DOI:
10.1039/C3NP70063A
(Highlight)
Nat. Prod. Rep., 2014,
31, 6-14
Natural products as starting points for the synthesis of complex and diverse compounds
Received
8th July 2013
, Accepted 4th October 2013
First published on 12th November 2013
Abstract
Covering: up to 2013
Natural products and their derivatives are used as treatments for numerous diseases. Many of these compounds are structurally complex, possessing a high percentage of sp3 hybridized carbons and multiple stereogenic centers. Due to the difficulties associated with the isolation of large numbers of novel natural products, lead discovery efforts over the last two decades have shifted toward the screening of less structurally complex synthetic compounds. While there have been many success stories from these campaigns, the modulation of certain biological targets (e.g. protein–protein interactions) and disease areas (e.g. antibacterials) often require complex molecules. Thus, there is considerable interest in the development of strategies to construct large collections of compounds that mimic the complexity of natural products. Several of these strategies focus on the conversion of simple starting materials to value-added products and have been reviewed elsewhere. Herein we review the use of natural products as starting points for the generation of complex compounds, discussing both early ad hoc efforts and a more recent systematization of this approach.
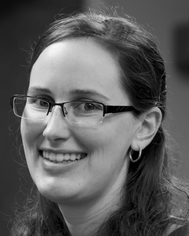 Karen C. Morrison | Karen Morrison earned her B.S. in chemistry from Harvey Mudd College in 2008 and her Ph.D. in chemistry from the University of Illinois at Urbana-Champaign in 2013. While in the lab of Professor Paul Hergenrother at UIUC, her work focused on the biological characterization of natural products and the development of stereochemically complex and structurally diverse compounds from natural products. Karen currently works with the California Council on Science and Technology in science policy. |
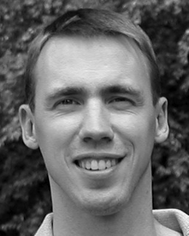 Paul J. Hergenrother | Paul Hergenrother earned his B.S. in chemistry from the University of Notre Dame in 1994, a PhD in Chemistry from the University of Texas, Austin in 1999, and spent two years as an American Cancer Society Postdoctoral Fellow at Harvard University. Professor Hergenrother started as an Assistant Professor at the University of Illinois, Urbana-Champaign in 2001, and in 2013 he was named as the Kenneth L. Rinehart Jr. Endowed Chair in Natural Products Chemistry. The Hergenrother lab uses organic compounds to define novel targets for the treatment of disease, with an emphasis on cancer and drug-resistant bacteria. |
1 Introduction
Natural products (NPs) have traditionally formed the backbone of modern drug discovery programs. The fruits of this effort include well known drugs such as paclitaxel (anticancer), artemisinin (antimalarial), daptomycin (antibacterial) and morphine (analgesic). Indeed, from the 1940s until 2010, 41% of anticancer and 65% of antibacterial small molecule drugs were either NPs or semi-synthetic derivatives of NPs.1
Despite their rich history in drug discovery, the 1990s saw a movement away from NPs and toward the screening of collections of synthetic compounds. This was due to several factors, including the increasing difficulty of identifying new NPs2,3 and the development of synthetic methods which could be used to rapidly create large collections of compounds.4,5 The libraries of organic compounds constructed in this high-throughput fashion are comprised of compounds that are easy to assemble, and thus can be synthesized via automated, combinatorial, or parallel syntheses. Such compounds tend to be structurally different to NPs: the complexity of ring systems, the percentage of carbons that are sp3 hybridized, the heteroatom content and the number of stereocenters are all markedly different in collections of synthetic compounds as compared to NPs.6 These structural variations lead to different interactions with macromolecular receptors, resulting in divergent biological activities.7–9 Based on this realization, there has been a movement to develop synthetic compounds that have complexity comparable to that of NPs. This strategy would provide compounds that represent the best of both worlds: structurally complex molecules with physicochemical properties analogous to those of NPs, accessed as rapidly as the other synthetic compounds.
The facile creation of large numbers of complex and diverse small molecules is an enormous challenge and represents one of the frontier problems in modern synthetic chemistry, medicinal chemistry, and chemical biology. Diversity-oriented synthesis (DOS), in which complex compounds are rapidly assembled from simple starting materials,10–12 has been widely adopted, and compounds generated from DOS efforts have been identified as hits in a diverse array of screens.13–16 Other strategies, including function-oriented synthesis (FOS),17 biology-oriented synthesis (BiOS),18 biosynthetic engineering,19 and differential functionalization of the periphery of NPs20,21 also show promise. These tactics are outside the scope of this Highlight and have been reviewed elsewhere.10,11,16–18
This Highlight will focus on a recent area of development aimed at creating complex small molecules directly from NPs. NPs in particular are exceptional starting points due to the high abundance and structural variation of many common classes of NPs; additionally, NPs provide an excellent framework for site selective and stereoselective transformations. This area of research is aided by historic work on degradation reactions performed on NPs during their structural elucidation; such degradation reactions can be used to access complex scaffolds related to NPs. In using NPs as the starting points for complex molecule synthesis, synthetic chemists can add diversity to the complexity installed by Nature. The examples covered in this Highlight fall into two broad categories: the use of a single reactive group on an NP to alter the structure (Fig. 1A), and the use of multiple reactive groups (Fig. 1B).
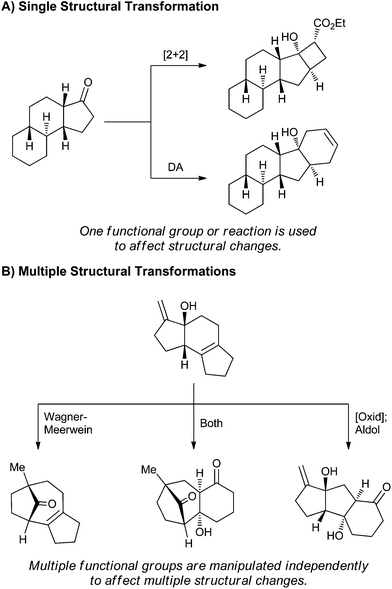 |
| Fig. 1 Classes of structural transformations for modifying natural products. | |
The earliest ad hoc attempts to modulate the structures of NPs utilized molecules with a single reactive group. The application of a single transformation class then results in multiple different products (Fig. 1A). As will be discussed in this Highlight, the forerunners in this area generated multiple products in one reaction, leading to difficult separations or modest yields.22 Efforts in the mid 2000s employed reagent control to afford products in a selective fashion,23,24 and work since 2011 has systematized this process to clearly and predictably create complex small molecules.25–28
In contrast to the single transformation model, use of multiple structural transformations (Fig. 1B) relies on NPs with rich functional diversity. Initial investigations focused on the elaboration of NPs for total syntheses or to optimize the activity or selectivity of the original NP through structural modifications.29,30 Recent efforts in this area have formalized this process of multiple structural transformations to produce several highly complex NPs.31 Application of this method to other NPs should allow for the construction of multiple classes of complex small molecules. Further maturation of the field from creating scaffolds to generating small libraries based on these novel structures will ultimately allow for the generation of large numbers of complex and diverse compounds.
2 Single structural transformations
2.1 Lathyrane diterpenes
The lathyrane diterpenes are a class of NPs isolated from the seeds of caper spurge Euphorbia lathyris (Fig. 2). These complex tricyclic small molecules can be recovered in multi-gram quantities (0.25% yield by mass),32 making them useful starting materials for conversion into other complex compounds. Although these NPs contain multiple functional groups, the reactive cyclopropane enone and either the epoxide (L1, 1) or the exocyclic olefin (L3, 2) serve as the key reactive groups that drive the transformations of these NPs. In a 2001 report by Sterner and coworkers,22 L1 and L3 were modified, on a gram-scale via acid-catalyzed reactions, to a variety of new structures. In both cases, the opening of the cyclopropane ring is stabilized by the enone, and subsequent collapse of the extended charged species results in 2–3 polycyclic structures from each transformation, generally in comparable yields (for example, 3 and 4 from 1, and 5 and 6 from 2). While stereoselectivity is not attained, this dramatic restructuring of the macrocycle is impressive and achieves yields that are synthetically useful. Sterner and coworkers also identified additional transformation products resulting from terpenes in which the enone had been reduced to an allylic alcohol (not shown).22
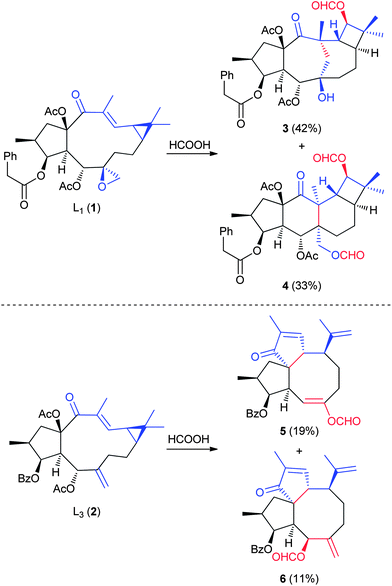 |
| Fig. 2 Structural transformation of lathyrane diterpenes via acid-catalyzed rearrangements. | |
2.2 Isosteviol and steviol
Isosteviol (7) and steviol (8) (Fig. 3) are diterpenes derived from the readily-available trisaccharide natural sweetener stevioside. While both 7 and 8 are mostly chemically inert, they possess a ketone or alkene, respectively, allowing for diversification of the NPs. Georg and coworkers used a systematic application of the Beckmann rearrangement of protected versions of both molecules (9 and 10) to achieve a variety of nitrogen insertion (11–12, 14–15) and rearrangement products (13 and 16), as shown in Fig. 3.25 Following oxime or imine formation, regioselective ring expansions and fragmentations result in six distinct molecular frameworks.
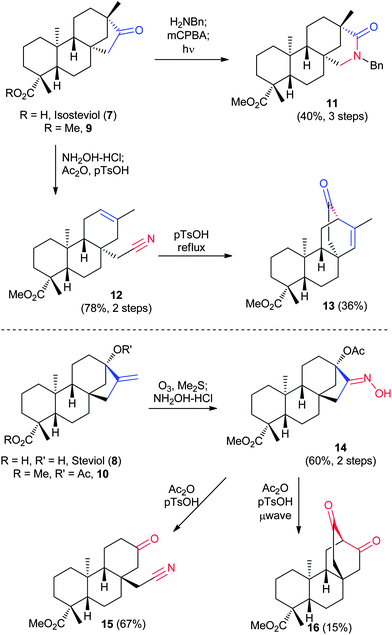 |
| Fig. 3 Beckmann rearrangements and fragmentations of isosteviol and steviol. | |
2.3
R-(+)-Sclareolide
The sesquiterpene R-(+)-sclareolide (17) (Fig. 4) is a common NP used in the fragrance industry and in chemical synthesis as a commercial chiral pool starting material. Apart from the lactone, 17 is chemically inert, making it an excellent candidate for single transformation structural changes. In 2003, de la Torre and Garcia devised an elimination–[2+2] photocycloaddition reaction scheme to transform 17 into two highly reactive cyclobutanes (21 and 22, Fig. 4).23 Unlike in the modification of 1/2 and 7/8, sclareolide is elaborated into several diverse scaffolds through a reagent control strategy. For example, addition of either allylmagnesium chloride or lithiated cyclopentene to alkene 18, followed by photochemical activation, results in cyclobutanes 21 (via19) and 22 (via20). The combination of scaffold diversification and derivatization offers an interesting opportunity for building polycyclic systems.24
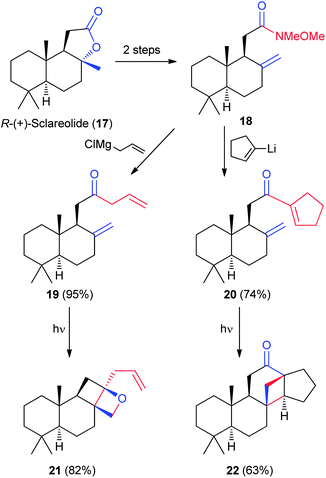 |
| Fig. 4 Elimination and light-catalyzed ring fusions from R-(+)-sclareolide. | |
2.4 Bryonolic acid
Bryonolic acid (23, Fig. 5), a pentacyclic triterpenoid, is found in the sprouts of the common zucchini and is isolable in multi-gram quantities (1.34% yield by mass),33 and contains a unique double bond fusion between the steroidal B and C rings. A protected version of bryonolic acid (24) renders this alkene the primary reactive site. Building from earlier ad hoc work with lanosterol, Tochtrop and coworkers systematically explored the oxidative cleavage of bryonolic acid across the central B/C rings (Fig. 5).26,27 Treatment with ruthenium chloride yields three distinct products (25–27). The newly created macrocycle (25) can undergo a transannular aldol fusion to give products such as 28 and 29 in varying ratios, depending on the conditions used.26,27 By altering the oxidation patterns, as well as the conditions used to form additional rings across the core of bryonolic acid, several unique scaffolds can be formed.
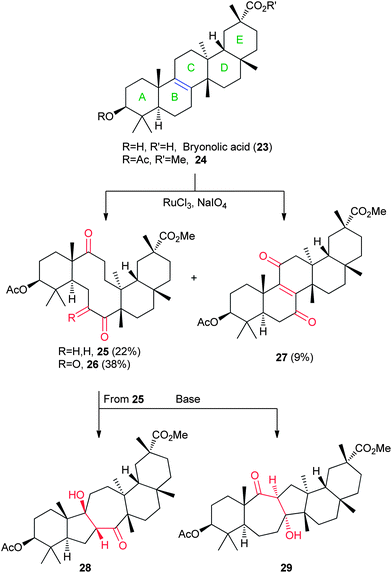 |
| Fig. 5 Oxidative cleavage and transannular fusion from bryonolic acid. | |
2.5 Fumagillol
The NP fumagillin, derived from Aspergillus fumigatus, is easily obtained by fermentation and is further hydrolyzed to the diepoxide fumagillol on a multi-gram scale (30, Fig. 6).34,35 The epoxides can be chemically functionalized separately from the alkene and alcohol. Additionally, modifications to the alkene and alcohol in later synthetic steps enable additional points for derivatization. In work done by Porco, Snyder, and coworkers, nucleophilic addition to the disubstituted epoxide, followed by Lewis acid-directed cyclization, forms two divergent bicyclic systems with varied substitution at the amine (31 and 32, R = aryl, benzyl, or alkyl).28 The choice of Lewis acid directs the formation of either the five- or six-membered ring with selectivities ranging from 66
:
32 to >20
:
1 for the isolated product. Careful selection of the reactive amine promotes further cyclizations with the trisubstituted alkene, such as lactone formation with D-phenylalanine to form 33 or hydroxylalkoxylation with ethylaniline to form 34. Together, this work is the most general example of a single chemical transformation generating multiple scaffolds.
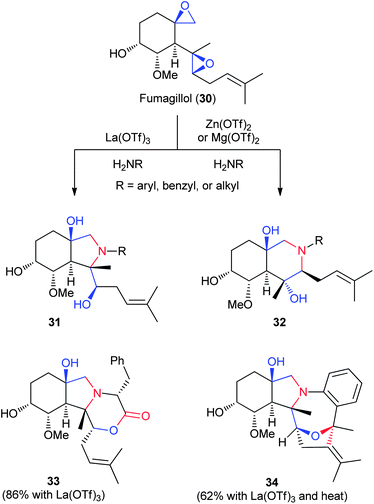 |
| Fig. 6 Transformation of fumagillol via catalyst-selective nucleophilic addition and cyclization. | |
2.6 Summary of single transformations
In the five examples described above, transformations are applied to an NP, resulting in dramatically different scaffolds. However, there are a limited number of structures that can be made, restricting both the diversity and number of compounds that can be created. On average, fewer than twenty compounds were synthesized in each of the previous five examples, and most of these contain similar or identical cores. As a result, transformations that can be applied independently to multiple regions of an NP offer new opportunities for complex library development.
3 Multiple structural transformations
3.1 Zerumbone
The cyclic sesquiterpene zerumbone (35, Fig. 7), isolated from the rhizome of wild ginger in 0.3–0.4% yield by mass,36 is one of the earliest examples of the application of multiple structural transformations in the generation of novel complex scaffolds.29 In work by Kitayama and coworkers, derivatives of 35 were created and elaborated to more complex NP-like scaffolds. By capitalizing on the different steric and electronic environments of the three alkenes, selective brominations, cyanations, and cleavages are all possible in order to synthesize a tricyclic ether (36),37 a transannular cyclopropane (37),36 and a linear product (38),38 depending on the order of the reactions. Each of these reaction sequences dramatically alters the original structure of zerumbone and relies on a variety of different synthetic transformations. However, further refinements would be necessary in order to fully apply this NP to the construction of large collections of complex molecules.
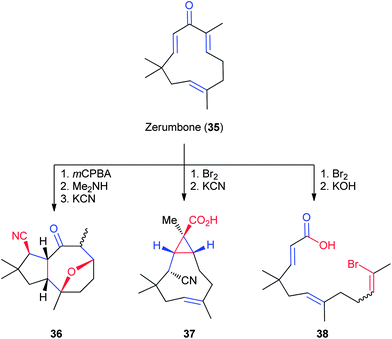 |
| Fig. 7 Multiple structural transformations from zerumbone in 2–3 steps. | |
3.2 Naltrexone
The synthetic opiate naltrexone (39, Fig. 8), which is structurally similar to the NP morphine, is used clinically to treat alcohol and opioid dependence. The Nagase group has identified numerous transformations that reorganize the ring structure of naltrexone in order to enhance the safety and selectivity of naltrexone for specific opioid receptors.30 From a synthetic standpoint, however, these transformations create dramatically altered products that could be tested for novel biological activity. These include elimination of the D-ring (40)39 and formation of additional ether rings through a D-ring cleaved intermediate (41 and 42).39,40 Transformation of the C-ring to a diene (43) enables a further Diels–Alder reaction, followed by further rearrangements, to afford cyclopropane δ-lactone 44.41,42 Unfortunately, there are relatively few positions for further derivatization of these scaffolds, which makes the use of this chemistry for library generation more difficult.
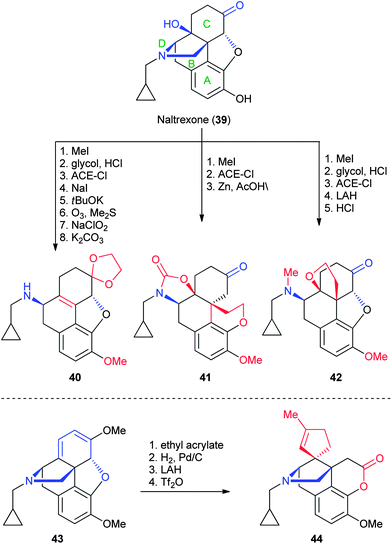 |
| Fig. 8 Multiple structural transformations from naltrexone in three or more steps. | |
3.3 Gibberellic acid
Gibberellic acid (45, Fig. 9) is a tetracyclic diterpene hormone used agriculturally on the ton scale to promote plant germination and growth.43,44 As an NP for transformation into complex and diverse structures, gibberellic acid contains a number of advantageous features that enable several divergent transformations across all the ring systems. These include the secondary allylic alcohol, A-ring lactone, and tertiary allylic alcohol on the C/D rings. Beginning from several classical degradation reactions using acid, base or hydrazine, Hergenrother and coworkers synthesized 19 divergent structures in 3–5 steps from the parent NP (Fig. 9).31 These included acetalization of the A-ring (46), aromatization of the A-ring with ring expansion of the D-ring (47), and cleavage of the B-ring to the diketone (48). Several other structures from gibberellic acid were further elaborated into small libraries, resulting in >45 total compounds synthesized, validating this NP as a useful starting point for the creation of large numbers of complex molecules.
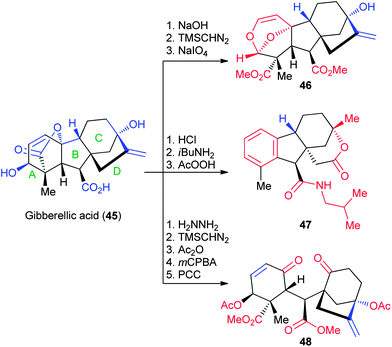 |
| Fig. 9 Multiple structural transformations of gibberellic acid in 3–5 steps. | |
3.4 Adrenosterone
Adrenosterone (49, Fig. 10), which is naturally produced in the adrenal cortex and is commercially available in gram quantities, contains three ketones. Each ketone has a unique pattern of reactivity, allowing for selective functionalization and manipulation of each ring. Hergenrother and coworkers applied a variety of transformations, including oxidative cleavages and Baeyer–Villiger oxidation (50), Beckmann fragmentations (51), and epoxidations (52), to synthesize 18 complex scaffolds from adrenosterone (Fig. 10).31 A total of 70 complex compounds based on several scaffolds were prepared from adrenosterone in sufficient quantities for biological testing.
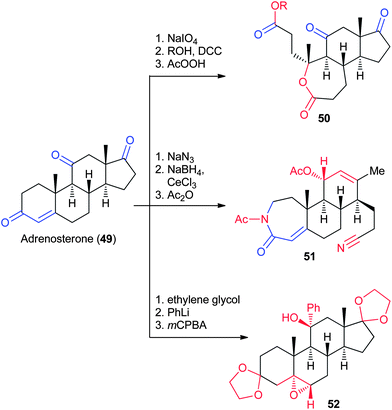 |
| Fig. 10 Multiple structural transformations of adrenosterone in 3 steps. | |
3.5 Quinine
The readily available NP quinine (53, Fig. 11) has been used for a variety of purposes, including as an antimicrobial and a bitter agent for tonics.45 Quinine also possesses structural features that prime this NP for several distinct structural transformations, including a tertiary amine, a secondary alcohol and an olefin. Hergenrother and coworkers utilized these functional groups to promote selective cleavage and rearrangement to the S-alkyl thiocarbamate (54), Hofmann-type elimination and ring closing metathesis (55), and Grignard addition followed by hemiaminal ether formation (56) on quinine (Fig. 11).31 Altogether, 12 distinct scaffolds were constructed, and thiocarbamate 54 was further functionalized to produce over thirty derivatives.
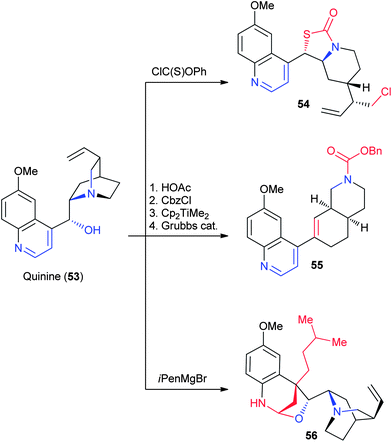 |
| Fig. 11 Multiple structural transformations of quinine in 1–4 steps. | |
3.6 Summary of multiple transformations
In the five examples described above, multiple classes of structural transformations are applied to each NP or NP scaffold. Although the earliest examples of this approach did not incorporate positions for library synthesis, the recent systematization of this approach and its application to gibberellic acid, adrenosterone and quinine has led to the construction of >160 complex and diverse compounds.31 This work suggests that NPs could serve as efficient starting materials for the creation of libraries of complex molecules.
3.7 Outlook on the field
Populating compound screening collections with complex and diverse members is a challenging endeavor. Numerous synthetic strategies, including DOS,16,46,47 FOS,17 and BiOS,18 have been proposed to address this need. In this Highlight, two developing strategies using NPs for the generation of complex and diverse compounds have been covered, whereby NPs are rearranged into distinct scaffolds amenable to library construction. The first strategy utilizes a single class of reaction, such as epoxide opening or acid catalysis, to give different types of products, depending on the conditions. This approach of transforming NPs has provided a number of interesting and useful scaffolds, but it typically affects only one portion of the molecule and generally utilizes relatively unfunctionalized NPs to ensure selectivity. A second class of transformations is the systematic reorganization of multiple rings or functional groups on an NP in order to affect the entire molecule. Recent work with gibberellic acid, adrenosterone, and quinine has expanded this strategy to the development of up to 19 scaffolds from one NP and the synthesis of small libraries to access >160 compounds.
Similar approaches to transform core structures could be applied to numerous NPs, although several criteria should ideally be met for these approaches to be successful. First, the NP must be available in sufficient quantity, either commercially or by isolation, to enable the generation of novel structures on a large enough scale for further biological analysis. Second, the NP should contain functional groups amenable to structural transformations, rather than derivatization alone. For example, a ketone offers opportunities for Baeyer–Villiger and Beckmann ring expansions (as shown with adrenosterone, steviol, and isosteviol) or ring contractions (as with the lathyrane terpenes and zerumbone), whereas a carboxylic acid lends itself more easily to simple amidations or esterifications (as with the functionalization of gibberellic acid or adrenosterone compounds). Similarly, epoxides are useful functional groups for forming new rings or contracting macrocycles, as highlighted by the lathyrane diterpenes and fumagillol. Alkenes provide a versatile handle for rearranging NPs, as they can be used for forming new rings or cleaving existing rings. Impressively, isolated alkenes and conjugated ketones were used in the transformation of each of the NPs discussed in this Highlight. Strained ring systems and heterocycles provide further handles for manipulating NPs.
The full application of these approaches for the creation of screening collections populated by large numbers of complex and diverse compounds necessitates the synthesis of scores of derivatives of each scaffold. Derivatization further allows for the tailoring of chemical properties, such as size, shape, and polarity, for the generation of focused libraries. As a result, the selection of NPs containing sites for diversification or the installation of new functional groups during the transformation process is ideal. The use of natural products as starting materials is a valuable method for the synthesis of collections of highly complex and diverse compounds.
4 Acknowledgements
We thank the Office of Naval Research (N00014-09-1-0249) and the University of Illinois for support of this work. K.C.M. is a National Science Foundation predoctoral fellow and a Robert C. and Carolyn J. Springborn graduate fellow.
5 References
- J. Newman and G. M. Cragg, Natural Products as Sources of New Drugs over the 30 Years from 1981 to 2010, J. Nat. Prod., 2012, 75, 311–335 CrossRef PubMed.
- R. H. Baltz, Renaissance in antibacterial discovery from actinomycetes, Curr. Opin. Pharmacol., 2008, 8, 557–563 CrossRef CAS PubMed.
- J. W. Li and J. C. Vederas, Drug discovery and natural products: end of an era or an endless frontier?, Science, 2009, 325, 161–165 CrossRef PubMed.
- T. W. Cooper, I. B. Campbell and S. J. Macdonald, Factors determining the selection of organic reactions by medicinal chemists and the use of these reactions in arrays (small focused libraries), Angew. Chem., Int. Ed., 2010, 49, 8082–8091 CrossRef CAS PubMed.
- M. E. Welsch, S. A. Snyder and B. R. Stockwell, Privileged scaffolds for library design and drug discovery, Curr. Opin. Chem. Biol., 2010, 14, 347–361 CrossRef CAS PubMed.
- H. Lachance, S. Wetzel, K. Kumar and H. Waldmann, Charting, navigating, and populating natural product chemical space for drug discovery, J. Med. Chem., 2012, 55, 5989–6001 CrossRef CAS PubMed.
- M. M. Hann, A. R. Leach and G. Harper, Molecular complexity and its impact on the probability of finding leads for drug discovery, J. Chem. Inf. Model., 2001, 41, 856–864 CrossRef CAS PubMed.
- A. A. Shelat and R. K. Guy, Scaffold composition and biological relevance of screening libraries, Nat. Chem. Biol., 2007, 3, 442–446 CrossRef CAS PubMed.
- J. Hert, J. J. Irwin, C. Laggner, M. J. Keiser and B. K. Shoichet, Quantifying biogenic bias in screening libraries, Nat. Chem. Biol., 2009, 5, 479–483 CrossRef CAS PubMed.
- S. L. Schreiber, Target-oriented and diversity-oriented organic synthesis in drug discovery, Science, 2000, 287, 1964–1969 CrossRef CAS.
- M. R. Spaller, M. T. Burger, M. Fardis and P. A. Bartlett, Synthetic strategies in combinatorial chemistry, Curr. Opin. Chem. Biol., 1997, 1, 47–53 CrossRef CAS.
- J. Cui, J. Hao, O. A. Ulanovskaya, J. Dundas, J. Liang and S. A. Kozmin, Creation and manipulation of common functional groups en route to a skeletally diverse chemical library, Proc. Natl. Acad. Sci. U. S. A., 2011, 108, 6763–6768 CrossRef CAS PubMed.
- W. R. Galloway, A. Isidro-Llobet and D. R. Spring, Diversity-oriented synthesis as a tool for the discovery of novel biologically active small molecules, Nat. Commun., 2010, 1, 80 CrossRef PubMed.
- P. A. Clemons, N. E. Bodycombe, H. A. Carrinski, J. A. Wilson, A. F. Shamji, B. K. Wagner, A. N. Koehler and S. L. Schreiber, Small molecules of different origins have distinct distributions of structural complexity that correlate with protein-binding profiles, Proc. Natl. Acad. Sci. U. S. A., 2010, 107, 18787–18792 CrossRef CAS PubMed.
- G. I. Schaefer, J. R. Perez, J. R. Duvall, A. F. Shamji and S. L. Schreiber, Discovery of small-molecule modulators of the Sonic Hedgehog pathway, J. Am. Chem. Soc., 2013, 135, 9675–9680 CrossRef CAS PubMed.
- C. J. O'Connor, H. S. Beckmann and D. R. Spring, Diversity-oriented synthesis: producing chemical tools for dissecting biology, Chem. Soc. Rev., 2012, 41, 4444–4456 RSC.
- P. A. Wender, V. A. Verma, T. J. Paxton and T. H. Pillow, Function-oriented synthesis, step economy, and drug design, Acc. Chem. Res., 2008, 41, 40–49 CrossRef CAS PubMed.
- S. Wetzel, R. S. Bon, K. Kumar and H. Waldmann, Biology-oriented synthesis, Angew. Chem., Int. Ed., 2011, 50, 10800–10826 CrossRef CAS PubMed.
- B. A. Pfeifer, S. J. Admiraal, H. Gramajo, D. E. Cane and C. Khosla, Biosynthesis of complex polyketides in a metabolically engineered strain of E. coli, Science, 2001, 291, 1790–1792 CrossRef CAS PubMed.
- H. E. Pelish, N. J. Westwood, Y. Feng, T. Kirchhausen and M. D. Shair, Use of biomimetic diversity-oriented synthesis to discover galanthamine-like molecules with biological properties beyond those of the natural product, J. Am. Chem. Soc., 2001, 123, 6740–6741 CrossRef CAS.
- B. C. Goess, R. N. Hannoush, L. K. Chan, T. Kirchhausen and M. D. Shair, Synthesis of a 10,000-membered library of molecules resembling carpanone and discovery of vesicular traffic inhibitors, J. Am. Chem. Soc., 2006, 128, 5391–5403 CrossRef CAS PubMed.
- G. Appendino, G. C. Tron, T. Jarevang and O. Sterner, Unnatural natural products from the transannular cyclization of lathyrane diterpenes, Org. Lett., 2001, 3, 1609–1612 CrossRef CAS PubMed.
- M. C. de la Torre, I. Garcia and M. A. Sierra, Photochemical access to tetra- and pentacyclic terpene-like products from R-(+)-sclareolide, J. Org. Chem., 2003, 68, 6611–6618 CrossRef CAS PubMed.
- M. C. de la Torre, I. Garcia and M. A. Sierra, Diversity oriented synthesis of hispanane-like terpene derivatives from (R)-(+)-sclareolide, Chem.–Eur. J., 2005, 11, 3659–3667 CrossRef CAS PubMed.
- O. E. Hutt, T. L. Doan and G. I. Georg, Synthesis of skeletally diverse and stereochemically complex library templates derived from isosteviol and steviol, Org. Lett., 2013, 15, 1602–1605 CrossRef CAS PubMed.
- V. A. Ignatenko, Y. Han and G. P. Tochtrop, Molecular library synthesis using complex substrates: expanding the framework of triterpenoids, J. Org. Chem., 2013, 78, 410–418 CrossRef CAS PubMed.
- V. A. Ignatenko and G. P. Tochtrop, Approach for Expanding Triterpenoid Complexity via Divergent Norrish-Yang Photocyclization, J. Org. Chem., 2013, 78, 3821–3831 CrossRef CAS PubMed.
- B. R. Balthaser, M. C. Maloney, A. B. Beeler, J. A. Porco Jr and J. K. Snyder, Remodelling of the natural product fumagillol employing a reaction discovery approach, Nat. Chem., 2011, 3, 969–973 CrossRef CAS.
- T. Kitayama, Attractive reactivity of a natural product, zerumbone, Biosci., Biotechnol., Biochem., 2011, 75, 199–207 CrossRef CAS.
- H. Nagase and H. Fujii, Synthesis of novel basic skeletons derived from naltrexone, Top. Curr. Chem., 2011, 299, 187–237 CrossRef CAS.
- R. W. Huigens III, K. C. Morrison, R. W. Hicklin, T. A. Flood Jr, M. F. Richter and P. J. Hergenrother, A ring-distortion strategy to construct stereochemically complex and structurally diverse compounds from natural products, Nat. Chem., 2013, 5, 195–202 CrossRef PubMed.
- G. Appendino, G. C. Tron, G. Cravotto, G. Palmisano and J. Jakupovic, An expeditious procedure for the isolation of ingenol from the seeds of euphorbia lathyris, J. Nat. Prod., 1999, 62, 76–79 CrossRef CAS PubMed.
- E. C. Barker, T. N. Gatbonton-Schwager, Y. Han, J. E. Clay, J. J. Letterio and G. P. Tochtrop, Bryonolic acid: a large-scale isolation and evaluation of heme oxygenase 1 expression in activated macrophages, J. Nat. Prod., 2010, 73, 1064–1068 CrossRef CAS PubMed.
-
F. R. Hanson; T. E. Eble, Fumagillin and preparation, US Patent, 2652356, 1950.
- D. S. Tarbell, R. M. Carman, D. D. Chapman, S. E. Cremer, A. D. Cross, K. R. Huffman, M. Kunstmann, N. J. McCorkindale, J. G. McNally, A. Rosowsky, F. H. L. Varino and R. L. West, The chemistry of fumagillin, J. Am. Chem. Soc., 1961, 83, 3096–3113 CrossRef CAS.
- T. Kitayama, T. Okamoto, R. K. Hill, Y. Kawai, S. Takahashi, S. Yonemori, Y. Yamamoto, K. Ohe, S. Uemura and S. Sawada, Chemistry of Zerumbone. 1. Simplified Isolation, Conjugate Addition Reactions, and a Unique Ring Contracting Transannular Reaction of Its Dibromide, J. Org. Chem., 1999, 64, 2667–2672 CrossRef CAS PubMed.
- T. Kitayama, T. Yokoi, Y. Kawai, R. K. Hill, M. Morita, T. Okamoto, Y. Yamamoto, V. V. Fokin, K. B. Sharpless and S. Sawada, The chemistry of zerumbone. Part 5: Structural transformation of the dimethylamine derivatives, Tetrahedron, 2003, 59, 4857–4866 CrossRef CAS.
- T. Kitayama, R. Iwabuchi, S. Minagawa, F. Shiomi, J. Cappiello, S. Sawada, R. Utsumi and T. Okamoto, Unprecedented olefin-dependent histidine-kinase inhibitory of zerumbone ring-opening material, Bioorg. Med. Chem. Lett., 2004, 14, 5943–5946 CrossRef CAS PubMed.
- H. Fujii, S. Imaide, A. Watanabe, K. Yoza, M. Nakajima, K. Nakao, H. Mochizuki, N. Sato, T. Nemoto and H. Nagase, A double decarboxylation reaction of an oxazolidinone and carboxylic Acid: its application to the synthesis of a new opioid lead compound, J. Org. Chem., 2010, 75, 995–998 CrossRef CAS PubMed.
- S. Imaide, H. Fujii, A. Watanabe, T. Nemoto, M. Nakajima, K. Nakao, H. Mochizuki and H. Nagase, Investigation of Beckett–Casy model 1: synthesis of novel 16,17-seco-naltrexone derivatives and their pharmacology, Bioorg. Med. Chem. Lett., 2010, 20, 1055–1058 CrossRef CAS PubMed.
- H. Fujii, Y. Watanabe, Y. Osa, T. Nemoto, N. Sato and H. Nagase, Novel rearrangement reaction of a 6,14-endoethanomorphinan derivative to a benzomorphan derivative, Tetrahedron, 2009, 65, 4808–4813 CrossRef CAS PubMed.
- H. Fujii, M. Narita, H. Mizoguchi, M. Murachi, T. Tanaka, K. Kawai, L. F. Tseng and H. Nagase, Drug design and synthesis of epsilon opioid receptor agonist: 17-(cyclopropylmethyl)-4,5alpha-epoxy-3,6beta-dihydroxy-6,14-endoethenomorphinan- 7alpha-(N-methyl-N-phenethyl)carboxamide (TAN-821) inducing antinociception mediated by putative epsilon opioid receptor, Bioorg. Med. Chem., 2004, 12, 4133–4145 CrossRef CAS PubMed.
- P. J. Curtis and B. E. Cross, Gibberellic acid. A new metabolite from the culture of filtrates of Gibberella fujikuroi., Chem. Ind., 1954, 1066 CAS.
- C. Rodrigues, L. P. Vandenberghe, J. de Oliveira and C. R. Soccol, New perspectives of gibberellic acid production: a review, Crit. Rev. Biotechnol., 2012, 32, 263–273 CrossRef CAS PubMed.
-
C. E. Song, Cinchona Alkaloids in Synthesis and Catalysis. Wiley: 2009 Search PubMed.
- M. D. Burke, E. M. Berger and S. L. Schreiber, A synthesis strategy yielding skeletally diverse small molecules combinatorially, J. Am. Chem. Soc., 2004, 126, 14095–14104 CrossRef CAS PubMed.
- D. S. Tan, Diversity-oriented synthesis: exploring the intersections between chemistry and biology, Nat. Chem. Biol., 2005, 1, 74–84 CrossRef CAS PubMed.
|
This journal is © The Royal Society of Chemistry 2014 |