. S4 in ESI†) is calculated by the non-linear curve fit method, and is much higher than that of 2 with the native β-CD (372 M−1). In line with the above results, the color change from colorless to yellow (λmax = 400 nm) is attributed to the formation of a supramolecular complex (probe L1) between 1b and 2. The primary amino groups present in the narrow rim of 1b (pKa 6.5–8.9)18,19a act as an internal base abstracting the proton of the phenolic hydroxyl group of 2 (pKa 6.70 ± 0.40).19b These observations indicate that conjugation increases upon the deprotonation of the phenolic –OH group of 2 inside the cavity of 1b. DFT calculations on 2 and its anionic form indicated that electron transfer takes place from the naphthyl group to the phenyl group by a PET process (Fig. S19 and Schemes S2 and S3 in ESI†).
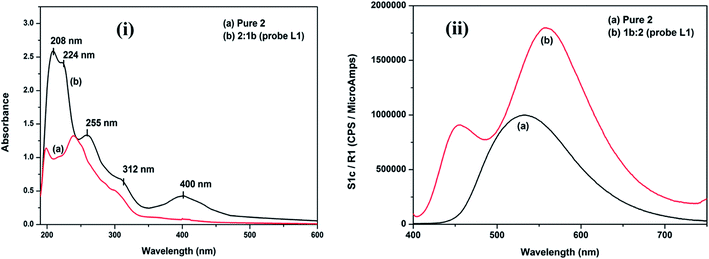 |
| Fig. 1 (i) UV-vis absorption spectra and (ii) fluorescence spectra of 2 (60 μM) (λexc = 360 nm; λemi = 533 nm) with and without per-6-ABCD 1b (60 μM) (λexc = 400 nm; λemi = 577 nm respectively) in the 5% CH3CN–water mixture. | |
The binding of 2 with 1b was also confirmed by NMR titration studies in a D2O + 5% DMSO-d6 mixture. It was found that the phenolic –OH proton signal (10.976 ppm) completely disappeared upon addition of 0.5 and 1.0 equivalents of 1b, whereas the amide NH proton signal broadened and underwent a continuous downfield shift from 10.164 to 10.498 to 10.969 ppm with increasing 1b concentration from 0, 0.5 to 1.0 equivalents (Figs. S7–S9 in ESI†). These observations clearly support a significant hydrogen bonding interaction between 1b and 2 (in probe L1) involving the amide –NH and naphtholate anion. The binding of the naphtholate anion with the amine protons of 1b was difficult to follow as the 1H-NMR signal of the –NH2 protons of 1b merged with the aliphatic/glycosidic hydrogen signals.
These observations also support hydrogen bonding taking place between 2 and 1b involving the amide NH and phenolic O− groups. Active participation of the amino groups in the formation of the supramolecular complex (probe L1) is also supported by the fact that there is negligible change in intensity in the UV-vis absorption and fluorescence emission spectra upon equimolar addition of 2 to β-CD (372 M−1) (Fig. S3(ii) in ESI†). In order to confirm the host–guest ratio between 1b and 2, a Job's plot was also recorded which indicated the formation of a 1
:
1 complex (Fig. S5 in ESI†). The optimized geometry of the complex and molecular modelling pictures were also studied (Figs. S14–S18 in ESI†).
The proposed structure for probe L1 has also found strong support from molecular modeling studies and ESI-MS analysis. In the proposed structure of probe L1, the mode of binding (2) plays a crucial role. This type of inclusion, in which the naphthyl group penetrates inside the CD cavity and the phenyl group of 2 stays outside (narrower rim side) (mode Ia, Fig. S15 in ESI†), has a lower complexation energy (ΔE = −76.92 kcal M−1) and is preferred over the other modes (mode Ib, mode IIa and IIb, Figs. S16–S18 in ESI†), (ΔE = −27.45 kcal M−1, −18.67 kcal M−1 and −44.16 kcal M−1). Mode Ia is stabilized by hydrogen bonding interactions between the ionised phenolic OH (–O−), amide NH group of 2 and amino groups of 1b. Interestingly, the dihedral angle between amide substituent and ionized phenolic OH group is reduced from 34 to 3° upon inclusion with 1b. Further characterization by ESI-MS also confirmed the formation of probe L1 (peak at m/z 1391.72, calcd for C59H91N8O30, [1b:2], probe L1, [M + H]+:1391.58) (Fig. S34 in ESI†).
Sensing response of probe L1 with various metal ions
In order to explore the selective binding of probe L1, UV-vis absorbance and fluorescence titrations were carried out against various mono-, di and trivalent metal ions of biological and ecological importance, viz., Li+, Na+, K+, Ag+, Mg2+, Ca2+, Mn2+, Fe2+, Co2+, Ni2+, Cu2+, Zn2+, Pd2+, Cd2+, Sn2+, Hg2+, Pb2+, Al3+, Cr3+, Fe3+, In3+, Sb3+ and Bi3+ by adding equimolar concentrations of metal ions to a fixed amount of probe L1 (60 μM) in an aqueous acetonitrile solution (Fig. 2). Remarkably, among these ions, only Hg2+ showed quenching of fluorescence with a concomitant blue shift (from 577 to 509 nm), whereas all other ions exhibited relatively little (Cd2+, Cu2+, Fe2+ and Pb2+) or negligible change in emission intensity (Fig. 2(ii)). Meanwhile, the absorption spectrum of probe L1 remained unchanged when various other metal ions such as Li+, Na+, K+, Ag+, Mg2+, Ca2+, Mn2+, Fe2+, Co2+, Ni2+, Cu2+, Zn2+, Pd2+, Cd2+, Sn2+, Pb2+, Al3+, Cr3+, Fe3+, In3+, Sb3+ and Bi3+ were added (Fig. 2(i)). However, upon addition of Hg2+ to probe L1 the colour turned from yellow to colorless with a disappearance of the absorption maxima at 400 nm. Fig. 2(iii) shows the selective binding of probe L1 to Hg2+ in an aqueous acetonitrile solution clearly indicating that the probe L1 selectively binds only with Hg2+ ions, over other metal ions.
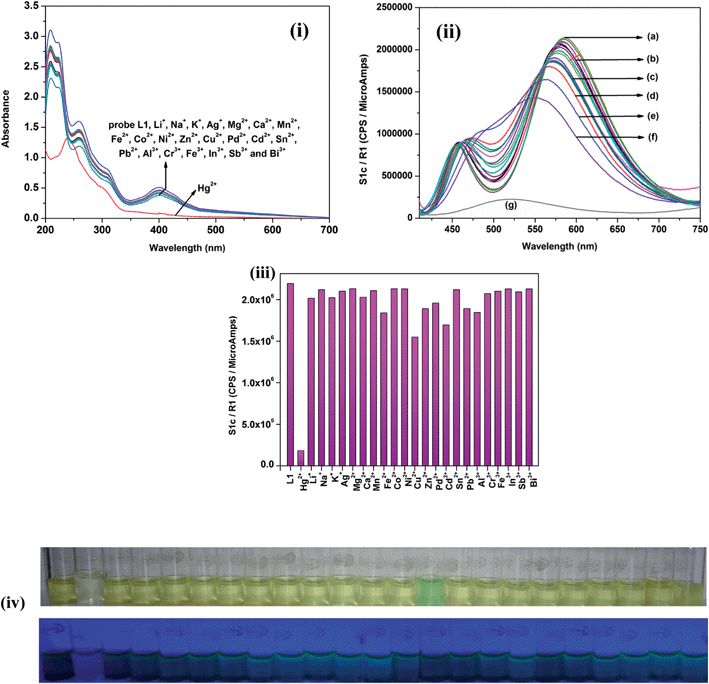 |
| Fig. 2 (i) UV-vis absorption spectra of probe L1 (60 μM) in the absence and presence of chloride salts of various metal cations (60 μM). (ii) Fluorescence spectra of probe L1 (60 μM) in the absence and presence of chloride salts of various metal cations (60 μM); (a) probe L1 only, (b) Li+, Na+, K+, Ag+, Mg2+, Ca2+, Mn2+, Co2+, Ni2+, Pd2+, Sn2+,Cr3+, Fe3+, In3+, Sb3+ and Bi3+ (c) Zn2+, Al3+ and Pb2+ (d) Fe2+ (e) Cd2+ (f) Cu2+ (g) Hg2+ in a 5% CH3CN–water mixture, (λexc = 400 nm; λemi = 577–509 nm; slit 5.0 nm/5.0 nm); (iii) metal ion selectivity of probe L1; (iv) color changes of probe L1 upon addition of various metal cations in daylight and excited by a UV lamp (365 nm) as in the order of probe L1, Hg2+, Li+, Na+, K+, Ag+, Mg2+, Ca2+, Mn2+, Fe2+, Co2+, Ni2+, Zn2+, Cu2+, Pd2+, Cd2+, Sn2+, Hg2+, Pb2+, Al3+, Cr3+, Fe3+, In3+, Sb3+ and Bi3+. | |
Sensing response of probe L1 with Hg2+ ions
In order to prove the selective binding of Hg2+ over other metal ions to probe L1, systematic UV-vis absorption and fluorescence emission titrations were carried out in an aqueous acetonitrile solution. It was found that the absorption spectrum of probe L1 (60 μM) underwent systematic variations when titrated with Hg2+ ions (0–60 μM), and the color of the solution turned from yellow to colorless. As seen in Fig. 3(i), while the absorbance of probe L1 at 313 and 400 nm decreased with increasing Hg2+ concentration, three new peaks appeared at 233, 261 and 341 nm with three distinctive isosbestic points at 364, 325 and 306 nm, respectively, (Fig. S24 in ESI†). Similarly, the emission spectrum of probe L1 (60 μM) underwent fluorescence quenching with a blue-shift (λemi = 577 to 509 nm, Δλemi = 68 nm) when titrated with Hg2+ (Figs. 3(ii) and S25 in ESI†). Beyond equimolar amounts of Hg2+, no significant fluorescence quenching is noticed. A distinguishable color change was observed in the solution of probe L1 (yellow solution), in visible as well as UV light (365 nm), only in the presence of Hg2+ (colorless solution) and not with other ions (Fig. 2(iii)). Thus probe L1 can act as a naked eye as well as fluorescent sensor for Hg2+ ions. The pH of 1b was 8.8 (ref. 19) and its complex with 2 (probe L1) was 8.6 and this value remains unchanged throughout the study. The sensitivity of probe L1 towards Hg2+ ions was found to be constant in the range of 7.5–12 (Fig. S36 in ESI†), which will be utilized in various important practical applications in both environmental and biological analyses.
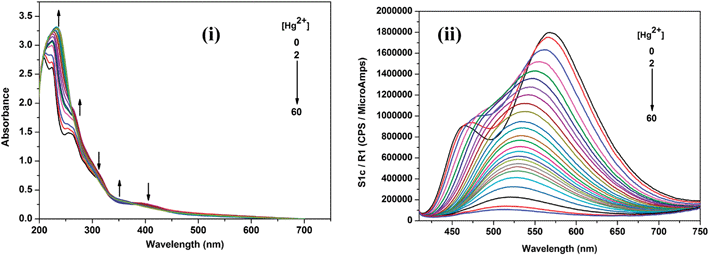 |
| Fig. 3 (i) UV-vis absorption spectra of probe L1 (60 μM) upon titration with Hg2+ (0–60 μM by 2 μM) in a 5% CH3CN–water mixture; (ii) fluorescence spectra of probe L1 (60 μM) upon titration with Hg2+ (0–60 μM by 2 μM step) in a 5% CH3CN–water mixture, (λexc = 400 nm; λemi = 577–509 nm, Δλemi = 68 nm, slit: 5 nm/5 nm). | |
A series of competitive experiments to test the practical applicability and interference of metal ions to the probe L1 for the selective colorimetric and fluorescent detection of Hg2+ ions was also performed. After adding Hg2+ to probe L1 (60 μM) the interfering metal ions viz., Li+, Na+, K+, Ag+, Mg2+, Ca2+, Mn2+, Fe2+, Co2+, Ni2+, Cu2+, Zn2+, Pd2+, Cd2+, Sn2+, Pb2+, Al3+, Cr3+, Fe3+, In3+, Sb3+ and Bi3+ (60 μM) were added and no significant change in the fluorescence behavior was observed, which indicates non-interference (influence) of the added metal ions to the detection of Hg2+ in presence of other metal ions (Fig. 4(i)). Although a very small quenching response with some metal ions (Fe2+, Cu2+, Zn2+, Cd2+, Pb2+ and Al3+) was observed, probe L1 + Hg2+ showed a negligible response with these metal ions. This indicates that probe L1 showed a clear Hg2+ ion dependent quenching behavior. The binding constant of metal ions with probe L1 and 2 were evaluated in an aqueous acetonitrile solution, in which Hg2+ bound strongly with probe L1 (9769 M−2) as compared to 2 (1695 M−1) and 1b (1796 M−1). Interestingly, Hg2+ shows a higher binding constant value with probe L1 than the other metal ions (Table S1 and Fig. S21 in ESI†). This Hg2+-sensitive fluorescence quenching behavior suggests that the probe L1 can be used as a selective sensing probe for the analysis of Hg2+ ions in the presence of other common interfering metal ions.
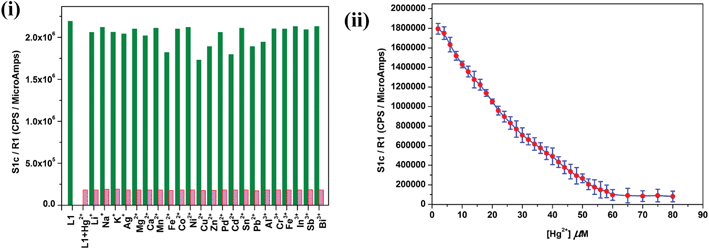 |
| Fig. 4 (i) Fluorescence response in selectivity of probe L1 (60 μM) for Hg2+ in the presence of other metal cations in an aqueous acetonitrile solution. The green bars represent the fluorescence intensity of probe L1 in the presence of one equivalent of the other metal ions. The magenta bars represent the change in fluorescence intensity that occurs upon subsequent addition of one equivalent of Hg2+ ions to the solution containing probe L1 and the other metal ions in the aqueous acetonitrile solution (λexc = 400 nm, λem = 577–509 nm, slit: 5 nm/5 nm); (ii) plot of probe L1 fluorescence intensity change (triplicate) with varied concentrations of Hg2+ from (0 to 60 μM). The error bars represent the standard deviation of the three independent measurements (RSD is 2.1% (n = 3)). | |
The use of probe L1 to sense other mercury salts, such as HgCl2, Hg(OAc)2, Hg(NO3)2 and Hg(ClO4)2 was also explored. Compared to the chlorate salts, probe L1 displayed similar fluorescence changes towards all other mercury species tested (Fig. S26 in ESI†). Zhang et.al., reported a substantially red-shifted fluorescence emission in 3-hydroxy-N-phenyl-2-naphthamide (2) in acetonitrile which was drastically enhanced upon addition of anions such as F−, CH3COO− and H2PO4−, with the enhancement depending on anion basicity.17a Moreover, both the probe L1 and probe L1-Hg2+ complexes showed a negligible response to the anions tested (as TBA salts), including NO3−, CH3COO−, F−, Cl−, CNS−, ClO4−, CO32− and SO42− (Fig. S27 and S28 in ESI†). All these results clearly reveal that probe L1 displayed specific selectivity towards Hg2+ with no interference from other competing ions.
The precision of the method was evaluated by studying the reproducibility. The limit of detection (LOD) for Hg2+ is 1 × 10−12 M with a RSD of 2.1% for the three independent measurements. The corresponding LOD is 1 × 10−12 M (0.2 ppt), which is much less than the maximum level of mercury in drinking water permitted by the United States Environmental Protection Agency (EPA). The sensing response speed of probe L1 for Hg2+ ion was found to be <1 second. As indicated in Fig. 4(ii), the fluorescence quenching (CPS/MicroAmps) of the probe L1 (60 μM) increased upon increasing the concentration of Hg2+. A good linear correlation (R2 = 0.9998) was observed for [Hg2+] ranging from 1 × 10−6 to 60 μM with the value of fluorescence quenching (S1c/R1(CPS/MicroAmps)).
Sensing response of various aminocyclodextrin complexes
In order to obtain good selectivity and sensitivity, a series of five aminocyclodextrin derivatives (Scheme 1) were also synthesized and assayed for sensing response to Hg2+ ions. A series of aminocyclodextrin–fluorophore complexes viz. per-6-amino-α-cyclodextrin (per-6-AACD, 1a):2 (probe L2), per-6-amino-γ-cyclodextrin (per-6-AGCD, 1c):2 (probe L3), mono-6-amino-α-cyclodextrin (mono-6-AACD, 1d):2 (probe L4) mono-6-amino-β-cyclodextrin (mono-6-ABCD, 1e):2 (probe L5) and mono-6-amino-γ-cyclodextrin (mono-6-GACD, 1f):2 (probe L6) were prepared, and assayed towards Hg2+ sensing. Upon complexation of fluorophore 2 with various aminocyclodextrins, its binding and photophysical behaviour were found to vary, depending on the basic nature, close proximity with the amino groups and cavity size of the aminocyclodextrins. Probes L2 and L3 exhibit similar photophysical behaviours compared to the other prepared probes. Complexation of fluorophore 2 with the mono-aminocyclodextrins (1d, 1e and 1f) shows similar photophysical behaviour with lower binding constant values (1921, 2896 and 3325 M−1, respectively) (Table S2 in ESI†). On the other hand, the peraminocyclodextrins (1a, 1b and 1c) bind with fluorophore 2 and show higher binding constant values (7347, 9769 and 10
692 M−1, which may ensure a stronger binding and deeper inclusion into the CD cavity) compared to the monoaminocyclodextrins.
Among the various prepared probes (L2–L6) titrated against various metal ions, probe L2 showed good selectivity towards Hg2+ compared to the other metal ions and a sensitivity as low as 2 × 10−10 M. However, probe L3 showed a lower sensitivity than probe L2 because of its larger cavity size (Figs. S29 and S30 in ESI†). On the other hand, probes L4–L6 showed a negligible response towards Hg2+ (Figs. S31–S33 in ESI†) (containing both amino and hydroxyl groups). These results clearly indicate that selectivity towards Hg2+ is found to depend upon cavity size/close proximity of amino groups of aminocyclodextrins, and the cooperative and complementary binding between probe (L1–L6) and the metal ion.
Proposed mechanism of Hg2+ ion sensing
A plausible mechanism based on the ESIPT process is proposed for the “turn-off” sensing of Hg2+ using probe L1 as given in Scheme 2. Probe L1 is formed through the in situ addition of 2 to 1b and the resulting solution is yellow in color (λmax = 400 nm) with a high fluorescence intensity (λexc = 400 nm, λemi = 577 nm). The yellow color is due to ionization of the –OH group by abstraction of a proton by the amino groups of 1b. This undergoes an ESIPT process in which the naphthoxide ion abstracts the proton from the –NH group resulting in a localized emission as well as ESIPT emission (Schemes S2 and S3 in ESI†). Upon addition of Hg2+, the absorption maximum at 400 nm disappeared and a new peak appeared at 360 nm with a marked color change, from yellow to colorless (Fig. S24 in ESI†). Further, a selective fluorescence quenching with a blue-shift from 577 to 509 nm (Δλemi = 68 nm) is observed in the emission titration (Fig. S25 in ESI†). Upon addition, Hg2+ binds simultaneously to the –O− and amide –NH group of 2 and the simultaneous coordination of the carbonyl group with the amino groups of 1b suppresses the ESIPT process.
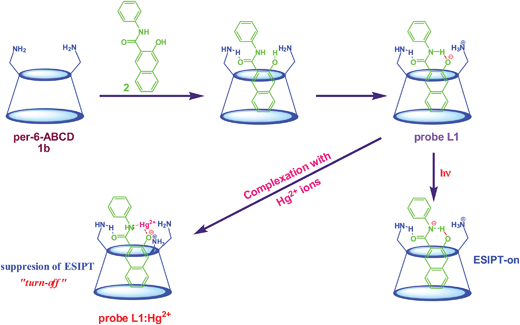 |
| Scheme 2 Proposed mechanism of Hg2+ ion sensing by per-6-ABCD:2 complex (probe L1) in a 5% CH3CN–water mixture. | |
In order to explore the ESIPT suppression process, fluorescent quantum yields (Φ) of the probe L1 (ΦL1) and probe L1–Hg2+ complex (ΦL1:Hg) were compared, which were calculated using the fluorescent quantum yield of fluorescein as a reference compound in 0.1 N NaOH solution (ΦF = 0.79%). It was found that the ΦL1 decreased from 0.72% to 0.08% (ΦL1:Hg) upon equimolar addition of Hg2+. These observations clearly confirm the suppression of the ESIPT process upon the binding of probe L1 to Hg2+.
The lifetimes (in ns) and the relative amplitudes of the different species are given in Table 1 (Fig. S37 in ESI†). When excited at 360 nm, fluorophore 2 shows two lifetime components, the structures of which are given in Scheme S3† (an intramolecularly H-bonded form I (τ2 = 4.105 ns; RA = 90.15) and a non H-bonded form II (τ1 = 0.932 ns; RA = 9.85)). Upon complexation with 1b (probe L1), the existence of two species is noticed, one in the free state (τ2 = 2.224 ns; RA = 15.49) and the other in the complexed state with a longer lifetime (τ1 = 5.434 ns; RA = 84.51). Upon addition of Hg2+ (from 0.1 equivalent to 1.1 equivalents), the fluorescence lifetime τ1 decreases with a concomitant increase in RA. However, τ2 remains relatively unchanged, with a gradual decrease in RA. These observations strongly support the proposed mechanism.
Table 1 Lifetime data and relative amplitudes for probe L1 and its components 2 and 1b and probe L1 titration with Hg2+ in a 5% CH3CN–water mixturea
Substrate |
λexc (nm) |
λemi (nm) |
Lifetime τ (ns) |
Relative amplitude |
χ2 |
τ1 |
τ2 |
RA1 |
RA2 |
[Probe L1] = 60 μM; [Hg2+] = 0–60 μM. |
2 |
360 |
533 |
0.932 |
4.105 |
9.85 |
90.15 |
1.0057 |
Probe L1 (1 : 1, 1b + 2) |
400 |
577 |
5.434 |
2.224 |
84.51 |
15.49 |
1.0658 |
L1 + 0.1 equi. of Hg2+ |
400 |
577 |
4.768 |
2.455 |
85.03 |
14.97 |
1.0049 |
L1 + 0.2 equi. of Hg2+ |
400 |
577 |
3.827 |
2.637 |
85.83 |
14.17 |
1.0806 |
L1 + 0.4 equi. of Hg2+ |
400 |
577 |
2.591 |
2.801 |
83.11 |
16.89 |
1.0555 |
L1 + 0.6 equi. of Hg2+ |
400 |
577 |
2.407 |
3.141 |
89.59 |
10.41 |
1.1471 |
L1 + 0.8 equi. of Hg2+ |
400 |
577 |
1.899 |
2.346 |
88.02 |
11.98 |
1.1075 |
L1 + 1.0 equi. of Hg2+ |
400 |
577 |
1.744 |
2.540 |
86.68 |
13.32 |
1.0812 |
L1 + 1.1 equi. of Hg2+ |
400 |
577 |
1.042 |
2.580 |
92.54 |
07.46 |
1.1428 |
To confirm the presence and need for seven amino groups in 1b for efficient binding of Hg2+, control experiments were carried out with/without the native β-CD:2 complex in the absence/presence of an external base, ethylenediamine and basic buffer (NH3/NH4Cl buffer, pH = 9.4). Upon addition of Hg2+ to the above experiments, only a very small amount of fluorescence quenching was observed. A control experiment was carried out with 1b:adamantane/2 complex (binding constant 8562 M−1) titrated against Hg2+ which showed no significant response in the emission intensity up to an equimolar concentration level of Hg2+.
The proposed mechanism also finds strong support from the binding constant values, Job's plot and molecular modeling studies. The binding constants of Hg2+ ion with probe L1 were calculated. The values show that the binding constant of Hg2+ to probe L1 (9769 M−2) is greater than its binding to 2 (1695 M−1) and 1b (1796 M−1). It is relevant to note that these values are the highest among the various metal ions. The H
:
G ratio of the Hg2+ ion with probe L1 shows the formation of 1
:
1 H
:
G complexes. Formation of the binary and then ternary complexes is also evident from ESI-MS. The molecular ion peaks (m/z found 1591.64, calcd for C59H89HgN8O30, [1b:2:Hg2+], [M − H]+: 1591.54; m/z found 1391.72, calcd for C59H91N8O30, [1b:2], and [M + H]+:1391.58) correspond to a ternary complex of probe L1 with Hg2+ and a binary complex of 1b and 2, respectively (Figs. S35 and S34 in ESI†).
Finally, the binding of Hg2+ with probe L1 and its components 2 and 1b was also confirmed by carbon NMR titration studies. These studies indicate that a negligible change in chemical shift values was observed in the 13C NMR spectra of the components 2 and 1b upon addition of 1.0 equivalent of Hg2+ ions (Figs. S10 and S11 in ESI†). However, in the 13C-NMR spectra of probe L1, significant deshielding is observed in the chemical shift values of the amide C
O (from 170.065 ppm to 173.403 ppm), C1′ aryl carbon attached to amide NH group (from 137.686 ppm to 138.636 ppm) and C3-carbon attached to –O− group in naphtholate anion (from 160.064 ppm to 163.132 ppm) (Figs. S12 and S13 in ESI†). This clearly indicates that Hg2+ ion complexation takes place with the amide –NH as well as naphtholate anion, which is responsible for its detection reported in the present study.
Thus the present study clearly establishes a more selective sensing of Hg2+ over other metal ions using a supramolecular complex of per-6-amino-β-cyclodextrin:3-hydroxy-N-phenyl-2-naphthamide (probe L1) in an aqueous acetonitrile solution. This selectivity and sensitivity are attributed to the stronger binding of Hg2+ to the amide NH and phenolic O− groups of 2 and simultaneous co-ordination of C
O group to the amino groups of 1b which makes them responsible for naked-eye as well as “turn-off” fluorescence sensing towards Hg2+ ions.
Real time monitoring study
To investigate further the potential utility and practical applicability of the sensing probe L1 to real samples, the levels of Hg2+ ions in five practical water samples such as drinking water, tap water, bore well water and two river waters were examined. All collected water samples were filtered three times through qualitative filter paper before use and the initial levels of Hg2+ ions were examined. The standard addition method was used to determine the concentration of Hg2+ by the addition of 30, 60 and 90 μM of Hg2+ to the above water samples. The observed results listed in Table 2 show that probe L1 is able to measure the concentrations of spiked Hg2+ ions with good recovery. These results clearly indicate that probe L1 can be potentially employed for Hg2+ detection in natural water samples.
Table 2 Analytical results for the detection of Hg2+ in five practical samples
Sample |
Determined [Hg2+]a (μM) |
Added [Hg2+] (μM) |
Found total [Hg2+]a (μM) |
Recovery (%) |
Mean ± standard deviation (n = 3). |
Drinking water |
0 |
30 |
29 ± 0.8 |
96.7 |
60 |
59 ± 1.2 |
98.3 |
90 |
90 ± 0.7 |
100.0 |
Tap water |
0 |
30 |
28 ± 1.3 |
93.3 |
60 |
60 ± 0.9 |
100.0 |
90 |
92 ± 1.6 |
102.2 |
Bore well water |
0 |
30 |
31 ± 0.9 |
103.3 |
60 |
58 ± 2.1 |
96.7 |
90 |
88 ± 1.4 |
97.8 |
River water # 1 |
0 |
30 |
31 ± 1.5 |
103.3 |
60 |
59 ± 1.2 |
98.3 |
90 |
91 ± 0.7 |
101.1 |
River water # 2 |
0 |
30 |
28 ± 2.3 |
93.3 |
60 |
57 ± 1.2 |
95.0 |
90 |
92 ± 1.8 |
102.2 |
Conclusions
In conclusion, the supramolecular probe L1 has been successfully employed as a naked-eye and “turn-off” fluorogenic sensor to detect environmentally hazardous Hg2+ ions with high selectivity and sensitivity in an aqueous solution medium and with a fast response time. The biocompatibility of this sensing system is also evident with per-6-amino-β-cyclodextrin as a supramolecular host and the 3-hydroxy-N-phenyl-2-naphthamide as the highly sensitive signal transductor with its unique photophysical properties. The blue shifted “on–off” fluorescence quenching and color change from yellow to colorless by the addition of Hg2+ is explained via suppression of the excited-state intramolecular proton transfer process (ESIPT) with a detection limit as low as 1 × 10−12 M of Hg2+ ions. The present sensor can also be applied to detect the level of Hg2+ ion in real water samples.
Acknowledgements
The authors gratefully acknowledge financial assistance from University Grants Commission-Basic Science Research and Department of Science and Technology, New Delhi, India. K. K. thanks the Council of Scientific and Industrial Research, New Delhi for the award of Senior Research Fellowship. Authors also gratefully acknowledge the National Centre for Ultrafast Processes (NCUFP), University of Madras, Taramani Campus, Chennai for lifetime measurement study.
References
-
(a) G. K. Walkup and B. Imperiali, J. Am. Chem. Soc., 1996, 118, 3053 CrossRef CAS;
(b) A. Miyawaki, J. Llopis, R. Helm, J. M. McCaffery, J. A. Adams, M. Ikura and R. Y. Tsien, Nature, 1997, 388, 882 CrossRef CAS PubMed;
(c) M. M. Henary and C. J. Fahrni, J. Phys. Chem. A, 2002, 106, 5210 CrossRef CAS;
(d) J. Homola, S. S. Yee and G. Gauglitz, Sens. Actuators, B, 1999, 54, 3 CrossRef CAS;
(e) F. Turner, Science, 2000, 290, 1315 CrossRef;
(f) P. Chen and C. He, J. Am. Chem. Soc., 2004, 126, 728 CrossRef CAS PubMed.
-
(a) S. J. Lee, J.-E. Lee, J. Seo, I. Y. Jeong, S. S. Lee and J. H. Jung, Adv. Funct. Mater., 2007, 17, 3441 CrossRef CAS;
(b) S. J. Lee, S. S. Lee, J. Y. Lee and J. H. Jung, Chem. Mater., 2006, 18, 4713 CrossRef CAS;
(c) E. Palomares, R. Vilar, A. Green and J. R. Durrant, Adv. Funct. Mater., 2004, 14, 111 CrossRef CAS.
- J. W. Steed and J. L. Atwood (King's College, London) (University of Missouri, Columbia), Supra-molecular Chemistry, John Wiley & Sons Ltd., Chichester, England, 2000, ISBN 0-471-98831-6 (hardback), ISBN 0-471-98791-3 (paperback, 745 pp, GBP 29-95) Search PubMed.
-
(a) M. Nendza, T. Herbst, C. Kussatz and A. Gies, Chemosphere, 1997, 35, 1875 CrossRef CAS;
(b) D. W. Boening, Chemosphere, 2000, 40, 1335 CrossRef CAS.
-
(a) E. M. Nolan and S. J. Lippard, Chem. Rev., 2008, 108, 3443 CrossRef CAS PubMed;
(b) H. N. Kim, M. H. Lee, H. J. Kim, J. S. Kim and J. Yoon, Chem. Soc. Rev., 2008, 37, 1465 RSC;
(c) H. N. Kim, W. X. Ren, J. S. Kim and J. Yoon, Chem. Soc. Rev., 2012, 41, 3210 RSC;
(d) A. P. de Silva, T. S. Moody and G. D. Wright, Analyst, 2009, 134, 2385 RSC.
- Mercury Update: Impact of Fish Advisories. EPA Fact Sheet EPA-823-F-01–011; EPA, Office of Water: Washington, DC, 2001.
-
(a) S. M. Ullrich, T. W. Tanton and S. A. Abdrashitova, Crit. Rev. Environ. Sci. Technol., 2001, 31, 241 CrossRef CAS;
(b) P. B. Tchounwou, W. K. Ayensu, N. Ninashvili and D. Sutton, Environ. Toxicol., 2003, 18, 149 CrossRef CAS PubMed;
(c) T. W. Clarkson and L. Magos, Crit. Rev. Environ. Sci. Technol., 2006, 36, 609 CAS;
(d) J. S. Kuwabara, Y. Arai, B. R. Topping, I. J. Pickering and G. N. George, Environ. Sci. Technol., 2007, 41, 2745 CrossRef CAS;
(e) J. Jiang, W. Liu, J. Cheng, L. Yang, H. Jiang, D. Bai and W. Liu, Chem. Commun., 2012, 48, 8371 RSC.
-
(a) J.-M. Lehn, Supramolecular Chemistry: Concepts and Perspectives, VCH, Weinheim, 1995 Search PubMed;
(b) A. Yamauchi, T. Hayashita, A. Kato, S. Nishizawa, M. Watanabe and N. Teramae, Anal. Chem., 2000, 72, 5841 CrossRef CAS;
(c) K. Takahashi, Chem. Rev., 1998, 98, 2013 CrossRef CAS PubMed;
(d) H. Sakuraba and H. Maekawa, J. Inclusion Phenom. Macrocyclic Chem., 2006, 54, 41 CrossRef CAS;
(e) S. V. Bhosale and S. V. Bhosale, Mini-Rev. Org. Chem., 2007, 4, 231 CrossRef CAS.
- D. S. Lawrence, T. Jiang and M. Levett, Chem. Rev., 1995, 95, 2229 CrossRef CAS.
-
(a) K. Harano, H. Kiyonaga and T. Hissano, Tetrahedron Lett., 1991, 32, 7557 CrossRef CAS;
(b) K. Surendra, N. S. Krishnaveni, R. Sridhar and K. R. Rao, Tetrahedron Lett., 2006, 47, 2125 CrossRef CAS PubMed.
-
(a) H. Luecke and F. A. Quiocho, Nature, 1990, 347, 402 CrossRef CAS PubMed;
(b) J. J. He and F. A. Quiocho, Science, 1991, 251, 1479 CAS.
-
(a) K. Kavallieratos, S. R. de Gala, D. J. Austin and R. H. Crabtree, J. Am. Chem. Soc., 1997, 119, 2325 CrossRef CAS;
(b) M. J. Deetz and B. D. Smith, Tetrahedron Lett., 1998, 39, 6841 CrossRef CAS;
(c) K. Kavallieratos, C. M. Bertao and R. H. Crabtree, J. Org. Chem., 1999, 64, 1675 CrossRef CAS PubMed;
(d) S.-S. Sun and A. J. Lees, Chem. Commun., 2000, 1687 RSC;
(e) K. Kavallieratos, R. A. Sachleben, G. J. V. Berkel and B. A. Moyer, Chem. Commun., 2000, 187 RSC;
(f) K. Choi and A. D. Hamilton, J. Am. Chem. Soc., 2001, 123, 2456 CrossRef CAS;
(g) H. Miyaji and J. L. Sessler, Angew. Chem., Int. Ed., 2001, 40, 154 CrossRef CAS;
(h) S. Kondo, T. Suzuki and Y. Yano, Tetrahedron Lett., 2002, 43, 7059 CrossRef CAS;
(i) C. P. Causey and W. E. Allen, J. Org. Chem., 2002, 67, 5963 CrossRef CAS PubMed;
(j) P. Piatek and J. Jurczak, Chem. Commun., 2002, 2450 RSC;
(k) S. J. Coles, J. G. Frey, P. A. Gale, M. B. Hursthouse, M. E. Light, K. Navakhun and G. L. Thomas, Chem. Commun., 2003, 568 RSC;
(l) S. Camiolo, P. A. Gale, M. B. Hursthouse, M. E. Light and C. N. Warriner, Tetrahedron Lett., 2003, 44, 1367 CrossRef CAS;
(m) B. Tomapatanaget, T. Tuntulani and O. Chailapakul, Org. Lett., 2003, 5, 1539 CrossRef CAS PubMed.
-
(a) X.-J. Zhu, S.-T. Fu, W.-K. Wong, J.-P. Guo and W.-Y. Wong, Angew. Chem., Int. Ed., 2006, 45, 3150 CrossRef CAS PubMed;
(b) Z. Xu, K.-H. Baek, H. N. Kim, J. Cui, X. Qian, D. R. Spring, I. Shin and J. Yoon, J. Am. Chem. Soc., 2010, 132, 601 CrossRef CAS PubMed;
(c) H. Yuasa, N. Miyagawa, T. Lzumi, M. Nakatani, M. Lzumi and H. Hashimoto, Org. Lett., 2004, 6, 1489 CrossRef CAS PubMed.
-
(a) K. Kanagaraj and K. Pitchumani, Chem.–Eur. J., 2013, 19, 14425 CrossRef CAS PubMed;
(b) K. Kanagaraj and K. Pitchumani, J. Org. Chem., 2013, 78, 744 CrossRef CAS PubMed;
(c) I. A. Azath, P. Puthiaraj and K. Pitchumani, ACS Sustainable Chem. Eng., 2013, 1, 174 CAS;
(d) I. A. Azath, P. Suresh and K. Pitchumani, New J. Chem., 2012, 36, 2334 RSC;
(e) K. Kanagaraj, P. Suresh and K. Pitchumani, Org. Lett., 2010, 12, 4070 CrossRef CAS PubMed;
(f) K. Kanagaraj and K. Pitchumani, Tetrahedron Lett., 2010, 51, 3312 CrossRef CAS PubMed;
(g) P. Suresh and K. Pitchumani, J. Org. Chem., 2008, 73, 9121 CrossRef CAS PubMed;
(h) P. Suresh and K. Pitchumani, Tetrahedron: Asymmetry, 2008, 19, 2037 CrossRef CAS PubMed.
-
(a) I. A. Azath and K. Pitchumani, Sens. Actuators, B, 2013, 188, 59 CrossRef CAS PubMed;
(b) K. Kanagaraj, A. Affrose, S. Sivakolunthu and K. Pitchumani, Biosens. Bioelectron., 2012, 35, 452 CrossRef CAS PubMed;
(c) I. A. Azath, P. Suresh and K. Pitchumani, Sens. Actuators, B, 2011, 155, 909 CrossRef CAS PubMed;
(d) P. Suresh, I. A. Azath and K. Pitchumani, Sens. Actuators, B, 2010, 146, 273 CrossRef CAS PubMed.
-
(a) I. Tabushi, N. Shimizu, T. Sugimoto, M. Shiozuka and K. Yamamura, J. Am. Chem. Soc., 1977, 99, 7100 CrossRef CAS;
(b) C. A. Haskard, C. J. Easton, B. L. May and S. F. Lincoln, Inorg. Chem., 1996, 35, 1059 CrossRef CAS PubMed;
(c) M. A. Mortellaro and D. G. Nocera, J. Am. Chem. Soc., 1996, 118, 7414 CrossRef CAS.
-
(a) X. Zhang, L. Guo, F.-Y. Wu and Y.-B. Jiang, Org. Lett., 2003, 5, 2667 CrossRef CAS PubMed;
(b) G. Lu, J. E. Grossman and J. B. Lambert, J. Org. Chem., 2006, 71, 1769 CrossRef CAS PubMed.
- P. R. Ashton, R. Koniger, J. F. Stoddart, D. Alker and V. D. Harding, J. Org. Chem., 1996, 61, 903 CrossRef CAS.
-
(a) B. Hamelin, L. Jullien, F. Guillo, J.-M. Lehn, A. Jardy, L. De Robertis and H. Driguez, J. Phys. Chem., 1995, 99, 17877 CrossRef CAS;
(b) Calculated using Advanced Chemistry Development(ACD/Labs) Software V11.02 (© 1994-2012 ACD/Labs) Search PubMed.
Footnote |
† Electronic supplementary information (ESI) available. See DOI: 10.1039/c3ra45837g |
|
This journal is © The Royal Society of Chemistry 2014 |