Spin canting and metamagnetism in 3D pillared-layer homospin cobalt(II) molecular magnetic materials constructed via a mixed ligands approach†
Received
24th September 2013
, Accepted 6th January 2014
First published on 7th February 2014
Abstract
Two new 3D pillared-layer cobalt(II) molecular magnetic materials, formulated as {[Co2(TDA)(TZ)(H2O)2]·H2O}n (1) and {[Co3(TDA)2(bpy)3]·6.75H2O}n (2) (H3TDA = 1H-1,2,3-triazole-4,5-dicarboxylic acid, HTZ = 1H-1,2,4-triazole and bpy = 4,4′-bipyridine), have been successfully assembled with H3TDA and auxiliary ligands, respectively. In 1, the neighboring Co(II) ions are connected via TDA3− to generate 2D layers, which are further bridged by TZ− to build a 3D 8-connected bcu net with a Schläfli symbol of (424·64), whereas in 2, the nearest Co(II) ions are connected via TDA3− to form a hexagonal [Co6(TDA)6]6− macrocycle composed of 24-membered atoms, which can be further linked to each other to generate 2D Kagomé layers. The Kagomé layers are connected by bpy pillars to display a 3D (3,4,4,4)-connected KAVGAQ net with a Schläfli symbol of {63}2{64·102}{64·82}2. Magnetic studies reveal that both of them show the spin canting and metamagnetic behaviors with critical temperatures of 3.5 K and 4 K, respectively.
Introduction
In the past decade, the design and construction of molecular magnetic materials have attracted considerable attention due to their potential applications in high-density information storage and quantum computing.1,2 Such molecular materials exhibiting versatile magnetic behaviors such as ferromagnetism,3 antiferromagnetism,4 ferrimagnetism5 and metamagnetism6 have been investigated in many homometallic (pure 3d or 4f) or heterometallic (3d–4f) complexes, in which spin carriers (in most cases the spin carriers are metal ions) are linked via bridging ligands in a special topological motif to communicate magnetic information. Generally speaking, ferromagnetic interaction is anticipated to generate a large spin ground state and helps to observe magnetic bistability below the critical temperature, but it is quite challenging to obtain ferromagnetic interaction compared to antiferromagnetic interaction. In contrast, antiferromagnetic interaction is more common in nature, always leading to the antiferromagnetic ground state.1a Compared to the classical ferromagnetism and antiferromagnetism, metamagnetism undergoes magnetic phase transitions induced by an external magnetic field at low temperature, and shows a suppressible long-range magnetic ordering and S-shape M–H curve.6 However, due to the critical prerequisites such as special topological spin structures and appropriate magnetic exchange pathways between spin carriers, examples of metamagnetism are still relatively limited. A useful strategy to obtain metamagnetic molecular materials is to assemble the spin carriers in a special topology such as triangle, which could generate competition of the magnetic moments between ferromagnetic interaction and antiferromagnetic interaction. In this case, the spins could be constrained to maintain a parallel state upon an external magnetic field. However, it is still an exciting challenge to synthesize such molecular magnetic materials in a rational way due to the difficulties in both syntheses and magneto-structural correlation analyses.
Our strategy to synthesize molecular magnetic materials is to assemble spin carriers via a mixed ligands approach which could result in special coordination symmetries.7 As a simple but useful secondary building unit (SBU), 1H-1,2,3-triazole-4,5-dicarboxylic acid (H3TDA) has been studied by us and other groups.7–9 For example, a 3D homospin cobalt(II) molecular topological ferrimagnet {[H2N(CH3)2][Co3(TDA)2(TZ)(H2O)]·3.5H2O}n, showing a critical temperature of 9 K and a compensation temperature of 5 K, has been reported recently.7b Antiferromagnetic interactions transmitted by H3TDA were observed in a 2D stair-like transition-metal coordination polymer {[Mn3(TDA)2(H2O)6]·6H2O}n
8a and a 3D heterometallic coordination polymer {[Cu4Na4(TDA)4(H2O)7]}n with the unprecedented planar metalloporphyrin-like subunits.9a Spin-competing behaviors as well as unusual multi-step magnetization jumps were studied in 3D Mn(II) coordination polymers {[Mn3(TDA)2(H2O)2(NH3)2]}n and {[Mn3(TDA)2(H2O)4]}n.9b More recently, two 2D heterometallic flexible mixed-spin Kagomé coordination polymers {[Cu2M(TDA)2(H2O)2]·2H2O}n [M = Fe(II) or Mn(II)] were reported, showing a reversible dynamic magnetic change.9c These results indicate that H3TDA is an effective magnetic exchange pathway to communicate magnetic information between the metal ions and therefore needs to be studied further.
In this contribution, the Co(II) ion is selected as spin carriers due to its high spin quantum number and magnetic anisotropy.1c,10 As a continuous exploitation of the concept of mixed ligands approach to synthesize Co(II)-based molecular ordered materials,7 two new 3D pillared-layer Co(II) molecular magnetic materials, {[Co2(TDA)(TZ)(H2O)2]·H2O}n (1) and {[Co3(TDA)2(bpy)3]·6.75H2O}n (2) (HTZ = 1H-1,2,4-triazole and bpy = 4,4′-bipyridine), were successfully obtained and characterized in detail. Both 1 and 2 exhibit 3D pillared-layer structures, but corresponding to an 8-connected bcu net with a Schläfli symbol of (424·64) and a (3,4,4,4)-connected KAVGAQ net with a Schläfli symbol of {63}2{64·102}{64·82}2, respectively. Magnetic studies reveal that both of them show interesting coexistence of spin-canting and metamagnetic behaviors at critical temperatures of 3.5 K and 4 K, respectively.
Experimental section
General methods and materials
H3TDA was prepared according to the literature.11 Other reagents and solvents were purchased from commercial sources and used as received without further purification. Elemental analyses for C, H and N were performed using a Perkin-Elmer 240 CHN elemental analyzer. IR spectra were recorded in the range 400–4000 cm−1 with a Bruker Tensor 27 Spectrometer on KBr disks. Powder X-ray diffraction measurements were recorded on a D/Max-2500 X-ray diffractometer using Cu/Kα radiation. Thermogravimetric analysis (TGA) was carried out using a Delta Series TA-SDTQ 600 under a nitrogen atmosphere from room temperature to 800 °C (10 °C min−1) using aluminum crucibles. Magnetic susceptibility measurements were performed on a Quantum Design SQUID MPMS XL-7 magnetometer. Diamagnetic corrections were made with Pascal's constants and a sample holder, which were approximately calculated to be −1.97 × 10−4 for 1 and −5.37 × 10−4 cm3 mol−1 for 2, respectively.
Synthesis of {[Co2(TDA)(TZ)(H2O)2]·H2O}n (1).
A mixture of Co(OAc)2·4H2O (0.0776 g, 0.3 mmol), H3TDA (0.0314 g, 0.2 mmol) and HTZ (0.0138 g, 0.2 mmol) was dissolved in H2O (10 mL) and C2H5OH (2.5 mL). The mixture was sealed in a 25 mL Teflon-lined stainless steel reactor heated at 160 °C for 72 h, and then cooled to room temperature at a speed of 1.5 °C h−1. Red rectangular crystals of 1 in ca. 12% yield (based on Co) were obtained. Anal. calcd for C6H8N6O7Co2 (%): C, 18.29; H, 2.05; N, 21.33. Found (%): C, 18.24; H, 2.09; N, 21.54. IR (KBr, cm−1): 3395(s), 1620(vs), 1585(s), 1513(m), 1423(vs), 1389(m), 1233(w), 1153(m), 1070(w), 875(w), 665(w).
Synthesis of {[Co3(TDA)2(bpy)3]·6.75H2O}n (2).
A mixture of H3TDA (0.0314 g, 0.2 mmol), bpy (0.0156 g, 0.1 mmol), Co(ClO4)2·6H2O (0.0366 g, 0.1 mmol), GdCl3·6H2O (0.0371 g, 0.1 mmol), LiOH·H2O (0.0084 g, 0.2 mmol) and H2O (5 mL) was sealed in a 25 mL Teflon-lined stainless steel reactor heated at 120 °C for 72 h, and subsequently cooled to room temperature at a rate of 1.2 °C h−1. Orange block crystals of 2 in ca. 35% yield (based on Co) were collected. Anal. calcd for C38H37.5N12O14.75Co3 (%): C 42.45, H 3.52, N 15.63. Found (%): C 42.18, H 3.94, N 15.65. IR (KBr, cm−1): 3429(vs), 1579(vs), 1414(vs), 1290(w), 1224(m), 1050(m), 823(s), 727(m), 629(s), 496(w).
Caution: Perchlorate salts and its complexes of metal ions are potentially explosive. Only a small amount of material should be prepared, and it should be handled with care.
X-ray crystallography
Data collections for 1 and 2 were performed on an Oxford Supernova diffractometer with graphite-monochromated Mo-Kα radiation (λ = 0.71073 Å). The structures were solved by direct methods. All non-hydrogen atoms were refined by full-matrix least-squares techniques on F2 using the SHELXS-97 and SHELXS-97 programs.12 Anisotropic thermal parameters were assigned to all non-hydrogen atoms. The hydrogen atoms were placed in idealized positions and located in the difference Fourier map. CCDC 909523 for 1 and CCDC 928525 for 2 contain the supplementary crystallographic data for this paper. The crystallographic data for 1 and 2 are listed in Table 1. Selected bond lengths and angles of 1 and 2 are listed in Table S1.†
Table 1 Crystal data and structural refinements for 1 and 2
Compounds |
1
|
2
|
R
1 = ∑(|F0| − |Fc|)/∑|F0|.
wR2 = [∑{w(|F0|2 − |Fc|2)2}/∑[w(|F0|2)2]]1/2.
|
Formula |
C6H8N6O7Co2 |
C38H37.5N12O14.75Co3 |
Formula weight |
394.04 |
1075.08 |
Temperature (K) |
293(2) |
293(2) |
Crystal system |
Monoclinic |
Triclinic |
Space group |
P21/n |
P![[1 with combining macron]](https://www.rsc.org/images/entities/char_0031_0304.gif) |
a (Å) |
8.9980(9) |
11.4527(14) |
b (Å) |
7.7962(9) |
11.6239(9) |
c (Å) |
18.1677(18) |
11.7754(10) |
α (°) |
90 |
64.825(8) |
β (°) |
101.649(10) |
81.406(9) |
γ (°) |
90 |
72.316(9) |
V (Å3) |
1248.2(2) |
1351.3(3) |
Z
|
4 |
1 |
D
c (g cm−3) |
2.086 |
1.304 |
μ (mm−1) |
2.704 |
0.977 |
F(000) |
776 |
535 |
θ range (°) |
2.36–25.00 |
3.09–25.01 |
GOF on F2 |
1.195 |
1.088 |
Reflections collected/unique |
4555/2203 |
8802/4747 |
R
int
|
0.0436 |
0.0762 |
R
1,a wR2 b [I > σ(I)] |
0.0707, 0.1587 |
0.0866, 0.2442 |
R
1, wR2 (all data) |
0.0892, 0.1666 |
0.1183, 0.2751 |
Residues (e Å−3) |
1.530/−0.569 |
1.292/−0.780 |
Results and discussion
Syntheses of 1 and 2
Solvothermal reaction of Co(II) ions with H3TDA and HTZ in a 4
:
1 mixture of water and ethanol at 160 °C, in the absence of any base, gives compound 1. When the auxiliary ligand HTZ was replaced by bpy, the reaction of Co(II) and H3TDA in the presence of Gd(III) and LiOH in water at 120 °C produces compound 2. The guest molecules and thermal stabilities for compounds 1 and 2 were confirmed by TGA measurements (Fig. S1, ESI†). The crystalline phase purities for 1 and 2 were confirmed by PXRD, in which the diffraction peaks of bulk samples are consistent with the simulated patterns in terms of the single crystal data (Fig. S2 and S3, ESI†).
Description of the crystal structures
Single-crystal X-ray diffraction analysis reveals that 1 crystallizes in monoclinic space group P21/n. Each unit cell contains crystallographically independent two Co(II) ions, one TZ−, one TDA3− ligand and two coordinated water molecules (Fig. 1a). Co1 is coordinated by three oxygen atoms (O2A, O3A and O4) and one nitrogen atom (N3) from two TDA3− and two nitrogen atoms (N5 and N6A) from two TZ− to form a distorted octahedral {CoN3O3} configuration. The bond lengths of Co1–O and Co1–N are in the range of 2.079(6)–2.183(6) Å and 2.070(7)–2.101(7) Å, respectively. The coordination environment of Co2 is similar to that of Co1, adopting an octahedral {CoN3O3} environment with one oxygen atom (O1A) and two nitrogen atoms (N1A and N2) from two TDA3−, two oxygen atoms (O5 and O6) from two water molecules and one nitrogen atom (N4) from TZ−. The bond lengths of Co2–O and Co2–N are in the range of 2.083(7)–2.156(7) Å and 2.121(7)–2.156(7) Å, respectively. The neighboring Co(II) ions are connected via TDA3− to generate 2D layers (Fig. 1b). Interestingly, in these 2D layers, there are single-chain helicate motifs with a screw pitch of 7.796 Å, in which the helixes show either left-hand (L) or right-hand (R). Additionally, the L- and R-type single-chain helixes are arranged alternatively, resulting in achirality of 1, confirmed by the achiral space group of 1. The neighboring layers are further bridged by TZ− to build a 3D framework (Fig. 1c). Topologically, if the tetranuclear Co(II) clusters are viewed as 8-connected nodes, the entire structure can be simplified as an 8-connected bcu net with a Schläfli symbol of (424·64)13 (Fig. 1d).
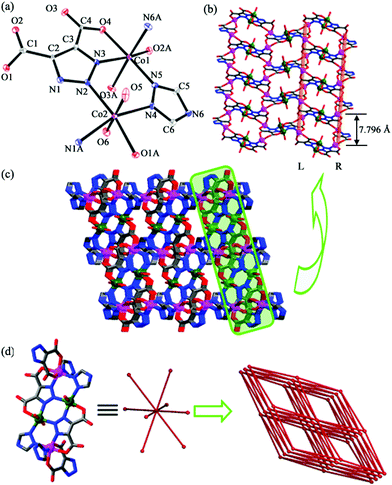 |
| Fig. 1 (a) The structural unit of 1 (thermal ellipsoids are drawn at the 30% probability level). (b) The 2D layer in 1. (c) The 3D structure of 1. Pink: Co1; cyan: Co2; red: O; blue: N; grey: C. (d) A schematic representation of the bcu network for 1. All hydrogen atoms and uncoordinated molecules were omitted for clarity. | |
2 crystallizes in triclinic space group P
. Each unit cell contains crystallographically independent one and a half Co(II) ions, one TDA3−, and one and a half bpy (Fig. 2a). Co1 and Co2 display similar coordination environments, adopting distorted octahedral {CoO2N4} coordination geometries with two oxygen atoms (O3 and O3A for Co1, O2 and O2A for Co2) and two nitrogen atoms (N3 and N3A for Co1, N1 and N1A for Co2) from two TDA3− ligands, two nitrogen atoms (N4 and N4A for Co1, N5 and N5A for Co2) from two bpy ligands. The bond lengths of Co1–N are in the range of 2.069(6)–2.178(7) Å and that of Co1–O is 2.100(5) Å, while the bond lengths of Co2–N are in the range of 2.075(6)–2.172(7) Å and that of Co2–O is 2.110(5) Å, respectively. In contrast to the coordination environments of Co1 and Co2, Co3 adopts a distorted octahedral {CoO4N2} geometry with four oxygen atoms (O1, O1A, O4 and O4A) from two TDA3−, and two nitrogen atoms (N6 and N6A) from two bpy ligands. The bond lengths of Co3–O are in the range of 2.078(5)–2.088(5) Å and that of Co3–N is 2.137(7) Å, respectively. Each TDA3− ligand chelates three Co(II) ions through the carboxylate oxygen atoms and the triazolate nitrogen atoms. In this case, each Co(II) ion is coordinated by two TDA3− ligands. Therefore, a hexagonal [Co6(TDA)6]6− macrocycle composed of 24-membered atoms with a diameter of about 1.2 nm is generated (Fig. 2b), which is further connected by TDA3− to generate 2D Kagomé layers (Fig. 2c). The 2D Kagomé layers are linked via bpy as pillars to build a 3D framework (Fig. 2d). Topologically, if TDA3− are viewed as 3-connected nodes with bpy ligands as bridging spacers, the framework can be described as a (3,4,4,4)-connected KAVGAQ net with a Schläfli symbol of {63}2{64·102}{64·82}2 (Fig. 2e), which was also observed in our previously reported Zn(II)-based framework {[Zn3(TDA)2(bpy)3](bpy)·10.5H2O}n.8c
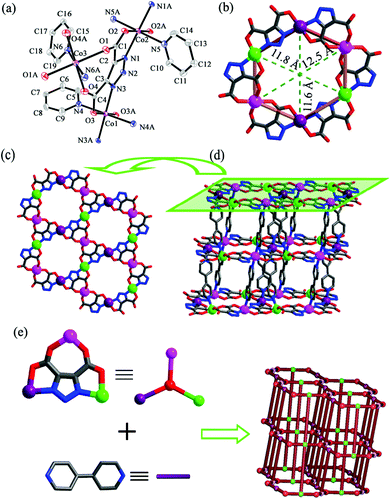 |
| Fig. 2 (a) The structural unit of 2 (thermal ellipsoids are drawn at the 30% probability level). (b) The hexagonal [Co6(TDA)6]6− macrocycles with 24-membered atoms. (c) The 2D Kagomé layer in 2. (d) The 3D framework of 2; green: Co1; purple: Co2; pink: Co3; red: O; blue: N; grey: C. (e) A schematic representation of the KAVGAQ network for 2. All hydrogen atoms and uncoordinated water molecules were omitted for clarity. | |
Magnetic properties
Static (dc) magnetic susceptibilities of 1 and 2 have been measured at an applied magnetic field of 1 kOe in the temperature range of 2–300 K. As shown in Fig. 3a, the χMT value of 1 at room temperature is 6.52 cm3 K mol−1, much higher than the expected spin-only value of 3.76 cm3 K mol−1 for two free high-spin Co(II) ions in an octahedral coordination environment due to the spin–orbit coupling.14 With decreasing temperature, χMT values decrease gradually, reaching a minimum of 1.02 cm3 K mol−1 at 4 K, then increase slightly to a maximum of 1.08 cm3 K mol−1 at 3 K, and finally drop to 0.81 cm3 K mol−1 at 2 K. The behavior around 3 K should be related to the spin-canting effect15 and that below 3 K may be due to intermolecular antiferromagnetic interactions and/or magnetic anisotropy of Co(II) ions.16 The fitting by the Curie–Weiss law with the data above 50 K gives C = 7.62 cm3 K mol−1 and θ = −48.9 K. The large and negative Weiss constant suggests dominant antiferromagnetic interactions as well as spin–orbit coupling. The field dependence of magnetizations performed at 2 K in the range of 0–50 kOe shows an S-shaped curve, indicating metamagnetic behavior (Fig. 3b). The critical field at 2 K is ca. 400 G estimated as the field at which a maximum dM/dH value is observed (inset of Fig. 3b). The zero-field-cooled (ZFC) magnetizations and field-cooled (FC) magnetizations differ below 3.5 K (Fig. 3c), suggesting the onset of long-range magnetic ordering of the canted units below this temperature. Plots of χMvs. T at applied fields ranging from 50 to 2000 Oe in 2–30 K (Fig. S4, ESI†) show that the susceptibilities are field-independent above 3.5 K, while they are strongly field-dependent below 3.5 K. This behaviour should correspond to field induced transition.6d,17 Dynamic alternating current (ac) magnetic susceptibilities show a frequency-independent maximum in χ′ accompanied by χ′′ without signal around 3.5 K, further confirming the presence of long-range magnetic ordering below this temperature (Fig. S5, ESI†). At 2 K, a hysteresis loop is observed with a coercive field (Hc) of 500 Oe and a remnant magnetization of 0.04Nβ (Fig. 3d), confirming the hard magnet-like behavior.18 These phenomena confirm the occurrence of spontaneous magnetization of 1 which is in agreement with the coexistence of metamagnetism and spin-canted antiferromagnetism. The canting angle (α) can be estimated to be 1.07°, calculated by the equation of α = 2 × sin−1Mr/(2 × Ms) (Mr is the remnant magnetization and Ms = gSNβ, where g = 4.3, S = 1/2 for the octahedral Co(II) at 2 K).19
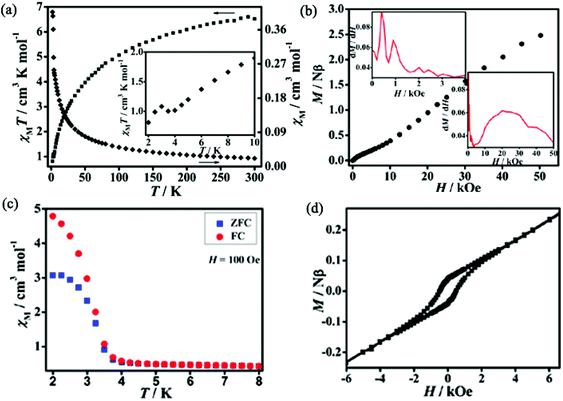 |
| Fig. 3 (a) The temperature dependence of magnetic susceptibilities for 1 in the range of 2–300 K at 1 kOe. Inset: temperature dependence of magnetic susceptibilities for 1 in the range of 2–10 K. (b) The field dependence of magnetizations for 1 at 2 K. Inset: the plots of the dM/dH vs. H at 2 K for 1. (c) ZFC/FC plots at 100 Oe for 1. (d) The hysteresis loop of 1 at 2 K. | |
Dc magnetic susceptibilities of 2 are plotted in Fig. 4a. At room temperature, the χMT value is 7.41 cm3 K mol−1, significantly higher than the expected spin-only value of 3.76 cm3 K mol−1 for three high-spin Co(II) ions. With decreasing the temperature, the χMT values decrease gradually, reaching a minimum of 1.61 cm3 K mol−1 at 6.5 K, then increases slightly to a maximum of 1.95 cm3 K mol−1 at 4.5 K, and finally drops to 0.68 cm3 K mol−1 at 2 K. Similarly to 1, the behavior of 2 around 4.5 K should be related to the spin-canting effect and that below 4.5 K may be due to intermolecular antiferromagnetic interactions and/or magnetic anisotropy of Co(II) ions. The fitting by Curie–Weiss law with the data above 15 K gives C = 8.53 cm3 K mol−1 and θ = −45.90 K, suggesting dominant antiferromagnetic interactions as well as the spin–orbit coupling of the Co(II) centers. The field dependence of magnetizations performed at 2 K in the range of 0–70 kOe show an S-shaped curve, typical of metamagnetic behavior (Fig. 4b). The critical field at 2 K is ca. 3500 G (inset of Fig. 4b). The magnetizations of ZFC and FC were measured in the temperature range of 2–15 K (Fig. 4c). The plots of ZFC magnetizations and FC magnetizations are similar to that of the reported metamagnetic 2D molecular material {[Ni(μ-N3)2(N,N-Et2-N′-Me-en)]}n (N,N-Et2-N′-Me-en = N,N-diethyl-N′-methylethylenediamine), featuring short-range, intralayer, ferromagnetic behavior and weak interlayer antiferromagnetic interactions.20 The FC magnetizations measured under an applied field of 50 Oe increase rapidly on cooling to 4 K and then diminish. This behavior is typical of metamagnetic ordering. The ZFC magnetizations measured upon warming under an applied field of 50 Oe show a maximum at 4 K and decrease rapidly as the temperature is increased further. The ZFC magnetizations and FC magnetizations show a divergence at 4 K, implying the onset of long-range magnetic ordering of the canted units below this temperature. Dynamic alternating current magnetic susceptibilities show a frequency-independent maximum in χ′ accompanied by χ′′ without signal at 4 K, further confirming the presence of long-range magnetic ordering at this temperature (Fig. S6, ESI†). Additionally, a small coercive field of 15 Oe and a remnant magnetization of 0.002Nβ are observed (Fig. 4d), confirming the soft magnet-like behavior. These results indicate the occurrence of spontaneous magnetization of 2, corresponding to metamagnetism and spin-canted antiferromagnetism. The canting angle (α) is roughly estimated to be about 0.053°.
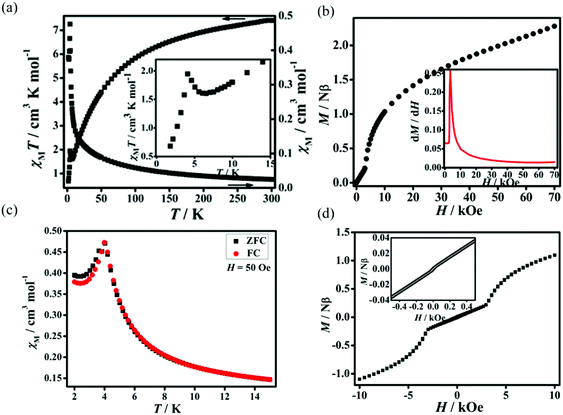 |
| Fig. 4 (a) The temperature dependence of magnetic susceptibilities for 2 in the range of 2–300 K at 1 kOe. Inset: temperature dependence of magnetic susceptibilities for 2 in the range of 2–15 K. (b) The field dependence of magnetizations for 2 at 2 K. Inset: the plot of the dM/dH vs. H at 2 K for 2. (c) ZFC/FC plots at 50 Oe for 2. (d) The hysteresis loop of 2 at 2 K. | |
Discussion
In fact, some molecular metamagnets have been reported in cobalt(II) molecular materials.6,21–23 Among them, one of the most attractive examples are those with 3D pillared-layer structures.6 However, reports on the metamagnetism with spin canting in 3D cobalt(II) materials are still rare and only a few examples of such cobalt(II) materials have been reported.24 Compared to the 3D cobalt(II) metamagnets with spin canting behavior (Table S2†), both 1 and 2 show lower critical temperatures and less remnant magnetization. However, a higher coercive field and a bigger canting angle were observed in 1. The magneto-structural correlation analyses of 1 and 2 are rather complicated. For compound 1, there are two nonequivalent Co(II) magnetic sites (Co1 and Co2), which are aligned in triangular arrangements (Fig. S7a, ESI†). The spins are constrained either parallel or antiparallel to the easy axis, in which magnetic moments of Co1 are antiparallel to that of Co2, to give an overall antiferromagnetic phase. When a small dc field but larger than the critical field at the low temperature was applied to compound 1, the system is in the metamagnetic phase. Furthermore, the interlayer weak antiferromagnetic coupling transmitted by TZ− for 1 should also be considered and is expected to contribute to the magnetic ordering.6 Under a stronger field at low temperature, the magnetic field can overcome the interlayer antiferromagnetic coupling, resulting in a second-order magnetic transition. At variance with compound 1, in 2, there are three Co(II) magnetic sites (Co1, Co2 and Co3, in which the magnetic sites of Co1 and Co2 are almost equivalent), which make the spin alignments arrange in triangular motifs as well and subsequently generate a Kagomé-like lattice (Fig. S7b, ESI†). The lattice consists of corner-sharing triangles of spins with AF coupling between the nearest neighbours. The triangular spins in the Kagomé lattice are likely to form spin frustration structure that precludes the cancelation of the magnetic moments. Such a magnetic structure also allows a magnetic transition from the spin frustration phase to a ferromagnetic phase upon the application of a dc field.
In addition, a mixed ligands approach used in this work has shown a promising way to construct new molecular magnetic materials. This approach can overcome the challenge of critical prerequisites from the spin carriers since the spin carriers in the periodic table and the coordination geometries of metal ions are limited. The mixed ligands could provide different coordination environments of the spin carriers resulting in special topological structures, as well as the expected or unexpected results. Recently, interesting pillared-layer homospin molecular ordered materials have been successfully realized via this approach,6,7 but still deserve to be exploited further.
Conclusions
In summary, two new 3D pillared-layer cobalt(II) molecular magnetic materials assembled with 1H-1,2,3-triazole-4,5-dicarboxylic acid and 1H-1,2,4-triazole or 4,4′-bipyridine have been successfully obtained and structurally studied in detail. Compound 1 is a 3D bcu net, while compound 2 displays a 3D KAVGAQ net. Both of them are 3D metamagnets accompanied by spin canting with the critical temperatures of 3.5 K and 4 K, respectively. This work demonstrates a useful strategy to obtain interesting 3D homospin metamagnets accompanied by spin canting via a mixed ligands approach. More synthetic studies to explore new molecular magnetic materials assembled via such an approach are in progress.
Acknowledgements
This work was supported by the MOST (973 Program 2012CB821702), the NSFC (21171100, and 90922032) and 111 Project (B12015).
Notes and references
-
(a)
O. Kahn, Molecular Magnetism, VCH, Weinheim, 1993 Search PubMed
;
(b)
D. Gatteschi, R. Sessoli and J. Villain, Molecular Nanomagnets, Oxford University Press, New York, 2006 Search PubMed
;
(c) M. Kurmoo, Chem. Soc. Rev., 2009, 38, 1353 RSC
;
(d) J. S. Miller, Chem. Soc. Rev., 2011, 40, 3266 RSC
.
-
(a) M. N. Leuenberger and D. Loss, Nature, 2001, 410, 789 CrossRef CAS PubMed
;
(b) W. Wernsdorfer, N. Aliaga-Alcalde, D. N. Hendrickson and G. Christou, Nature, 2002, 416, 406 CrossRef PubMed
;
(c) L. Bogani and W. Wernsdorfer, Nat. Mater., 2008, 7, 179 CrossRef CAS PubMed
.
-
(a) W. Kaneko, M. Ohba, H. Ōkawa and S. Kitagawa, Inorg. Chem., 2006, 45, 7191 CrossRef CAS PubMed
;
(b) N. Yanai, W. Kaneko, K. Yoneda and S. Kitagawa, J. Am. Chem. Soc., 2007, 129, 3396 CrossRef PubMed
;
(c) H. Ōkawa, M. Sadakiyo, T. Yamada, M. Maesato, M. Ohba and H. Kitagawa, J. Am. Chem. Soc., 2013, 135, 2256 CrossRef PubMed
;
(d) N. A. G. Bandeira, D. Maynau, V. Robert and B. L. Guennic, Inorg. Chem., 2013, 52, 7980 CrossRef CAS PubMed
.
-
(a) P. Kar, R. Haldar, C. J. Gomez-Garcia and A. Fhosh, Inorg. Chem., 2012, 51, 4265 CrossRef CAS PubMed
;
(b) T. Liu, Y. H. Chen, Y. J. Zhang, Z. M. Wang and S. Gao, Inorg. Chem., 2006, 45, 9148 CrossRef CAS PubMed
;
(c) Y. F. Bi, S. T. Wang, M. Liu, S. C. Du and W. P. Liao, Chem. Commun., 2013, 49, 6785 RSC
;
(d) K. C. Xiong, F. L. Jiang, Y. L. Gai, D. Q. Yuan, D. Han, J. Ma, S. Q. Zhang and M. C. Hong, Chem.–Eur. J., 2012, 18, 5536 CrossRef CAS PubMed
.
-
(a) M. Kurmoo, H. Kumagai, S. M. Hughes and C. J. Kepert, Inorg. Chem., 2003, 42, 4709 CrossRef PubMed
;
(b) K. S. Hagen, S. G. Nail, B. H. Huynh, A. Masello and G. Chrisyou, J. Am. Chem. Soc., 2009, 131, 7516 CrossRef CAS PubMed
;
(c) M. H. Zeng, M. C. Wu, H. Liang, Y. L. Zhou, X. M. Chen and S. W. Ng, Inorg. Chem., 2007, 46, 7241 CrossRef CAS PubMed
;
(d) L. Cañadillas-Delgado, O. Fabelo, J. A. Rodríguez-Velamazán, M. H. Lemée-Cailleau, S. A. Mason, E. Pardo, F. Lloret, J. P. Zhao, X. H. Bu, V. Simonet, C. V. Colin and J. Rodríguez-Carvajal, J. Am. Chem. Soc., 2012, 134, 19772 CrossRef PubMed
;
(e) B. Shaabani, A. A. Khandar, S. S. Kazemi, Z. Shaghaghi, A. K. Boudalis, V. Psycharis and A. Raptopoulou, Polyhedron, 2013, 49, 61 CrossRef CAS PubMed
.
-
(a) A. Rujiwatra, C. J. Kepert, J. B. Claridge, M. J. Rosseinsky, H. Kumagai and M. Kurmoo, J. Am. Chem. Soc., 2001, 123, 10584 CrossRef CAS PubMed
;
(b) E. Q. Gao, P. P. Liu, Y. Q. Wang and Q. L. Wang, Chem.–Eur. J., 2009, 1217 CrossRef CAS PubMed
;
(c) W. K. Chang, R. K. Chiang, Y. C. Jiang, S. L. Wang, S. F. Lee and K. H. Lii, Inorg. Chem., 2004, 43, 2564 CrossRef CAS PubMed
;
(d) Z. L. Huang, M. Drillon, N. Masciocchi, A. Sironi, J. T. Zhao, P. Rabu and P. Panissod, Chem. Mater., 2000, 12, 2805 CrossRef CAS
;
(e) Y. L. Zhou, M. C. Wu, M. H. Zeng and H. Liang, Inorg. Chem., 2009, 48, 10146 CrossRef CAS PubMed
.
-
(a) S. Y. Zhang, W. Shi, Y. H. Lan, N. Xu, X. Q. Zhao, A. K. Powell, B. Zhao, P. Cheng, D. Z. Liao and S. P. Yan, Chem. Commun., 2011, 47, 2859 RSC
;
(b) J. Y. Zou, W. Shi, N. Xu, L. L. Li, J. K. Tang, H. L. Gao, J. Z. Cui and P. Cheng, Chem. Commun., 2013, 49, 8226 RSC
.
-
(a) W. Shi, X. Y. Chen, N. Xu, H. B. Song, B. Zhao, P. Cheng, D. Z. Liao and S. P. Yan, Eur. J. Inorg. Chem., 2006, 4931 CrossRef CAS
;
(b) C. Chen, S. Y. Zhang, H. B. Song, W. Shi, B. Zhao and P. Cheng, Inorg. Chim. Acta, 2009, 362, 2749 CrossRef CAS PubMed
;
(c) J. Y. Zou, H. L. Gao, W. Shi, J. Z. Cui and P. Cheng, CrystEngComm, 2013, 15, 2682 RSC
;
(d) J. Y. Zou, N. Xu, W. Shi, H. L. Gao, J. Z. Cui and P. Cheng, RSC Adv., 2013, 3, 21511 RSC
;
(e) J. Y. Zou, W. Shi, N. Xu, H. L. Gao, J. Z. Cui and P. Cheng, Eur. J. Inorg. Chem., 2014, 407 CrossRef CAS
.
-
(a) Y. F. Yue, B. W. Wang, E. Q. Gao, C. J. Fang, C. He and C. H. Yan, Chem. Commun., 2007, 2034 RSC
;
(b) W. X. Zhang, W. Xue, Y. Z. Zheng and X. M. Chen, Chem. Commun., 2009, 3804 RSC
;
(c) W. X. Zhang, W. Xue and X. M. Chen, Inorg. Chem., 2011, 50, 309 CrossRef CAS PubMed
;
(d) Y. F. Yue, J. Liang, E. Q. Gao, C. J. Fang, Z. G. Yan and C. H. Yan, Inorg. Chem., 2008, 47, 6115 CrossRef CAS PubMed
;
(e) W. X. Zhang, W. Xue, J. B. Lin, Y. Z. Zheng and X. M. Chen, CrystEngComm, 2008, 10, 1770 RSC
;
(f) S. Wang, T. T. Zhao, G. H. Li, L. Wojtas, Q. S. Huo, M. Eddaoudi and Y. L. Liu, J. Am. Chem. Soc., 2010, 132, 18038 CrossRef CAS PubMed
;
(g) T. T. Zhao, X. M. Jing, J. Wang, D. M. Wang, G. H. Li, Q. S. Huo and Y. L. Liu, Cryst. Growth Des., 2012, 12, 5456 CrossRef CAS
.
-
(a) J. Vallejo, I. Castro, R. Ruiz-García, J. Cano, M. Julve, F. Lloret, G. D. Munno, W. Wernsdorfer and E. Pardo, J. Am. Chem. Soc., 2012, 134, 15704 CrossRef CAS PubMed
;
(b) S. Y. Zhang, Z. J. Zhang, W. Shi, B. Zhao, P. Cheng, D. Z. Liao and S. P. Yan, Dalton Trans., 2011, 40, 7993 RSC
.
- L. E. Hinlel, G. O. Richards and O. Thomas, J. Chem. Soc., 1937, 1432 Search PubMed
.
- M. Sheldrick, Acta Crystallogr., Sect. A: Fundam. Crystallogr., 2008, 64, 112 CrossRef PubMed
.
-
(a) V. A. Blatov, A. P. Shevchenko and V. N. Serezhkin, J. Appl. Crystallogr., 2000, 33, 1193 CrossRef CAS
. TOPOS software is available for download at http://www.topos.ssu.samara.ru;
(b)
M. O'Keeffe, O. M. Yaghi and S. Ramsden, Reticular Chemistry Structure Resourece, 2007, database available at http://rcsr.anu.edu.au/ Search PubMed
.
-
(a)
E. L. Carlin, Magnetochemistry, Springer-Verlag, 1986 Search PubMed
;
(b) K. C. Xiong, F. L. Jiang, Y. L. Gai, D. Q. Yuan, L. Chen, M. Y. Wu, K. Z. Su and M. C. Hong, Chem. Sci., 2012, 3, 2321 RSC
;
(c) L. Daumann, P. Comba, J. A. Larrabee, G. Schenk, R. Stranger, G. Cavigliasso and L. R. Gahan, Inorg. Chem., 2013, 52, 2029 CrossRef CAS PubMed
.
-
(a) M. E. Lines, J. Chem. Phys., 1971, 55, 2977 CrossRef CAS PubMed
;
(b) A. V. Palii, O. S. Reu, S. M. Ostrovsky, S. I. Klokishner, B. S. Tsukerblat, Z. M. Sun, J. G. Mao, A. V. Prosvirin, H. H. Zhao and K. R. Dunbar, J. Am. Chem. Soc., 2008, 130, 14729 CrossRef CAS PubMed
;
(c) A. V. Palii, B. S. Tsukerblat, E. Coronado, J. M. Clemente-Juan and J. J. Borrás-Almenar, J. Chem. Phys., 2003, 118, 5566 CrossRef CAS PubMed
.
-
(a) E. Q. Gao, Y. F. Yue, S. Q. Bai, Z. He and C. H. Yan, J. Am. Chem. Soc., 2004, 126, 1419 CrossRef CAS PubMed
;
(b) M. Monfort, I. R. Dra, J. Ribas and H. Stoeckli-Evans, Angew. Chem., Int. Ed., 2000, 39, 191 CrossRef CAS
.
- A. Demessence, A. Yassar, G. Rogez, L. Miozzo, S. D. Brion and P. Rabu, J. Mater. Chem., 2010, 20, 9401 RSC
.
-
(a) D. Maspoch, D. Ruiz-Molina, K. Wurst, N. Domingo, M. Cavallini, F. Biscarini, J. Tejada, C. Rovira and J. Veciana, Nat. Mater., 2003, 2, 190 CrossRef CAS PubMed
;
(b) J. Arcas, A. Hernado, J. M. Barandiarán, C. Prados, M. Vázquez, P. Marín and A. Neuweiler, Phys. Rev. B: Condens. Matter Mater. Phys., 1998, 58, 5193 CrossRef CAS
.
- X. Y. Wang, Z. M. Wang and S. Gao, Inorg. Chem., 2008, 47, 5720 CrossRef CAS PubMed
.
- M. Monfort, I. Resino, J. Ribas and S. Stoeckli-Evans, Angew. Chem., Int. Ed., 2000, 39, 191 CrossRef CAS
.
-
(a) T. Liu, Y. J. Zhang, Z. M. Wang and S. Gao, Inorg. Chem., 2006, 45, 2782 CrossRef CAS PubMed
;
(b) Z. Hulvey, B. C. Melot and A. K. Cheetham, Inorg. Chem., 2010, 49, 4594 CrossRef CAS PubMed
.
-
(a) T. H. Yang, Y. Liao, L. M. Zheng, R. E. Dinnebier, Y. H. Sua and J. Ma, Chem. Commun., 2009, 3023 RSC
;
(b) X. Y. Wang, B. L. Li, X. Zhu and S. Gao, Eur. J. Inorg. Chem., 2005, 3277 CrossRef CAS
;
(c) S. Midollini, A. Orlandini, P. Rosa and L. Sorace, Inorg. Chem., 2005, 44, 2060 CrossRef CAS PubMed
.
-
(a) P. Yin, S. Gao, L. M. Zheng and X. Q. Xin, Chem. Mater., 2003, 15, 3233 CrossRef CAS
;
(b) H. Kumagai, C. J. Kepert and M. Kurmoo, Inorg. Chem., 2002, 41, 3410 CrossRef CAS PubMed
;
(c) X. M. Zhang, Z. M. Hao, W. X. Zhang and X. M. Chen, Angew. Chem., Int. Ed., 2007, 46, 3456 CrossRef CAS PubMed
;
(d) T. K. Maji, W. Kaneko, M. Ohba and S. Kitagawa, Chem. Commun., 2005, 4613 RSC
.
-
(a) M. H. Zeng, W. X. Zhang, X. Z. Sun and X. M. Chen, Angew. Chem., Int. Ed., 2005, 44, 3079 CrossRef CAS PubMed
;
(b) Q. X. Jia, Y. Q. Wang, Q. Yue, Q. L. Wang and E. Q. Gao, Chem. Commun., 2008, 4894 RSC
;
(c) K. C. Mondal, G. E. Kostakis, Y. H. Lan, C. E. Anson and A. K. Powell, Inorg. Chem., 2009, 48, 9205 CrossRef CAS PubMed
;
(d) J. H. Yoo, J. H. Lim, S. W. Choi, H. C. Kim and C. S. Hong, Inorg. Chem., 2007, 46, 1529 CrossRef PubMed
.
Footnote |
† Electronic supplementary information (ESI) available: XRPD spectra, IR spectra, TG spectra, additional figures and magnetic properties. CCDC 909523 for 1 and 928525 for 2. For ESI and crystallographic data in CIF or other electronic format see DOI: 10.1039/c3qi00045a |
|
This journal is © the Partner Organisations 2014 |