DOI:
10.1039/C3NJ01008B
(Focus)
New J. Chem., 2014,
38, 22-27
Filling carbon nanotubes for nanobiotechnological applications
Received
(in Montpellier, France)
26th August 2013
, Accepted 20th November 2013
First published on 22nd November 2013
Abstract
With the great development in new filling methodologies for preparing endohedral carbon nanotubes, encapsulation strategies employing biomedically relevant molecular guests have emerged rapidly in recent years. All of these hybrid nanomaterials feature distinct properties and potential applications depending on both the chemical nature and spatial arrangement of the encapsulated molecular guests. In this focus article, we discuss the most significant examples in which carbon nanotube (CNTs) hybrids, filled with suitable molecular species, are used for biomedical applications. CNTs containing strongly emitting molecules hold great promises for diagnostic devices, whereas those filled with radioactive species and magnetically-active nanoparticles are attracting considerable attention for theranostic applications. Examples describing the use of the CNTs' tubular cavity as an active reservoir for the controlled release of drugs are also discussed.
1. Potential multifunctionality of CNTs in nanomedicine
CNTs are a unique class of nanomaterials that show remarkable structural and physicochemical properties, such as the presence of a hollow cavity, high thermal and electrical conductivity, mechanical strength and in some instances peculiar optical absorption features.1 These properties make CNTs useful candidates for biomedical engineering,2 drug-delivery and stimuli-responsive cancer theranostics.3
Thanks to their cylindrical structure, CNTs are ideal substrates for both covalent and non-covalent immobilization of bioactive species on their external surface (the exohedral functionalization route) or by filling their internal hollow cavity (the endohedral functionalization strategy). Proteins, lipids, nucleic acids and drugs have been efficiently exohedrally and endohedrally coupled to CNTs yielding a new family of theranostic tools.4,5 In fact, a great number of studies dealt with both in vitro and in vivo cellular targeting of exohedrally functionalized CNT derivatives to exploit their intrinsic physical properties such as NIR absorption or Raman scattering for bioimaging6 and light-7 or radiofrequency-induced8 heating for thermal ablation of cancer cells.
2. Encapsulation strategies
As well-established in classical supramolecular chemistry with tailored self-assembled cages,9 cavity-confinement can be a useful approach to isolate functional molecules or nanostructures from an external environment, which otherwise would be unstable, highly reactive, difficult to prepare or simply toxic.10 Since Luzzi's seminal work on the first CNTs filled with C60 (C60@CNTs),11 it has been largely shown that the inner tubular space of CNTs can behave as an active host to confine a large variety of molecular guests.12 By ex situ (through the open tips)12–14 or in situ (during the nanotube synthesis) insertion, organic or inorganic nanostructures could be confined within the CNTs' cavity, with the graphitic sheets being shields for the inner phase and a reactive surface for the exohedral functionalization through non-covalent and covalent approaches. Endohedral functionalization of CNTs was achieved soon after their discovery, when liquid metals such as Pb could be encapsulated by capillary forces.15 Following, other metal nanoparticles,16 salts,17 and organic compounds (dyes such as β-carotene18 or phthalocyanines,19 coronene,20 metallocenes21 and carbon nanostructures such as graphene22 and C60 derivatives23) have been successfully encapsulated inside CNTs through different routes, showing how CNTs can easily act as versatile molecular containers or as nanoscale reaction vessels.24
Several strategies can be used for filling CNTs according to the fillers' physicochemical properties and stability. Among the different encapsulation routes, one can classify the methods following the heating conditions (Fig. 1). High temperature processes include: (i) chemical vapor deposition (CVD) for inserting metal phases25 (Fe, Co, Ni, Sn, Ga and In at Tfilling > 500 °C);16,25 (ii) melting of pure metals (Tfilling > Tmelting) and subsequent capillary insertion into CNTs' cavities26 (CNTs are wettable by liquids with a surface tension below 100–200 mN m−1 and thus could be filled through the open ends);27 (iii) gas phase insertion–polymerization (Tfilling = 400–500 °C) of polycyclic aromatic compounds, to produce graphene nanoribbons inside CNTs;28 and (iv) in situ thermal decomposition of organometallic precursors29 (Tfilling = 100–400 °C) leading to nanoparticles.30 Some of these methods were revealed to be very useful for the production of metal-filled CNTs with magnetic properties exploitable for theranostic purposes.31 To preserve the structural integrity of biomolecules, milder filling strategies (i.e., working at r.t.) have been also developed taking advantage of the: (i) supercritical CO2 extraction;32 (ii) slow concentration of protein solutions;33 and (iii) through “nano-extraction” and “nano-condensation” methods.13 In the nano-extraction methodology, guest molecules have to display strong affinity to CNTs and be poorly solvated in the medium.
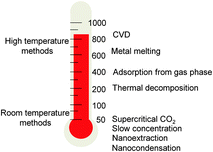 |
| Fig. 1 Classification of CNTs filling methods according to the used T. | |
3. Filling CNTs for bioimaging applications
Contrast agents (CAs) play a prominent role in magnetic resonance imaging (MRI), since annually about 30% of the MRI procedures performed worldwide employ CAs.34 Lanthanide Gd3+ ion is one of the most used species in MRI CAs, as a consequence of both its symmetric electronic ground state (8S7/2) and very large magnetic moment (μ2 = 63 μB2).35 However, aquated Gd3+ ions are toxic and they have to be employed in a sequestered form, such as encapsulated36 or chelated35 species. In this respect, Wilson and co-workers have used ultra-short single-walled CNTs (US-tubes), obtained following the fluorination/pyrolysis procedure reported by Margrave and co-workers,37 as “nanocapsules” for Gd3+ ions.38,39 The US-tubes were loaded by soaking under sonication an aqueous solution of GdCl3, allowing the lanthanide ions to be introduced through both the end-of-tube openings and the sidewall defects. High-resolution transmission electron microscopy (HRTEM) imaging coupled to energy dispersive X-ray spectroscopy (EDS) confirmed the encapsulation of the Gd3+ ions in the hybrid Gd3+@US-tube material. For the magnetic fields typically used in clinical imaging, the relaxivity of the Gd3+@US-tubes probe was extraordinarily greater than that of the currently employed CAs at 20–60 MHz (170 mM−1 s−1vs. 4.0 mM−1 s−1, nearly 40 times larger), and even more pronounced at very low fields such as 0.01 MHz (635 mM−1 s−1vs. 7.0 mM−1 s−1, approximately 90 times larger). The relationship between structural basis and high performance as an MRI contrast agent has recently been reported.40 A recent example by the same group showed the applicability of the Gd3+@US-tubes for imaging mesenchymal stem cells41 and as pH smart probes, under MRI.42 The same group has later reported that nitroxide radicals like 2,2,6,6-tetramethylpiperidine 1-oxyl (TEMPO) derivatives can be efficiently confined in US-tubes and used as high performance MRI contrast agents.43
Radiocontrast agents, such as 125I− and I2 are very effective CAs for imaging (both) and therapeutic applications (125I−), such as for the systemic radiotherapy of thyroid cancer.44 Wilson and co-workers firstly took advantage of US-tubes to confine and retain such species for bio-applications. Initially, they demonstrated that 125I− can be adsorbed readily from an aqueous solution on to the US-tubes, showing extremely low 125I− wash-off rates.45 Later, they were able to load I2 inside US-tubes equipped exohedrally with serinol amide, carboxyl or polyethyleneglycol (PEG) moieties after malonate ester reaction.46 Raman spectroscopy, X-ray photoelectron spectroscopy (XPS) and atomic force microscopy (AFM) were used to assess both the degree of functionalization and exfoliation of the US tubes, which became more opaque as X-ray-CAs than the reference samples under micro-computed tomography (CT) evaluation.
Taking advantage of the high-temperature closing reaction of the single-walled CNTs (SWCNTs) tips and of the molten-phase capillary wetting technique,47 Davis, Kostarelos, Tobias and co-workers reported the first example of filled nanotubes with molten radioactive Na125I, which yielded capped Na125I@SWCNTs hybrids.48 In this way, the excess of non-encapsulated salts could be easily removed with repeated washing cycles. CuBr, NaI or Na125I salts could be also loaded inside steam-purified and shortened SWCNTs,49 creating nanocapsules free of external material which could be easily derivatized through the 1,3-dipolar cycloaddition reaction of azomethyne ylides,50 yielding a final hybrid radioactive bioconjugate GlcNAc-D-Na125I@SWCNTs, bearing N-acetylglucosamine (GlcNAc) moieties exohedrally (Fig. 2a). Detection of the γ-radiation stemming from the encapsulated Na125I through single-photon emission computed tomography (SPECT), allowed the determination of the bio-distribution of the GlcNAc-D-Na125I@SWCNTs hybrids at very low doses of radioactivity (0.2–1.0 MBq) compared to that used in imaging studies of classical CNTs by single photon emission CT (SPECT/CT) technologies. The striking localization and persistence of GlcNAc-D-Na125I@SWCNTs in the lungs (several days post-injection, Fig. 2b) along with the apparent good tolerance is now under investigation for longer-term delivery of radionuclides, with possible applications in lung cancer diagnosis and treatment. Reports describing the use of US-tubes to host other biomedically-relevant elements such as bismuth (as BiOCl/Bi2O3) for X-ray-based imaging51 or astatine (211AtCl) for α-particle radiotherapy,52 have been also reported with promising outcomes.
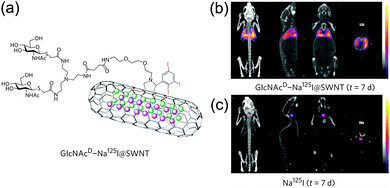 |
| Fig. 2
GlcNAc-D-Na125I@SWCNTs structural features (a) and whole-animal SPECT/CT imaging using the nanohybrid (b) or reference Na125I (c) with a scanning time of 40–60 min 7 days post injection (adapted with permission from ref. 48, Copyright 2010, Nature Publishing Group). | |
The luminescent properties of organic molecular emitters, such as intensity and color, are strongly dependent on the local chemical environment, aggregation phenomena or parasitic interactions. A valuable approach to control molecular emission would consist of the isolation of the luminophores within a cavity. In this respect, following the nano-extraction methodology,13 we have recently reported on the endohedral functionalization of multi-walled CNTs (MWCNTs) with a neutral and hydrophobic tris-hexafluoro acetylacetonate EuIII complex (Fig. 3).53
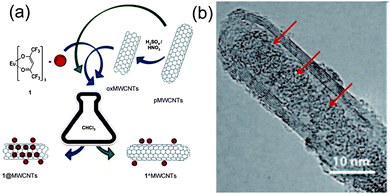 |
| Fig. 3 Preparative protocols for EuIII complex insertion in MWCNTs (a) and HRTEM images of EuIII@MWCNTs showing the heavy atoms as black spots ((b), adapted with permission from ref. 53, Copyright 2011, Wiley-VCH). | |
EuIII complexes are among the most versatile luminophores available as they show intense and chromatically pure emissions thanks to the peculiar nature of the “inner” electronic f levels.54 To afford EuIII encapsulation, MWCNTs were oxidized following a two-step procedure, where treatment with a concentrated sulphonitric mixture led to the opening of the tips and carboxylation reaction, while the second step (heating at 450 °C in air for 1 h) allowed decarboxylation. Despite the confinement within the graphitic cavity (through the nano-extraction), the emission properties of the lanthanide complex were maintained in the nanohybrid.
4. Filling CNTs for drug-delivery
Hilder and Hill calculated the optimal SWCNTs diameter for encapsulation of small-molecule anticancer drugs, such as cisplatin (1 nm), paclitaxel (between 1.83 and 2.54 nm), or doxorubicin (between 1.77 and 2.10 nm).55,56 Among the different anticancer drugs, cisplatin is certainly the most studied bioactive molecule for endohedral CNTs functionalization, because it can be easily localized in the tubular framework by microscopic (TEM) and spectroscopic (XPS, EDS) characterization techniques. Büchner and co-workers reported the first MWNTs encapsulating a cisplatin analogue (carboplatin, CP) using a wet chemical approach (CP@MWCNTs).57 Interestingly, interfacing CP@MWCNTs nanostructures with bladder cancer cells enhanced the cytotoxicity activity compared to empty MWCNTs. Other examples describing endohedral SWCNTs58 or MWCNTs59 containing cisplatin or its derivatives have been also reported.60
In a parallel work, Ren and Pastorin described the use of SWCNTs and double-walled CNTs (DWCNTs) as capped “carbon nano-bottles” for storing drugs prepared by sealing the open ends of filled CNTs (Fig. 4).61
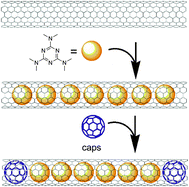 |
| Fig. 4 Schematic representation for preparing “carbon nano-bottles” loaded with the antitumor agent HMM and C60 using a two-step nano-extraction strategy (adapted with permission from ref. 61, Copyright 2008, Wiley-VCH). | |
In particular, the antitumor drug hexamethylmelamine (HMM) was employed as a model drug while C60 was the removable stopper. Using a two-step nano-extraction method, HMM was loaded first immersing the nanotubes in a saturated aqueous solution of HMM and then in an EtOH solution saturated with C60. Notably, removal of the fullerene stopper could be achieved by dispersing the sealed “nano-bottles” in CH2Cl2.
The same group has then switched to MWCNTs as drug delivery scaffolds, to reduce the possibility of leakage of the hosted phase from the sidewalls,62 by nano-extracting cisplatin and using caps made of functional gold nanoparticles (GNPs). GNPs caps decreased the rate of cisplatin release, especially in the first 1 h, thus suggesting an active role for the GNP caps in controlling the drug release. Later, Honek and co-workers reported NIR-light externally-inducible drug-delivery from filled CNTs.63 In that study CNTs have been filled with indole and exohedrally equipped with a peptide targeting cancer cells that overexpress the receptor EphB4 and the dye Lucifer yellow (LY). Cell–nanomaterial interactions were assessed by confocal microscopy by labelling EphB4 with the green fluorescent protein (GFP). Then, by incubating cells with the nanomaterial for 30 min and irradiating with a 10 s pulsed NIR laser for 10 or 15 min, the EphB4-expressing cells underwent apoptosis after 12 hours, while the reference cells (EphB4 negative) were not affected. It is worth also mentioning that inductive heating with an external alternating current or pulsed magnetic field triggered drug discharge from filled CNTs, allowing for the lowering of the effective concentration of the anticancer drug taxol toward selected cancer cells by about 100 times.64
5. Filling CNTs for magnetically-driven biomedical applications
Among the different encapsulable bioactive nanomaterials, magnetic nanoparticles (MNPs) hold great potential for magnetic drug-targeting and/or delivery (MDT and MDD respectively) MRI, and localized magnetic fluid hyperthermia (MFH).65 Filling CNTs with magnetically-active nanostructures can be easily achieved following in situ procedures, exploiting CVD protocols. If high quantities of metal catalyst precursors such as ferrocene (Fc), cobaltocene or Fe2CO10 are used, metal nanowires of α-Fe, iron carbides, or alloys, like FeCO, can be easily confined in CNTs during their synthesis.25 Ren and co-workers have shown that weakly-magnetic nanotubes, bearing minor quantities of confined catalyst residues, could be used for shuttling genetic material inside cells through the so-called CNTs spearing process.66 Exohedral DNA–CNTs hybrids were magnetically driven through cell membranes, causing a high transduction efficiency in Bal17 B-lymphoma cells or primary neurons. MDT, shepherding of mesenchymal stem cells,67 and the measurements of specific protein binding68 could be also performed with weakly-magnetic CNTs. Biocompatibility studies of highly metal-filled CNTs (M@CNTs) in the presence of lipids69 or human serum albumin70 and high drug-loading capacity71 have been recently reported. Fe-filled MWCNTs (Fe@MWCNTs), noncovalently functionalized with pluronic F127, were developed for in vitro and in vivo MR-guided laser-induced thermotherapy, with promising outcomes.72 It was also demonstrated that injection of N-doped MWCNT into a murine renal tumor implanted on a mouse flank followed by 30 s of low-energy NIR exposure was effective at preventing tumor growth and inducing long-term (>180 days) remission in 80% of the treated mice.73 We have also recently reported on the preparation and biological evaluation of Fe@MWCNTs functionalized exohedrally with the epidermal growth factor receptor (EGFR)-targeting antibody Cetuximab (Ctx).74 Fe@MWCNTs containing magnetically-active iron phases (α-Fe and Fe3C, as revealed by EDS, XPS, X-ray diffraction and 57Fe-Mössbauer) were grown through CVD using Fc as the metal catalyst precursor and toluene as a complementary carbon feedstock. Organic derivatization of the external wall, following the procedure of Bahr and Tour,75 yielded Fe@MWCNTs decorated exohedrally with benzylic primary amino groups that could be derivatised straightforwardly with Ctx (Fe@MWCNTs-Ctx, Fig. 5a) under typical amidation reaction conditions. The binding activities of Fe@MWCNTs-Ctx towards EGFR+ cells were assessed by enzyme-linked immunosorbent assay (ELISA, Fig. 5b) and Western blotting, both showing that Ctx retained its fundamental structural and targeting properties even after the conjugation step, as also confirmed by computational studies.76 Magnetic filtration experiments showed that after 8 minutes of exposure to a permanent magnet, EGFR+ cells were coagulated whereas those that were EGFR− remained in suspension. Cell viability assessment showed that exposure of high doses (up to 200 μg mL−1) of Fe@MWCNT did not cause significant cytotoxicity regardless of the cell line. Subsequently, MFH under an alternating current magnetic field of 83 KA m−1 and f = 220 KHz for 15 minutes was exploited on isolated and mixed EGFR+ and EGFR− cells suspensions. When isolated, EGFR+ cells were revealed to be affected significantly in their viability (about 20–25% of cell death), whereas EGFR− negative cells remained essentially unaltered under the same conditions (about 7–8% of cell death). Upon application of the MFH, it was possible to assess by confocal microscopy an increased cytotoxicity for the EGFR+ cells. It was also revealed clearly that Fe@MWCNTs-Ctx surround selectively and agglomerate onto EGFR+ cells (Fig. 5c), therefore maximizing local Fe concentration, which is responsible for the MFH effect.
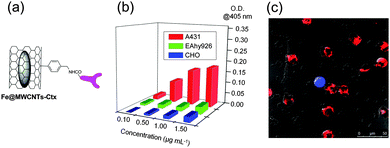 |
| Fig. 5 Structural representation of Fe@MWCNTs-Ctx (a), ELISA results (b) and confocal microscopy image of mixed cells in the presence of Fe@MWCNTs-Ctx (c). EGFR+ cells are red, while EGFR− cells are blue or green (adapted with permission from ref. 74, Copyright 2013, Wiley-VCH). | |
6. Toxicological properties
Since CNTs are nowadays produced at the multi-kg scale and have started to be implemented in commercially available products,77 their safety profile is under vigorous investigation. CNTs’ in vivo behavior seems to be closely associated with exohedral surface physicochemical properties, size distribution, doses and administration route.3,78 Most commonly, CNTs are expelled through both renal and fecal pathways,3 with recent findings also suggesting possible enzymatic degradation pathways.79 Filled CNTs are subjected to the same criteria of toxicological evaluation as their unfilled counterparts, the external sidewall properties determining the biocompatibility degree of such nanomaterials. As a general consideration, improved biocompatibility with proper exohedral functionalization and optimized size distribution will help to achieve minimal toxicity.
7. Conclusions
Filling strategies for preparing CNT hybrids for nanobiotechnological applications have emerged rapidly in recent years. These studies paved the way for the controlled delivery of bioactive molecules under external inputs. In this respect, filling of CNTs with magnetically-responsive nanoparticles is one of the most promising research approaches, thanks to the possibility of merging CNTs covalent and non-covalent chemistry with a multitude of biomedical activities mediated by electromagnetic stimulation.
Acknowledgements
D. B. gratefully acknowledges the EU through the ERC Starting Grant “COLORLANDS”, ITN “FINELUMEN” PITN-GA-2008-215399 projects, the FP7 NMP small collaborative project “SACS”, the FRS-FNRS (FRFC contracts no. 2.4.550.09 and MIS no. F.4.505.10.F), the “Loterie Nationale”, the Science Policy Office of the Belgian Federal Government (BELSPO-IAP 7/05 project), the MIUR through the FIRB “Futuro in Ricerca” (“SUPRACARBON”, contract no. RBFR10DAK6). R. M. thanks FRS-FNRS for his research associate position.
Notes and references
-
M. S. Dresselhaus, G. Dresselhaus and P. Avouris, Carbon Nanotubes: Synthesis, Structures, Properties and Applications, Springer-Verlag, Berlin, Heidelberg, 2001 Search PubMed.
- C. Cha, S. R. Shin, N. Annabi, M. R. Dokmeci and A. Khademhosseini, ACS Nano, 2013, 7, 2891–2897 CrossRef CAS PubMed.
- E. Heister, E. W. Brunner, G. R. Dieckmann, I. Jurewicz and A. B. Dalton, ACS Appl. Mater. Interfaces, 2013, 5, 1870–1891 CAS.
- C. Ménard-Moyon, E. Venturelli, C. Fabbro, C. Samorì, T. Da Ros, K. Kostarelos, M. Prato and A. Bianco, Expert Opin. Drug Discovery, 2010, 5, 691–707 CrossRef PubMed.
- Y. Zhang, Y. Bai and B. Yan, Drug Discovery Today, 2010, 15, 428–435 CrossRef CAS PubMed.
- Z. Liu, S. Tabakman, K. Welsher and H. Dai, Nano Res., 2010, 2, 85–120 CrossRef PubMed.
- N. W. S. Kam, M. O'Connell, J. A. Wisdom and H. Dai, Proc. Natl. Acad. Sci. U. S. A., 2005, 102, 11600–11605 CrossRef CAS PubMed.
- C. J. Gannon, P. Cherukuri, B. I. Yakobson, L. Cognet, J. S. Kanzius, C. Kittrell, R. B. Weisman, M. Pasquali, H. K. Schmidt, R. E. Smalley and S. A. Curley, Cancer, 2007, 110, 2654–2665 CrossRef CAS PubMed.
- C. Schmuck, Angew. Chem., Int. Ed., 2007, 46, 5830–5833 CrossRef CAS PubMed.
-
U. H. Brinker and J.-L. Mieusset, Molecular Encapsulation: Organic Reactions in Constrained Systems, John Wiley & Sons, Chichester, UK, 2010 Search PubMed.
- B. W. Smith, M. Monthioux and D. E. Luzzi, Nature, 1998, 396, 323–324 CrossRef CAS PubMed.
- A. N. Khlobystov, D. A. Britz and G. A. D. Briggs, Acc. Chem. Res., 2005, 38, 901–909 CrossRef CAS PubMed.
- M. Yudasaka, K. Ajima, K. Suenaga, T. Ichihashi, A. Hashimoto and S. Iijima, Chem. Phys. Lett., 2003, 380, 42–46 CrossRef CAS PubMed.
- F. Simon, H. Kuzmany, H. Rauf, T. Pichler, J. Bernardi, H. Peterlik, L. Korecz, F. Fülöp and A. Jánossy, Chem. Phys. Lett., 2004, 383, 362–367 CrossRef CAS PubMed.
- P. M. Ajayan and S. Iijima, Nature, 1993, 361, 333–334 CrossRef CAS.
- D. Eder, Chem. Rev., 2010, 110, 1348–1385 CrossRef CAS PubMed.
- A. Ilie, J. S. Bendall, K. Nagaoka, S. Egger, T. Nakayama and S. Crampin, ACS Nano, 2011, 5, 2559–2569 CrossRef CAS PubMed.
- K. Yanagi, Y. Miyata and H. Kataura, Adv. Mater., 2006, 18, 437–441 CrossRef CAS.
- K. Schulte, J. C. Swarbrick, N. A. Smith, F. Bondino, E. Magnano and A. N. Khlobystov, Adv. Mater., 2007, 19, 3312–3316 CrossRef CAS.
- T. Okazaki, Y. Iizumi, S. Okubo, H. Kataura, Z. Liu, K. Suenaga, Y. Tahara, M. Yudasaka, S. Okada and S. Iijima, Angew. Chem., Int. Ed., 2011, 50, 4853–4857 CrossRef CAS PubMed.
- L. J. Li, A. N. Khlobystov, J. G. Wiltshire, G. A. D. Briggs and R. J. Nicholas, Nat. Mater., 2005, 4, 481–485 CrossRef CAS PubMed.
- A. Chuvilin, E. Bichoutskaia, M. C. Gimenez-Lopez, T. W. Chamberlain, G. A. Rance, N. Kuganathan, J. Biskupek, U. Kaiser and A. N. Khlobystov, Nat. Mater., 2011, 10, 687–692 CrossRef CAS PubMed.
-
F. Simon and M. Monthioux, Carbon Meta-Nanotubes, John Wiley & Sons, 2011, pp. 273–321 Search PubMed.
- A. N. Khlobystov, ACS Nano, 2011, 5, 9306–9312 CrossRef CAS PubMed.
- U. Weissker, S. Hampel, A. Leonhardt and B. Büchner, Materials, 2010, 3, 4387–4427 CrossRef CAS.
- D. Mattia and Y. Gogotsi, Microfluid. Nanofluid., 2008, 5, 289–305 CrossRef CAS.
- T. W. Ebbesen, J. Phys. Chem. Solids, 1996, 57, 951–955 CrossRef CAS.
- A. V. Talyzin, I. V. Anoshkin, A. V. Krasheninnikov, R. M. Nieminen, A. G. Nasibulin, H. Jiang and E. I. Kauppinen, Nano Lett., 2011, 11, 4352–4356 CrossRef CAS PubMed.
- B. L. Cushing, V. L. Kolesnichenko and C. J. O'Connor, Chem. Rev., 2004, 104, 3893–3946 CrossRef CAS PubMed.
- W. Baaziz, S. Begin-Colin, B. P. Pichon, I. Florea, O. Ersen, S. Zafeiratos, R. Barbosa, D. Begin and C. Pham-Huu, Chem. Mater., 2012, 24, 1549–1551 CrossRef CAS.
- S. Boncel, A. P. Herman and K. Z. Walczak, J. Mater. Chem., 2012, 22, 31–37 RSC.
- M. Del Carmen Giménez-López, F. Moro, A. La Torre, C. J. Gómez-García, P. D. Brown, J. Van Slageren and A. N. Khlobystov, Nat. Commun., 2011, 2, 407 CrossRef PubMed.
- J. J. Davis, M. L. H. Green, H. A. O. Hill, Y. C. Leung, P. J. Sadler, J. Sloan, A. V. Xavier and S. C. Tsang, Inorg. Chim. Acta, 1998, 272, 261–266 CrossRef CAS.
-
A. E. Merbach and E. Toth, The Chemistry of Contrast Agents in Medical Magnetic Resonance Imaging, Wiley, Chichester, 2001 Search PubMed.
- P. Caravan, J. J. Ellison, T. J. McMurry and R. B. Lauffer, Chem. Rev., 1999, 99, 2293–2352 CrossRef CAS PubMed.
- R. D. Bolskar, A. F. Benedetto, L. O. Husebo, R. E. Price, E. F. Jackson, S. Wallace, L. J. Wilson and J. M. Alford, J. Am. Chem. Soc., 2003, 125, 5471–5478 CrossRef CAS PubMed.
- Z. Gu, H. Peng, R. H. Hauge, R. E. Smalley and J. L. Margrave, Nano Lett., 2002, 2, 1009–1013 CrossRef CAS.
- B. Sitharaman, K. R. Kissell, K. B. Hartman, L. A. Tran, A. Baikalov, I. Rusakova, Y. Sun, H. A. Khant, S. J. Ludtke, W. Chiu, S. Laus, É. Tóth, L. Helm, A. E. Merbach and L. J. Wilson, Chem. Commun., 2005, 3915–3917 RSC.
- R. Sethi, Y. MacKeyev and L. J. Wilson, Inorg. Chim. Acta, 2012, 393, 165–172 CrossRef CAS PubMed.
- Q. Ma, M. Jebb, M. F. Tweedle and L. J. Wilson, J. Mater. Chem. B, 2013, 1, 5791–5797 RSC.
- L. A. Tran, R. Krishnamurthy, R. Muthupillai, M. da Graça Cabreira-Hansen, J. T. Willerson, E. C. Perin and L. J. Wilson, Biomaterials, 2010, 31, 9482–9491 CrossRef CAS PubMed.
- K. B. Hartman, S. Laus, R. D. Bolskar, R. Muthupillai, L. Helm, E. Toth, A. E. Merbach and L. J. Wilson, Nano Lett., 2008, 8, 415–419 CrossRef CAS PubMed.
- E. J. Rivera, R. Sethi, F. Qu, R. Krishnamurthy, R. Muthupillai, M. Alford, M. A. Swanson, S. S. Eaton, G. R. Eaton and L. J. Wilson, Adv. Funct. Mater., 2012, 22, 3691–3698 CrossRef CAS.
- G. Dai, O. Levy and N. Carrasco, Nature, 1996, 379, 458–460 CrossRef CAS PubMed.
- Y. A. Mackeyev, J. W. Marks, M. G. Rosenblum and L. J. Wilson, J. Phys. Chem. B, 2005, 109, 5482–5484 CrossRef CAS PubMed.
- J. M. Ashcroft, K. B. Hartman, K. R. Kissell, Y. Mackeyev, S. Pheasant, S. Young, P. A. W. Van Der Heide, A. G. Mikos and L. J. Wilson, Adv. Mater., 2007, 19, 573–576 CrossRef CAS.
- L. Shao, G. Tobias, Y. Huh and M. L. Green, Carbon, 2006, 44, 2855–2858 CrossRef CAS PubMed.
- S. Y. Hong, G. Tobias, K. T. Al-Jamal, B. Ballesteros, H. Ali-Boucetta, S. Lozano-Perez, P. D. Nellist, R. B. Sim, C. Finucane, S. J. Mather, M. L. H. Green, K. Kostarelos and B. G. Davis, Nat. Mater., 2010, 9, 485–490 CrossRef CAS PubMed.
- B. Ballesteros, G. Tobias, L. Shao, E. Pellicer, J. Nogués, E. Mendoza and M. L. H. Green, Small, 2008, 4, 1501–1506 CrossRef CAS PubMed.
- V. Georgakilas, K. Kordatos, M. Prato, D. M. Guldi, M. Holzinger and A. Hirsch, J. Am. Chem. Soc., 2002, 124, 760–761 CrossRef CAS PubMed.
- E. J. Rivera, L. A. Tran, M. Hernández-Rivera, D. Yoon, A. G. Mikos, I. A. Rusakova, B. Y. Cheong, M. D. G. Cabreira-Hansen, J. T. Willerson, E. C. Perin and L. J. Wilson, J. Mater. Chem. B, 2013, 1, 4792–4800 RSC.
- K. B. Hartman, D. K. Hamlin, D. S. Wilbur and L. J. Wilson, Small, 2007, 3, 1496–1499 CrossRef CAS PubMed.
- L. Maggini, J. Mohanraj, H. Traboulsi, A. Parisini, G. Accorsi, N. Armaroli and D. Bonifazi, Chem.–Eur. J., 2011, 17, 8533–8537 CrossRef CAS PubMed.
- J. C. G. Bünzli, Acc. Chem. Res., 2006, 39, 53–61 CrossRef PubMed.
- T. A. Hilder and J. M. Hill, Curr. Appl. Phys., 2008, 8, 258–261 CrossRef PubMed.
- T. A. Hilder and J. M. Hill, Micro Nano Lett., 2008, 3, 41–49 CAS.
- S. Hampel, D. Kunze, D. Haase, K. Krämer, M. Rauschenbach, M. Ritschel, A. Leonhardt, J. Thomas, S. Oswald, V. Hoffmann and B. Büchner, Nanomedicine, 2008, 3, 175–182 CrossRef CAS PubMed.
- A. Guven, I. A. Rusakova, M. T. Lewis and L. J. Wilson, Biomaterials, 2012, 33, 1455–1461 CrossRef CAS PubMed.
- C. Tripisciano, S. Costa, R. J. Kalenczuk and E. Borowiak-Palen, Eur. Phys. J. B, 2010, 75, 141–146 CrossRef CAS.
- J. Li, S. Q. Yap, C. F. Chin, Q. Tian, S. L. Yoong, G. Pastorin and W. H. Ang, Chem. Sci., 2012, 3, 2083–2087 RSC.
- Y. Ren and G. Pastorin, Adv. Mater., 2008, 20, 2031–2036 CrossRef CAS.
- J. Li, S. Q. Yap, S. L. Yoong, T. R. Nayak, G. W. Chandra, W. H. Ang, T. Panczyk, S. Ramaprabhu, S. K. Vashist, F. S. Sheu, A. Tan and G. Pastorin, Carbon, 2012, 50, 1625–1634 CrossRef CAS PubMed.
- Z. Su, S. Zhu, A. D. Donkor, C. Tzoganakis and J. F. Honek, J. Am. Chem. Soc., 2011, 133, 6874–6877 CrossRef CAS PubMed.
- C. H. Wu, C. Cao, J. H. Kim, C. H. Hsu, H. J. Wanebo, W. D. Bowen, J. Xu and J. Marshall, Nano Lett., 2012, 12, 5475–5480 CrossRef CAS PubMed.
- M. Colombo, S. Carregal-Romero, M. F. Casula, L. Gutiérrez, M. P. Morales, I. B. Böhm, J. T. Heverhagen, D. Prosperi and W. J. Parak, Chem. Soc. Rev., 2012, 41, 4306–4334 RSC.
- D. Cai, J. M. Mataraza, Z. H. Qin, Z. P. Huang, J. Y. Huang, T. C. Chiles, D. Carnahan, K. Kempa and Z. F. Ren, Nat. Methods, 2005, 2, 449–454 CrossRef CAS PubMed.
- O. Vittorio, P. Quaranta, V. Raffa, N. Funel, D. Campani, S. Pelliccioni, B. Longoni, F. Mosca, A. Pietrabissa and A. Cuschieri, Nanomedicine, 2011, 6, 43–54 CrossRef CAS PubMed.
- X. Diao, H. Chen, G. Zhang, F. Zhang and X. Fan, J. Nanomater., 2012, 806019 Search PubMed.
- I. Monch, A. Meye, A. Leonhardt, K. Kramer, R. Kozhuharova, T. Gemming, M. P. Wirth and B. Buchner, J. Magn. Magn. Mater., 2005, 290–291, 276–278 CrossRef PubMed.
- A. Taylor, K. Lipert, K. Kramer, S. Hampel, S. Fussel, A. Meyel, R. Klingeler, M. Ritschel, A. Leonhardt, B. Buchner and M. P. Wirth, J. Nanosci. Nanotechnol., 2009, 9, 5709–5716 CrossRef CAS PubMed.
- E. C. Vermisoglou, G. Pilatos, G. E. Romanos, E. Devlin, N. K. Kanellopoulos and G. N. Karanikolos, Nanotechnology, 2011, 22, 355602 CrossRef PubMed.
- X. Ding, R. Singh, A. Burke, H. Hatcher, J. Olson, R. A. Kraft, M. Schmid, D. Carroll, J. D. Bourland, S. Akman, F. M. Torti and S. V. Torti, Nanomedicine, 2011, 6, 1341–1352 CrossRef CAS PubMed.
- A. Burke, X. Ding, R. Singh, R. A. Kraft, N. Levi-Polyachenko, M. N. Rylander, C. Szot, C. Buchanan, J. Whitney, J. Fisher, H. C. Hatcher, R. D'Agostino Jr, N. D. Kock, P. M. Ajayan, D. L. Carroll, S. Akman, F. M. Torti and S. V. Torti, Proc. Natl. Acad. Sci. U. S. A., 2009, 106, 12897–12902 CrossRef CAS PubMed.
- R. Marega, F. De Leo, F. Pineux, J. Sgrignani, A. Magistrato, A. D. Naik, Y. Garcia, L. Flamant, C. Michiels and D. Bonifazi, Adv. Funct. Mater., 2013, 23, 3173–3184 CrossRef CAS.
- J. L. Bahr and J. M. Tour, Chem. Mater., 2001, 13, 3823–3824 CrossRef CAS.
- F. De Leo, J. Sgrignani, D. Bonifazi and A. Magistrato, Chem.–Eur. J., 2013, 19, 12281–12293 CrossRef CAS PubMed.
- M. F. L. De Volder, S. H. Tawfick, R. H. Baughman and A. J. Hart, Science, 2013, 339, 535–539 CrossRef CAS PubMed.
- J. Kolosnjaj-Tabi, K. B. Hartman, S. Boudjemaa, J. S. Ananta, G. Morgant, H. Szwarc, L. J. Wilson and F. Moussa, ACS Nano, 2010, 4, 1481–1492 CrossRef CAS PubMed.
- G. P. Kotchey, S. A. Hasan, A. A. Kapralov, S. H. Ha, K. Kim, A. A. Shvedova, V. E. Kagan and A. Star, Acc. Chem. Res., 2012, 45, 1770–1781 CrossRef CAS PubMed.
|
This journal is © The Royal Society of Chemistry and the Centre National de la Recherche Scientifique 2014 |
Click here to see how this site uses Cookies. View our privacy policy here.