DOI:
10.1039/C3RA44059A
(Paper)
RSC Adv., 2014,
4, 467-473
A self-assembled net structured film for the immobilization of tris(2,2′-bipyridyl)ruthenium(II) and its ultrasensitive electrogenerated chemiluminescent sensing for phenol†
Received
1st August 2013
, Accepted 1st October 2013
First published on
2nd October 2013
Abstract
A self-assembled net structure to immobilize tris(2,2′-bipyridyl)ruthenium(II) ditetrakis(4-chlorophenyl)borate (RuDB) onto a gold surface is presented here for the first time. The formation of the net structure involved alternately depositing 1,10-decanedithiol (DDT) and gold nanoparticles (AuNPs) on the gold surface. The RuDB molecules became distributed in the spaces between the long chains of DDT and were successfully immobilized in the formed net structure. Repeating the modification process fabricated a much larger net structure incorporating more RuDB. The fabrication process is very simple, effective and low cost. An electrogenerated chemiluminescence (ECL) study confirmed that RuDB immobilized in the net structure was considerably stable and exhibited excellent ECL behavior. Moreover, the ECL signal of the proposed sensor was sensitively inhibited by phenol and exhibited a low detection limit of 0.6 nM with a signal-to-noise ratio of 3. Consequently, the design of the net structure for immobilizing probe molecules may be promising for other sensing applications.
Introduction
Electrogenerated chemiluminescence (ECL), in recent decades, has become one of the most important and valuable detection methods in the analytical chemistry field.1–3 Immobilization of ECL probes on the electrode surface could simplify the experimental instrument, reduce the consumption of expensive reagents, and fabricate regenerable solid-state ECL sensors. Till now, plenty of approaches for the effective immobilization of tris(2,2′-bipyridyl)ruthenium(II) (Ru(bpy)32+), one of the most extensively studied ECL probes, have been proposed.4 These include the direct attachment of Ru(bpy)32+ to an electrode surface using the Langmuir–Blodgett technique5 or a self-assembly method,6 incorporation of Ru(bpy)32+ into the ionomer Nafion or Nafion-derived films,7 and the sol–gel method.8 Additionally, the use of nanomaterials to obtain signal enhancement has been one of the most interesting research fields in the development of the solid-state ECL technique.9–11 Various nanomaterials are becoming increasingly popular to organize the ECL probes.12–14 Nanoparticles, such as Au,15,16 Ag,17 Pt,18 TiO2,19 SiO2,20 and ZrO2,21 have been used to make Ru(bpy)32+–ECL sensors exhibiting excellent performances since they increase the active area and improve the electrochemical properties of the interface.
With the purpose of effectively immobilizing Ru(bpy)32+ on the electrode surface and obtaining signal enhancement, a simple but effective method based on a self-assembled net structure consisting of Au–S bonds is presented here for the first time. In previous reports, it has been clearly demonstrated that there are strong interactions between Au atoms and S atoms,22 and multilayer gold nanoparticles (AuNPs) can be formed on an electrode surface in the presence of a dithiol linker molecule.23,24 Moreover, it has been reported that favorable electrochemistry was observed with electrode–SAM–metallic nanoparticles systems.25,26 Considering the above properties, we employed 1,10-decanedithiol (DDT) and AuNPs to fabricate a net structure on a gold electrode surface which was used to effectively immobilize hydrophobic RuDB (Scheme 1), formed from water-soluble Ru(bpy)3Cl2 through a simple double decomposition reaction. Since DDT with two sulfhydryl groups can link to an Au atom at each end, a net structure based on Au–S bonds could be fabricated when DDT and AuNPs were alternately deposited on the gold electrode surface. During the self-assembly process, the luminophore RuDB molecules became distributed in the spaces between the long chains of DDT and were successfully immobilized in the formed net structure. The resultant solid-state Ru(bpy)32+–ECL sensor was studied and exhibited excellent ECL behavior. It can be concluded from the results that the design of the net structure led to a low consumption of RuDB, and additionally it simplified the assembly operation, enhanced the ECL intensity and increased the sensitivity of the sensor in the presence of AuNPs.
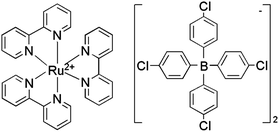 |
| Scheme 1 The structural formula of the new complex RuDB. | |
In ECL analysis, numerous analytes are detected based on their inhibition or enhancement properties. The inhibition effect of phenols on the Ru(bpy)32+ ECL signal was reported previously.27,28 It has been demonstrated that numerous compounds, including phenols, are detectable with the detection limits in the range of 10−8 to 10−9 mol L−1 for a Ru(bpy)32+–TPrA system.27 In our investigation, it was observed that the ECL intensity of the present sensor decreased with the addition of phenol and was linearly proportional to the concentration of phenol. The sensor also exhibited good stability and a low detection limit of 0.6 nM with a signal-to-noise ratio of 3. Owing to its high stability, effectiveness and low cost, the design of the self-assembled net structured film opens up a new way to expand other sensing applications.
Experimental
Chemicals and apparatus
Tris(2,2′-bipyridyl)ruthenium(II) dichloride hexahydrate (Ru(bpy)3Cl2·6H2O, 99%) and potassium tetrakis(4-chlorophenyl)borate (K[4-(Clph)4B], 98%) were purchased from Fluka Chemical Corp. (Ronkondoma, NY). Tri-n-propyl amine (TPrA, 98%) and 1,10-decanedithiol (DDT, 95%) were supplied by Alfa Aesar. The gold colloids with a mean size of 10 nm in diameter and potassium ferricyanide were obtained from Sigma-Aldrich and Chengdu Chemicals (Sichuan, China), respectively. A phosphate-buffered saline (PBS) solution was prepared by neutralizing a 0.2 M NaH2PO4 solution with concentrated NaOH solution. Other chemicals were of analytical grade and were used as received without further purification. Doubly distilled water was used throughout the whole experiment.
The Gaussian 03 program29 was utilized to estimate the length of the DDT molecules linked with Au atoms. ECL measurements were carried out with a Remax MPI-E ECL instrument (Xi'an Remax Electronic Science Tech. Co. Ltd., Xi'an, China) using a conventional three-electrode system. A gold electrode was employed as the working electrode, with an Ag/AgCl electrode (saturated KCl) and a platinum wire as the reference and counter electrodes, respectively. The cyclic voltammetry (CV) and electrochemical impedance spectroscopy (EIS) were performed with an Autolab electrochemical workstation (PGSTAT 30/302, Eco-Chemie, The Netherlands B.V.), using another conventional three-electrode system. A gold electrode was used as the working electrode, with a saturated calomel electrode (SCE) as the reference electrode and a Pt wire as the counter electrode. The mass spectra were determined with a TSQ Quantum Ultra mass spectrometer (Thermo Fisher Scientific, San Jose, CA). A S-4800 scanning electron microscope (SEM) (Hitachi, Tokyo, Japan) operating at 20 kV and an atomic force microscope (AFM) (SHIMADZU SPM-9600) were used to investigate the morphology of the modified gold substrate. X-ray photoelectron spectroscopy (XPS) analysis was performed on a photoelectron spectroscopy instrument (Kratos, XSAM 800).
Synthesis of RuDB
The synthesis of RuDB involved a double decomposition reaction in which water-soluble Ru(bpy)3Cl2 was transformed into hydrophobic RuDB. The equation for this double decomposition reaction is as follows:
2K[4-(Clph)4B] + Ru(bpy)3Cl2 → [Ru(bpy)3][(4-(Clph)4B)]2 + 2KCl |
The detailed synthetic procedure is described in the ESI,† and the molecular weight and structure of RuDB were fully verified by mass spectrometry as shown in Fig. S1, ESI.†
Preparation of the self-assembled layers of DDT/Ru–AuNPs on the gold electrode surface
The gold electrode was polished carefully with 1.0, 0.3, and 0.05 μm Al2O3 powders using a fine polishing cloth to obtain a mirror surface, and then ultrasonically cleaned with doubly distilled water and acetone for 5 min respectively. Prior to modification, the bare gold electrode was scanned between −0.2 and 1.6 V in 0.5 M H2SO4 solution until a reproducible cyclic voltammogram (CV) was obtained. After a thorough rinsing with doubly distilled water, the electrode was immersed in a solution containing 0.5 μL TPrA, 100 μL of an acetone solution of RuDB (0.5 g L−1) and 1.0 mL of an absolute ethanol solution of DDT (2.5 mM) for about 90 min. Subsequently, the gold electrode was transferred into 1 mL of colloidal AuNPs (10 nm in diameter) for about 30 min at 4 °C and then the DDT/Ru–AuNPs monolayer was fabricated. The above modification process could be repeated until the desired number of DDT/Ru–AuNPs layers had been attached onto the gold substrate.
Results and discussion
Fabrication of the DDT/Ru–AuNPs net structured film on the gold electrode surface
When a bare gold electrode was immersed into a solution containing DDT and RuDB, DDT with its excellent film forming properties assembled onto the gold surface because of strong Au–S interactions, and a sulfhydryl-derivated surface was consequently obtained.22 Simultaneously, the coverage of DDT on the gold electrode surface was influenced by nearby RuDB molecules, and some RuDB molecules became distributed in the spaces between the long chains of DDT. Then the available sulfhydryl group on the other end of each DDT molecule linked with another Au atom when the as-prepared electrode was treated with AuNPs. As a result, the RuDB molecules in the spaces between the long chains of DDT were successfully immobilized in the formed net structure. Eventually, a larger net structure inlaid with numerous RuDB molecules was successfully fabricated on the gold electrode surface through repeating the modification process. A schematic illustration of the stepwise self-assembly procedure to form DDT/Ru–AuNPs double layer on the gold electrode surface is shown in Scheme 2. The spatial confinement of the net structure results in the effective immobilization of RuDB on the gold surface.
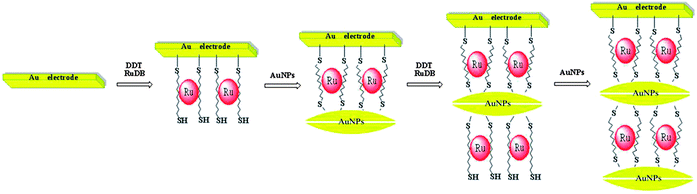 |
| Scheme 2 The self-assembly steps to form DDT/Ru–AuNPs double layer on a gold electrode surface (for convenience when drawing the second DDT/Ru–AuNPs layer, only the two opposite interfaces of the spherical AuNPs are depicted). | |
Computations for studying the feasibility of immobilizing RuDB in the net structure
In order to confirm the theoretical feasibility of immobilizing RuDB in the net structure, the Gaussian 03 program29 was utilized to compare the dimensions of the RuDB molecule with the length of the DDT molecule in an approximate situation, since the real situation involves a fixed gold substrate which is computationally relatively complex. A unimolecular model which consisted of a DDT molecule linked to an Au atom at the ends was designed to estimate the length of the DDT molecule in the real situation. In the investigation, the designed unimolecular model was optimized using the B3LYP density functional in combination with the 6-311++g(d,p) basis set on C, H and S, and the LANL2DZ basis set on Au. In the assumed situation, the optimal result from the Gaussian 03 system showed that the length of the DDT molecule bonded to two Au atoms was about 18.10 Å, while the dimensions of Ru(bpy)32+ (a = b = 10.76 Å, c = 16.39 Å) have been reported previously30 and the anion (4-Clph)4B− is well known to have smaller dimensions. Thus, the bound DDT molecule was long enough and, in the vertical direction of the gold electrode surface (“z-direction”), provided adequate space to hold a RuDB molecule. This also meant that, after the first modification step, the other sulfhydryl group of the DDT molecule was available in space to bond with the AuNPs.
Scanning electron microscopy (SEM) and atomic force microscopy (AFM)
Fig. 1 shows the SEM and AFM images of a DDT/Ru–AuNPs layer on a gold surface. In the high magnification SEM image (Fig. 1(A)) of the modified gold surface, the granular structure was extremely evident as expected, and was observably distinguishable from the smooth gold background (inset). In accordance with the used AuNPs, the observed granules with an average diameter of 10 nm were distributed with uniform spherical shapes. It is worth mentioning that the DDT layers and the RuDB located below the AuNPs could not be observed here.
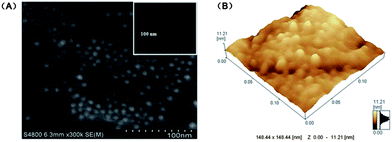 |
| Fig. 1 (A) SEM and (B) AFM images of the DDT/Ru–AuNPs layer on the gold surface. The inset in (A) shows the SEM image of the bare gold substrate. | |
As shown in Fig. 1(B), the highest point of the DDT/Ru–AuNPs monolayer on the Au substrate was 11.21 nm, which indicated that some parts of the gold substrate were modified with the erect DDT molecules (∼18.10 Å in length theoretically) and AuNPs (10 nm in diameter) monolayer. It can also be inferred from the SEM and AFM images that some flat structures of the DDT molecules may be distributed on the gold surface where the AuNPs were not attached, leading to a low average height in Fig. 1(B).
Cyclic voltammetry (CV) and electrochemical impedance spectroscopy (EIS)
Fig. 2(A) displays the CV curves of Fe(CN)63−/4− probed by the bare gold electrode and the gold electrode at different modification stages. Clearly, the currents of the gold electrode at different modification stages (curves b, c, d and e) were much smaller than those of the bare gold electrode (curve a), and no obvious voltammetric response of Fe(CN)63−/4− was even exhibited in some cases. The presence of DDT significantly blocked the electron transfer on the gold electrode surface, resulting in an obvious decrease in the current and even a disappearance of the redox peaks for Fe(CN)63−/4−, which is in accordance with a previous report.22 Thus, curves b, c, d and e show the variation in the capacitive current. Compared with the DDT/Ru monolayer (curve b), the DDT/Ru–AuNPs films presented an increased current because the AuNPs possess excellent conductivity properties and effectively promote electron transfer between the electrolyte and the electrode surface. As expected, the currents decreased at first, followed by an increase when the modification was repeated.
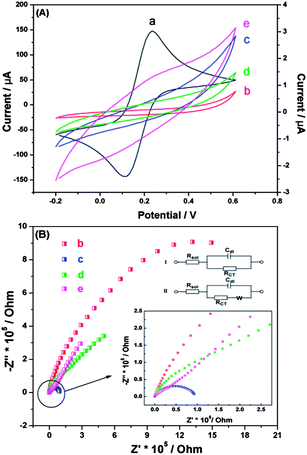 |
| Fig. 2 (A) CV and (B) EIS of 0.005 M Fe(CN)63−/4− and 0.1 M KCl at (a) a bare gold electrode, (b) a DDT/Ru modified gold electrode, (c) a DDT/Ru–AuNPs monolayer modified gold electrode, (d) a DDT/Ru–AuNPs–DDT/Ru modified gold electrode and (e) a DDT/Ru–AuNPs double layer modified gold electrode. In (A), curve a corresponds to the left Y axis, while curves b, c, d and e correspond the right axis. The circuits (I) and (II) in (B) were selected to fit curves b, c and d, e, respectively. | |
Electrochemical impedance spectroscopy (EIS) was also employed to characterize the stepwise self-assembly procedure of the DDT/Ru–AuNPs double layer on the gold electrode surface. The EIS of the bare gold electrode exhibited an almost straight line (data not shown), which was a feature of the diffusion-limited process.31 In Fig. 2(B), curve b for the DDT/Ru monolayer modified gold electrode exhibits part of a semicircle, while curve c for the DDT/Ru–AuNPs film modified gold electrode shows a whole semicircle with a much smaller diameter. Both are characteristic of the electron transfer limited process. After the DDT/Ru and AuNPs were successively attached to the DDT/Ru–AuNPs monolayer modified gold electrode, their impedance spectra, curves d and e, included semicircle portions at higher frequencies and a linear portion at lower frequencies. In order to obtain the proximal resistance values, circuits (I) and (II) were selected to fit curves b, c and d, e, respectively. The results showed that the changes in the charge transfer resistance (Rct) of the gold electrode at different modification stages were extraordinarily obvious. The Rct of the bare gold electrode with a 4 mm diameter was about 73 Ω, while it sharply increased to 2.050 MΩ after DDT/Ru was deposited onto the gold electrode surface. This is because DDT attached on the gold electrode surface to form a dense insulating monolayer, which significantly obstructed the electron transfer and resulted in the dramatically increased Rct value. When the DDT/Ru monolayer modified electrode was treated with AuNPs, the Rct decreased to 92.60 kΩ, indicating that the AuNPs had successfully assembled on the gold electrode surface and provided the necessary conduction pathways, making it easier for electron transfer to take place.32 Subsequently, the Rct increased to 180.8 kΩ, followed by a decrease to 30.63 kΩ when another DDT/Ru layer and AuNPs layer were attached successively. These data show that a DDT/Ru–AuNPs double layer had formed, which was consistent with the CV results.
X-ray photoelectron spectroscopy (XPS)
Since X-ray photoelectron spectroscopy (XPS) provides elemental information about the surface composition, it was applied to further confirm the attachment of the DDT/Ru–AuNPs double layer on the gold electrode surface. The XPS spectra of the DDT/Ru–AuNPs double layer on the gold substrate is shown in Fig. 3(A), and clearly indicates the presence of Ru (3d), C (1s), S (2p) and Au (4f). In addition, high-resolution XPS spectra of Ru (3d), C (1s), S (2p) and Au (4f) provide abundant information on the DDT/Ru–AuNPs double layer. Curve fitting of the high-resolution XPS spectrum at the C (1s), Ru (3d) region (Fig. 3(B)) generated three prominent peaks at 284.77 eV, 281.96 eV and 285.86 eV, which are assigned, respectively, to C (1s), Ru (3d5/2) and Ru (3d3/2), evidencing methylene carbon33 and Ru(II).34 The binding energy of the S (2p) peak shown in Fig. 3(C) is 163.48 eV, which is close to the S (2p3/2) of the alkyl sulfhydryl bonded to Au,33 implying the existence of a Au–S bond. Moreover, as shown in Fig. 3(D), in the Au (4f) region, curve fitting resulted in two peaks at 83.79 eV and 87.46 eV for Au (4f7/2) and Au (4f5/2) respectively,32 which is further supporting evidence for the existence of a Au–S bond. The XPS data for each element are consistent with previously published studies. Thus, the attachment of the DDT/Ru–AuNPs films on the gold electrode surface was further confirmed by XPS analysis.
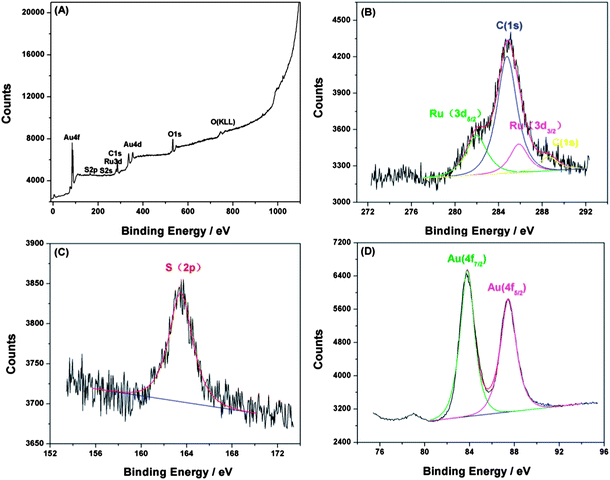 |
| Fig. 3 (A) XPS spectra of the DDT/Ru–AuNPs double layer on the gold surface. High-resolution XPS spectra of (B) Ru (3d) and C (1s), (C) S (2p), and (D) Au (4f) for the DDT/Ru–AuNPs double layer. | |
ECL behavior of RuDB immobilized in the net structured film
A DDT/Ru–AuNPs double layer modified gold electrode (4 mm in diameter) was employed to investigate the ECL of RuDB immobilized in the net structure. The electrolyte was purged with N2 and a constant flow (20 mL s−1) was maintained during the measurements. As a co-reactant, TPrA was consumed continuously in the ECL reaction and the stirring action of N2 guaranteed that the TPrA molecules diffused to the surface of the AuNPs in time to participate in the ECL reaction. The ECL was generated via a single oxidative step including both TPrA and Ru(bpy)32+ oxidations according to well-established methods.2 A characteristic peak for Ru(bpy)32+ at around 1.2 V was displayed, which is similar to that of Ru(bpy)32+ in an aqueous solution reported previously.35Fig. 4 clearly demonstrates that the ECL of RuDB immobilized in the net structure possessed a much high intensity and stability, which is attributed to the increased active area and the improved electrochemical properties of the interface in the presence of AuNPs.
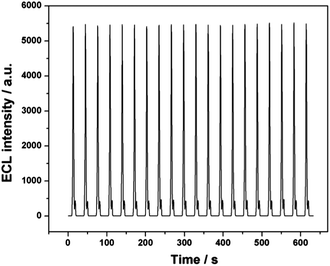 |
| Fig. 4 ECL intensity–time curves of the DDT/Ru–AuNPs double layer with 25 mM TPrA in pH 7.5 PBS (0.2 M) solution over 20 continuous cycles of CV scans. The voltage of the PMT was set at 800 V. Scan rate: 100 mV s−1. | |
The pH value of the electrolyte and the scan rate were two major influencing factors on the ECL intensity of the Ru(bpy)32+–TPrA system. Thus, the effects of pH and scan rate on the ECL of RuDB were investigated and the results are shown in Fig. S3, ESI.† The PBS solution at pH 7.5 and a scan rate of 100 mV s−1 were the optimal conditions and were therefore employed for the ECL investigation in the following experiments.
In order to confirm the importance of the net structure for immobilization of the ECL reagent, the ECL of the DDT/Ru–AuNPs double layer, DDT/Ru–DDT/Ru and Ru–AuNPs–Ru–AuNPs modified gold electrodes were compared. As shown in Fig. 5(A), in the absence of either DDT or AuNPs, much lower and unstable ECL signals were observed. When the gold electrode was modified twice with the solution containing DDT and RuDB, the DDT/Ru–DDT/Ru modified gold electrode was obtained. Without the modification using AuNPs, the ECL intensity of the resultant gold electrode was only 7.6% of that of the DDT/Ru–AuNPs double layer modified gold electrode. On the other hand, a gold electrode was modified with a solution containing only RuDB in the first step, and then modified with AuNPs. Repeating this cycle led to a Ru–AuNPs–Ru–AuNPs modified gold electrode, which exhibited a much weaker ECL signal (only 1.3% of that of the DDT/Ru–AuNPs double layer modified gold electrode). Moreover, it was observed that both the DDT/Ru–DDT/Ru and Ru–AuNPs–Ru–AuNPs modified gold electrodes exhibited very unstable ECL signals. The ECL intensity decreased continuously to the point where very weak ECL peaks were presented. Thus, both AuNPs and DDT played crucial roles in the effective immobilization and improved ECL performance of RuDB.
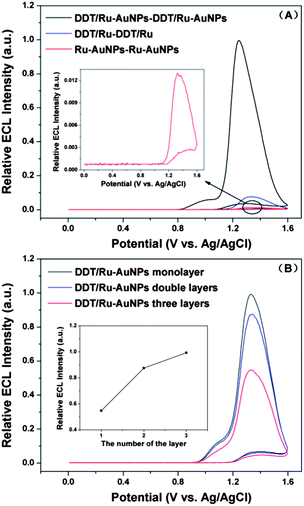 |
| Fig. 5 ECL–potential graphs for (A) the DDT/Ru–AuNPs double layer, DDT/Ru–DDT/Ru and Ru–AuNPs–Ru–AuNPs modified gold electrodes; and (B) modified gold electrodes with different numbers of DDT/Ru–AuNPs layers. | |
The desired number of DDT/Ru–AuNPs layers could be fabricated when the modification process was repeated. Fig. 5(B) shows the ECL for different numbers of DDT/Ru–AuNPs layers on the gold electrode surface. As the number of layers increased, much more RuDB molecules were immobilized in the larger net structure, leading to the obvious increase in ECL intensity.
ECL sensing by the DDT/Ru–AuNPs layers for phenol
In ECL analysis, numerous analytes are detected based on their inhibition properties. The inhibition effect of phenols on the Ru(bpy)32+ ECL signals was reported previously.27,28 Herein, the gold electrode coated with the DDT/Ru–AuNPs double layer was used as an ECL probe in 0.2 M PBS solution (pH 7.5) at a scan rate of 100 mV s−1 to detect phenol. As shown in Fig. 6, the ECL intensity was significantly inhibited by phenol and was linearly proportional to the concentration of phenol. The detection limit was as low as 0.6 nM and the ECL intensity was quenched almost completely by only tens of nM of phenol. The sensitive response of the presented ECL sensor to phenol is attributed to the high-intensity and stable ECL signal of RuDB in the net structure and the favourable electrochemical properties of AuNPs.
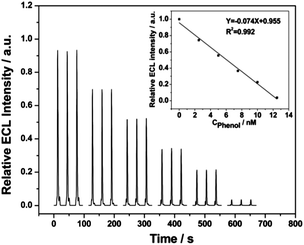 |
| Fig. 6 ECL intensity–time curves of the DDT–Ru–AuNPs double layer modified gold electrode (4 mm in diameter) in the presence of different concentrations of phenol: 0, 2.5, 5.0, 7.5, 10.0 and 12.5 nM. The inset displays a plot of the ECL intensity against the concentration of phenol. | |
As reported previously,28 the mechanism of this ECL inhibition is associated with energy transfer from the excited-state of Ru(bpy)32+* to benzoquinone. In the reaction process, it is benzoquinone, the electro-oxidation product of phenol, that quenches RuDB*, leading to the decrease in the ECL intensity.
Conclusions
In summary, a special net structure was designed elaborately for the effective immobilization of RuDB molecules on a gold electrode surface. DDT with two sulfhydryl groups was employed for bonding covalently to both the gold electrode and AuNPs to construct a DDT/Ru–AuNPs film on the gold electrode surface. Simultaneously, RuDB molecules became distributed in the spaces between the long chains of DDT and were successfully immobilized during the fabrication of the net structure. The modification process could be repeated until the desired number of DDT/Ru–AuNPs layers was obtained. This self-assembly technique for the immobilization of RuDB has been proposed here for the first time and has been employed for solid-state ECL detection. An ECL sensor with a high intensity and good stability was obtained and exhibited ultrasensitive inhibition by phenol. Coupled with the excellent film forming properties and the low consumption but good ECL behaviour of RuDB, this present sensor exhibited great potential in solid-state ECL detection. What is more, the design of the net structure may be promising for other sensing applications.
Acknowledgements
We would express our sincere thanks to the financial support from the National Natural Science Foundation of China (20927007, 21175094 and 21177090) and the Youth Foundation of Sichuan University (2010SCU11048).
Notes and references
- R. J. Forster, P. Bertoncello and T. E. Keyes, Annu. Rev. Anal. Chem., 2009, 2, 359 CrossRef CAS PubMed.
- W. Miao, Chem. Rev., 2008, 108, 2506 CrossRef CAS PubMed.
- M. M. Richter, Chem. Rev., 2004, 104, 3003 CrossRef CAS PubMed.
- H. Wei and E. Wang, TrAC, Trends Anal. Chem., 2008, 27, 447 CrossRef CAS PubMed.
- L. M. Moretto, T. Kohls, D. Badocco, P. Pastore, N. Sojic and P. Ugo, J. Electroanal. Chem., 2010, 640, 35 CrossRef CAS PubMed.
- B. Zhang, S. Shi, W. Shi, Z. Sun, X. Kong, M. Wei and X. Duan, Electrochim. Acta, 2012, 67, 133 CrossRef CAS PubMed.
- Y. Qu, X. Liu, X. Zheng and Z. Guo, Anal. Sci., 2012, 28, 571 CrossRef CAS.
- G. M. Greenway, A. Greenwood, P. Watts and C. Wiles, Chem. Commun., 2006, 85 RSC.
- S. Guo and S. Dong, J. Mater. Chem., 2011, 21, 16704 RSC.
- E. Rampazzo, S. Bonacchi, D. Genovese, R. Juris, M. Marcaccio, M. Montalti, F. Paolucci, M. Sgarzi, G. Valenti, N. Zaccheroni and L. Prodi, Coord. Chem. Rev., 2012, 256, 1664 CrossRef CAS PubMed.
- S. Deng and H. Ju, Analyst, 2013, 138, 43 RSC.
- Y. Zhang, L. Li, H. Yang, Y.-n. Ding, M. Su, J. Zhu, M. Yan, J. Yu and X. Song, RSC Adv., 2013, 3, 14701 RSC.
- Y. Yu, M. Zhou and H. Cui, J. Mater. Chem., 2011, 21, 12622 RSC.
- C. Wang, Y. E. L. Fan, S. Yang and Y. Li, J. Mater. Chem., 2009, 19, 3841 RSC.
- L. Mao, R. Yuan, Y. Chai, Y. Zhuo, X. Yang and S. Yuan, Talanta, 2010, 80, 1692 CrossRef CAS PubMed.
- Z. Liu, C. Zhou, B. Zheng, L. Qian, Y. Mo, F. Luo, Y. Shi, M. M. F. Choi and D. Xiao, Analyst, 2011, 136, 4545 RSC.
- L. Gao, L. Fan and J. Zhang, Langmuir, 2009, 25, 11844 CrossRef CAS PubMed.
- Y. Du, B. Qi, X. Yang and E. Wang, J. Phys. Chem. B, 2006, 110, 21662 CrossRef CAS PubMed.
- Y. Li, F. Yang and X. Yang, Analyst, 2009, 134, 2100 RSC.
- X. Yang, R. Yuan, Y. Chai, Y. Zhuo, L. Mao and S. Yuan, Biosens. Bioelectron., 2010, 25, 1851 CrossRef CAS PubMed.
- S. N. Ding, J. J. Xu, W. J. Zhang and H. Y. Chen, Talanta, 2006, 70, 572 CrossRef CAS PubMed.
- M. D. Porter, T. B. Bright, D. L. Allara and C. E. D. Chidsey, J. Am. Chem. Soc., 1987, 109, 3559 CrossRef CAS.
- C. J. Addison, S. O. Konorov, A. G. Brolo, M. W. Blades and R. F. B. Turner, J. Phys. Chem. C, 2009, 113, 3586 CAS.
- M. Brust, D. Bethell, C. J. Kiely and D. J. Schiffrin, Langmuir, 1998, 14, 5425 CrossRef CAS.
- J. B. Shein, L. M. H. Lai, P. K. Eggers, M. N. Paddon-Row and J. J. Gooding, Langmuir, 2009, 25, 11121 CrossRef CAS PubMed.
- J. Dyne, Y.-S. Lin, L. M. H. Lai, J. Z. Ginges, E. Luais, J. R. Peterson, I. Y. Goon, R. Amal and J. J. Gooding, ChemPhysChem, 2010, 11, 2807 CrossRef CAS PubMed.
- H. Cui, F. Li, M. J. Shi, Y. Q. Pang and X. Q. Lin, J. Electroanal. Chem., 2005, 17, 589 CrossRef CAS.
- J. McCall, C. Alexander and M. M. Richter, Anal. Chem., 1999, 71, 2523 CrossRef CAS PubMed.
-
M. J. Frisch, G. W. Trucks, H. B. Schlegel, G. E. Scuseria, M. A. Robb, J. R. Cheeseman, J. A. Montgomery, Jr., T. Vreven, K. N. Kudin, J. C. Burant, J. M. Millam, S. S. Iyengar, J. Tomasi, V. Barone, B. Mennucci, M. Cossi, G. Scalmani, N. Rega, G. A. Petersson, H. Nakatsuji, M. Hada, M. Ehara, K. Toyota, R. Fukuda, J. Hasegawa, M. Ishida, T. Nakajima, Y. Honda, O. Kitao, H. Nakai, M. Klene, X. Li, J. E. Knox, H. P. Hratchian, J. B. Cross, V. Bakken, C. Adamo, J. Jaramillo, R. Gomperts, R. E. Stratmann, O. Yazyev, A. J. Austin, R. Cammi, C. Pomelli, J. Ochterski, P. Y. Ayala, K. Morokuma, G. A. Voth, P. Salvador, J. J. Dannenberg, V. G. Zakrzewski, S. Dapprich, A. D. Daniels, M. C. Strain, O. Farkas, D. K. Malick, A. D. Rabuck, K. Raghavachari, J. B. Foresman, J. V. Ortiz, Q. Cui, A. G. Baboul, S. Clifford, J. Cioslowski, B. B. Stefanov, G. Liu, A. Liashenko, P. Piskorz, I. Komaromi, R. L. Martin, D. J. Fox, T. Keith, M. A. Al-Laham, C. Y. Peng, A. Nanayakkara, M. Challacombe, P. M. W. Gill, B. G. Johnson, W. Chen, M. W. Wong, C. Gonzalez and J. A. Pople, GAUSSIAN 03, Gaussian, Inc., Wallingford, CT, 2004 Search PubMed.
- D. P. Rillema, D. S. Jones, C. Woods and H. A. Levy, Inorg. Chem., 1992, 31, 2935 CrossRef CAS.
- G. Jie, B. Liu, H. Pan, J. J. Zhu and H. Y. Chen, Anal. Chem., 2007, 79, 5574 CrossRef CAS PubMed.
- L. Zhang, X. U. Jiang, E. K. Wang and S. J. Dong, Biosens. Bioelectron., 2005, 21, 337 CrossRef CAS PubMed.
- X. Zhao, Y. Cai, T. Wang, Y. Shi and G. Jiang, Anal. Chem., 2008, 80, 9091 CrossRef CAS PubMed.
- C. Elmasides, D. I. Kondarides, W. Grünert and X. E. Verykios, J. Phys. Chem. B, 1999, 103, 5227 CrossRef CAS.
- Y. Zu and A. J. Bard, Anal. Chem., 2000, 72, 3223 CrossRef CAS.
Footnote |
† Electronic supplementary information (ESI) available. See DOI: 10.1039/c3ra44059a |
|
This journal is © The Royal Society of Chemistry 2014 |