DOI:
10.1039/C3NJ00603D
(Paper)
New J. Chem., 2014,
38, 63-69
Formation of gold nanoparticles upon chitosan leading to formation and collapse of gels†
Received
(in Montpellier, France)
6th June 2013
, Accepted 27th September 2013
First published on 30th September 2013
Abstract
In this article we have demonstrated the formation of chitosan–gold hydro gels that collapse in time. The mechanism for the formation and collapse in time is proposed. The gels were characterized using scanning electron microscopy (SEM), attenuated total reflectance Fourier transform infrared spectroscopy (ATR-FTIR), rheometry and X-ray diffraction (XRD). The formation of the gels is hypothesised to be due to the electrostatic interaction between the AuCl4− and the NH3+ in the protonated chitosan. The collapse of the gel is attributed to the reduction of Au(III) to Au nanoparticles on the chitosan surface. The formation and the subsequent collapse of the gel were unique to gold under the experimental conditions. A model is proposed for the formation and the collapse of the gel. We coin the term “self collapsing gels” for these kinds of gels. The gels were tested for their abilities to be used for the encapsulation of drugs using insulin. This self collapsing nature of the gels combined with biocompatibility may benefit drug delivery research as encapsulants for the controlled release of drugs.
1. Introduction
Biopolymers are an important class of polymeric materials that have attracted the attention of the scientific community greatly. Chitosan is a biopolymer and is derived from chitin, which is the second most abundantly available biopolymer in nature.1 Chitosan and its derivatives have found extensive biomedical applications since they are biocompatible and biodegradable.2,3 Chitosan is also shown to have an antibacterial property.4 Some of its applications include heavy metal chelation, wound healing, cell immobilization in wastewater treatment and as an additive in food and cosmetics.5,6 The properties of chitosan solutions depend upon various parameters that include concentration, temperature, pH, ionic strength, type of acid and acetyl groups present.7
In addition to these properties, chitosan is reported to have a good ability to bind with other polymers and inorganic materials and thus form composite materials.8 Typically, such hybrid materials show the properties of the individual building blocks along with synergetic effects, due to the interaction between the individual components.9 The possible multifunctional properties of these kinds of composites attracted wide attention recently. Gold nanoparticles (Au NPs) are extensively used to create composites with chitosan and its derivatives. Many applications of gel–chitosan composites have been reported. These include surface-enhanced Raman scattering,10 electrochemical sensors,11 molecular imaging12 and catalysis.13 A green synthetic approach for making gold nanoparticles is new and useful for the environment.14 Recently, Hortigüela et al.15 reported an interesting synthetic approach that induced chitosan gelation upon the simultaneous formation of AuNPs. They reported that prolonged thermal treatment at 80 °C promoted the hydrogels to become a viscous liquid.
In this article we report a single-step approach for the formation of a chitosan–gold hydrogel and the collapse of the gel in time with the formation of chitosan–gold nanoparticle composites at room temperature (30 ± 2 °C). The studies were done as a function of the AuCl4− concentration, pH, temperature and reaction time. Importantly, we report for the first time the formation and the collapse of a gold–chitosan hybrid gel with time and therefore we coin the term “self collapsing gels” for these classes of gels that collapse due to the chemical content. One of the possible applications of the gel that will collapse at specific times may include the encapsulation of a drug and its controlled delivery. The as-synthesized gels are also found to be sensitive to external stimuli like pH, gold concentration and heat. Such responsive hybrid-microgels may be interesting for a variety of applications including catalysis, optical, sensors, smart actuators and transport media.
2. Experimental section
2.1. Reagents
Chitosan powder and acetic acid (99.9% purity) were purchased from SRL Pvt. Ltd, Mumbai, India. Chloroauric acid powder (HAuCl4·3H2O) with 49% Au was purchased from the CDH Company Pvt. Ltd, India. Double distilled water was used throughout the synthesis process. Human insulin (biphase isophane insulin injection I.P. 40 IU ml−1) was purchased from Torrent Pharmaceutical Ltd, India.
2.2. Preparation of chitosan gold gels
In this work, 1% (w/v) of chitosan powder (i.e.) 2 g/200 ml of chitosan and 1.5% (w/v) of acetic acid (i.e.) 3 g/200 ml were dissolved in 200 ml of double distilled water. The solution was stirred using a magnetic stirrer and heated at 60 °C for 60 min until the chitosan powder was completely dissolved and a semi-transparent thick chitosan solution was obtained. To this solution, appropriate amounts of 1 M HAuCl4·3H2O were added to have 1–5 mM HAuCl4.
2.3. Characterization
UV-visible absorption spectra were recorded using a Perkin-Elmer Lambda 25 spectrophotometer in the range 200–1100 nm. A FEI Quanta FEG 200 was used for taking the high resolution scanning electron microscope (HRSEM) images. The samples were spot-cast upon ITO substrates and dried under ambient conditions. The instrument RheoPlus (Anton Paar) was used to measure the viscosity of the samples. The viscosity was measured at 25 °C and at a shear rate of 10 s−1. The X-ray diffraction (XRD) patterns of the sample were collected using a Bruker AXS, D8 Discover diffractometer. The wavelength used was 1.54184 Å. The samples were scanned in the 2θ range from 10 to 90°. All the peaks were assigned and compared with the database published by the Joint Committee on Powder Diffraction Standards (JCPDS).
3. Results and discussions
3.1. Formation of gels
Fig. 1A shows the effect of concentrations of HAuCl4 (1–5 mM) on the formation of the gold–chitosan hydrogel at room temperature. A direct correlation was observed between the initial concentration of the HAuCl4 and the rate of gel formation. The addition of the gold precursor also resulted in color changes of the solution with time. For 1 mM HAuCl4, it started as yellow, then became wine red in 24 h and finally became dark brown. Samples containing 2 mM HAuCl4 also showed a similar trend as that of 1 mM HAuCl4. This indicates the formation of gold nanoparticles. Very recently, Boufi et al.,17 have proved the ability of chitosan to reduce Au(III) to Au NPs in absence of any external chemical reducing agents. They observed a color change of a AuCl4− chitosan solution from yellowish to red when exposed to UV irradiation and attributed the color change to the formation of Au NPs. Huang and Yang16 also reported a similar color change from pale yellow-to red after heating a AuCl4− chitosan solution at 80 °C for 2 h and UV-irradiation for 15 min. Our study showed the color change and formation of Au NPs at room temperature (30 ± 2 °C) in the absence of any external physical and chemical agents. Interestingly, upon increasing the concentration of HAuCl4 to 3 mM and above, the formation of a gel was observed and the rate of formation was found to be concentration dependent. It was also observed that as time progressed, the gels broke and became a viscous liquid again. The gels with 3, 4 and 5 mM HAuCl4 concentrations collapsed after 2, 3 and 4 days, respectively. Unlike previous reports, the observed gel formation and the subsequent collapse of the gel due to the formation of Au NPs might be due to the difference in the concentration of the reactants employed.
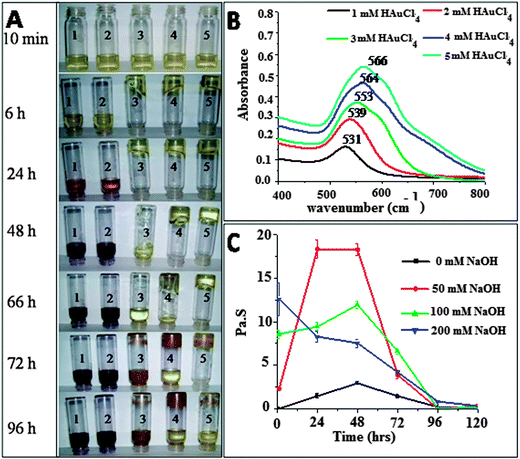 |
| Fig. 1 (A) Photographic images showing the time dependent response of chitosan–HAuCl4 complex. (1) 1 mM HAuCl4 (2) 2 mM HAuCl4 (3) 3 mM HAuCl4 (4) 4 mM HAuCl4 and (5) 5 mM HAuCl4. (B) UV-vis spectra of chitosan–gold (C) change in viscosity of the gels (1 wt% chitosan + 5 mM HAuCl4) with time as a function of NaOH concentration. | |
3.1. UV-vis measurements
The composite was characterized using a UV-vis spectrophotometer. Fig. 1B shows the UV-vis spectra of the chitosan solutions with various concentrations of HAuCl4, measured after the collapse of the gel. A broad absorption peak was observed at around 531 nm for the chitosan solution with 1 mM HAuCl4. This peak corresponds to the excitation of the plasmon resonance band for gold NPs. It is also evident from the figure that as the concentration of the HAuCl4 increased, both the position of the peak (red-shift) and its absorbance increased linearly (Fig. S1 of ESI†). The progressive red-shift observed in the position of the absorbance can be attributed to the increase in the size of the gold nanoparticles. This observation is in agreement with the color change observed with the increase in the gold precursor concentration. The data reveal the formation of the gold nanoparticles on the chitosan template and the size of the gold particles formed are a function of the spiked concentration of HAuCl4. The results also confirm the inherent reducing ability of chitosan to reduce Au(III) to Au NPs in the absence of any external physical or chemical reducing agents.
3.2. Effect of pH and heat
Experiments were also conducted to know the effect of external stimuli like pH and temperature on the gel formation. The pH of the solution was varied using a 0.1 M NaOH solution. A predetermined volume of 1 M HAuCl4 was added to 5 mL of 1 wt% chitosan, to have a final concentration of 5 mM HAuCl4 and stirred for 2 minutes. To these, appropriate amounts of the NaOH were added to have concentrations of 50, 100 and 200 mM and stirred for 2 minutes. The pH was in the acidic range for all the samples. The pH for the 1 wt% chitosan solution, chitosan + 5 mM HAuCl4 and chitosan + 5 mM HAuCl4 + 100 mM NaOH were 4, 3.3 and 4.7, respectively.
The gel responded well to pH variations and the gel became harder with an increase in the concentration of the NaOH. This phenomenon was further confirmed by measuring the viscosity of the gels containing 5 mM HAuCl4 as a function of the concentration of NaOH added. The samples were also analyzed for their viscosities as a function of time and the results are shown in Fig. 1C. From the figure, it was observed that for a solution with 50 mM NaOH, the viscosity increased to a maximum in two days and then decreased. For 100 to 200 mM NaOH, the viscosity increased rapidly and reached a maximum value in ∼1 h. The reduction in viscosity was observed with time. This indicates that the addition of NaOH accelerates the formation of the gel. To know the effect of heat, a chitosan–gold gel having 5 mM HAuCl4 was placed on a hot plate. It was observed that the color of the gel exposed to the hot plate gradually changed from yellow to red. In time the entire gel became red in color and it collapsed. These results are shown in Fig. S2 of the ESI.† This indicates that heat accelerates the formation of the gold nanoparticles and subsequently the gel collapses due to reduction of the gold.
3.3. SEM measurements
Fig. 2 shows the SEM images of the gel (after collapsing) made using 5 mM HAuCl4. The EDAX images show that Au, Cl, C, N and O are distributed uniformly in the gel. The presence of Sn in the EDAX image is due to the ITO substrate. Fig. 3A, B and C show the HRSEM images for the parent chitosan, a chitosan–gold gel formed using 5 mM HAuCl4 and a chitosan–gold gel formed using 5 mM HAuCl4 and 100 mM NaOH, respectively. The samples were obtained by drying the solutions after the gel had collapsed. It was observed that chitosan did not show any orderly features (Fig. 3A). The chitosan gel formed using 5 mM HAuCl4, had spherical gold nanoparticles of size 71 ± 37 nm (Fig. 3B) while the chitosan gel formed using 5 mM HAuCl4 and 100 mM NaOH had gold nanoparticles of size 50 ± 9 nm (Fig. 3C). In the gel prepared in the presence of 100 mM NaOH, most of the particles were spherical, while some where triangular and rod shaped. The study also reveals that the gel is sensitive to external stimuli and small variations in the synthesis protocol can lead to a hybrid gel with different morphologies. This may help in making gels with different properties and hence different applications. The EDAX spectrum for the gel formed using 5 mM HAuCl4 is shown in Fig. 3D. The corresponding spectra of the gel formed using 5 mM HAuCl4 and 100 mM NaOH are shown in Fig. S3 of the ESI.† To confirm if the particle size increased with the increase in the concentration of HAuCl4 (as indicated by UV-vis measurements), SEM images of chitosan with 1 mM HAuCl4 were also taken (Fig. S4 of ESI†). The size of the nanoparticles formed using 1 mM HAuCL4 was 7.3 ± 1.6 nm, while the chitosan gel formed using 5 mM HAuCl4, had spherical gold nanoparticles of size 71 ± 37 nm (Fig. 3B). These results are in agreement with the UV-vis measurements and confirm that the size of the nanoparticles increases as the concentration of HAuCl4 increases.
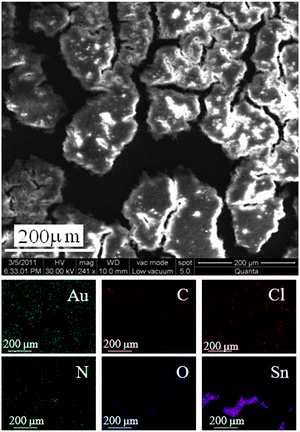 |
| Fig. 2 SEM image of a chitosan–gold complex (1 wt% chitosan + 5 mM HAuCl4). Corresponding EDAX mappings for various elements present in the complex are shown below. | |
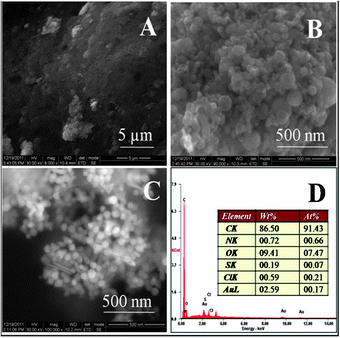 |
| Fig. 3 (A) HRSEM image of protonated chitosan. (B) HRSEM image of the composite containing 1 wt% chitosan and 5 mM HAuCl4. (C) HRSEM image of the composite containing 1 wt% chitosan, 5 mM HAuCl4 and 100 mM NaOH. (D) EDAX spectrum of the composite containing 1 wt% chitosan and 5 mM HAuCl4. | |
3.4. FTIR measurements
The samples were analyzed using ATR-FTIR (Fig. 4A). The spectra of the gel sample (chitosan–gold gel made using 5 mM HAuCl4) were obtained at different time intervals (2–28 h) from the time of formation of the gels. Peaks were observed at 3227, 2927, 2886, 1635, 1550, 1406, 1340, 1153, 1064, 1018 and 897 cm−1, respectively for both chitosan and the chitosan gels formed using 5 mM HAuCl4. Here, 3100–3500 cm−1 are due to OH and NH, 2927–2886 cm−1 are due to CH, 1635 cm−1 is due to the coupling of CO and NH, 1550 cm−1 is due to NH and 1406 cm−1 is due to the coupling of CN and NH.18 The position of the transmittance peaks for the protonated chitosan and the gel did not show any change between the time interval 2–28 h (Fig. 4A). Since the UV-vis measurements did not show any absorbance peak corresponding to that of the Au nanoparticles (531 nm) during this time interval (2–28 h), this indicates that the formation of the gel is due to electrostatic interactions between Au(III) and the chitosan. To further understand the effect of the formation of the nanoparticles upon the gels, FTIR spectra for the gel made using 5 mM HAuCl4 were taken at two different times (1) 6 h (the gel had freshly formed) and (2) 120 h (the gel had completely collapsed). The results are shown in Fig. S5 of the ESI.† For chitosan, the amide I, amide II and amide III bands are located at 1656, 1593 and 1373 cm−1, respectively.19 From Fig. S5, ESI,† it was observed that the absorbance for amide II and amide III were suppressed for the time equal to 120 h. Also, the position of the amide III peak was shifted from 1395 (for 6 h) to 1385 (for 120 h). This indicates that the formation of the gold nanoparticles affects the amide bands of the chitosan. This can lead to a weakening of the binding links and thereby result in the collapse of the gels.
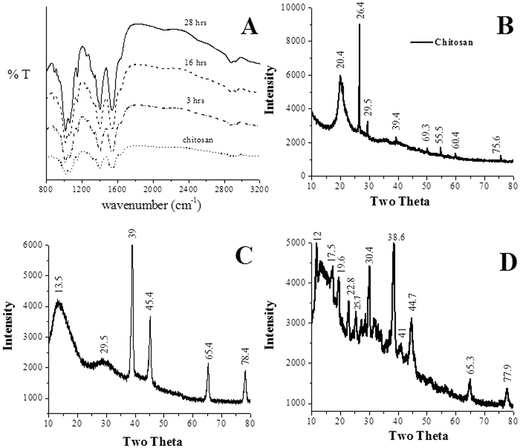 |
| Fig. 4 (A) ATR-IR spectra of the chitosan composite (1 wt% chitosan + 5 mM HAuCl4). Traces are shifted vertically for clarity. (B) XRD pattern of chitosan, (C) XRD pattern of the composite (1 wt% chitosan + 5 mM HAuCl4) and (D) XRD pattern of the composite containing 1 wt% chitosan, 5 mM HAuCl4 and 100 mM NaOH. | |
3.5. XRD analysis
The XRD patterns of the as-synthesized samples were also recorded and the data are shown in Fig. 4B, C and D. The samples for the analyses were obtained by drying the solutions after the gel had collapsed. The XRD of the gels formed using 5 mM HAuCl4 showed peaks for Au (2θ equal to 38.2°, 44.5°, 64.67° and 77.54°). The position and the intensity of the peaks corresponding to Au matched with the reported value (PCPDFWIN-01-1172). The gel formed using 5 mM HAuCl4 + 100 mM NaOH also showed peaks for Au (for 2θ equal to 38.2°, 44.5°, 64.67° and 77.54°) (PCPDFWIN-01-1172). The peaks corresponding to NaCl (27.4, 31.8, 53.8, 56.6, 66.2, 73.3 and 75.3) (PCPDFWIN-01-0993) and NaOH (11.7, 22.8, 28.7, 32.5 and 36.5) (PCPDFWIN-75-0642) were also observed (Fig. 4). The presence of planes corresponding to Au further confirms the inherent ability of chitosan to reduce Au(III) to Au(0).
3.6. Estimation of ratio of gold(III) and gold NPs
The role of the ratio Au(III)/AuNPs in the stability of the gel was found by the following technique. Chitosan–1 mM HAuCl4 and chitosan–3 mM HAuCl4 were prepared. The UV-vis absorbance was measured as a function of time for both the samples. The results are shown in Fig. 5. It was observed that the chitosan–1 mM HAuCl4 formed a liquid, while chitosan–3 mM HAuCl4 formed a gel. At 48 h, the chitosan–3 mM HAuCl4 had collapsed and become a liquid. Interestingly, the intensity of the absorbance maxima for the chitosan–3 mM HAuCl4 was comparable to that of the chitosan–1 mM HAuCl4. Also, the area under the peak (500–750 nm) for the chitosan–3 mM HAuCl4 was nearly the same as that of the area under the peak (450–700 nm) for the chitosan–1 mM HAuCl4. Hence, the amount of gold as nanoparticles was the same in the chitosan–1 mM HAuCl4 and in the chitosan–3 mM HAuCl4 collapsed gel. The absorbance spectra for the chitosan–1 mM HAuCl4 solution reached maximum in 2 days, indicating that all the gold was reduced as nanoparticles. This indicates that the amount of nanoparticles in the chitosan–3 mM HAuCl4 at the time of the collapse was 1 mm. Hence, the remaining 2 mM was Au(III). This indicates that the ratio of Au(III) and AuNPs is 2
:
1. When the ratio becomes less than 2
:
1, the gel collapses and becomes a liquid. These results show that out of 3 Au(III) ions, even if one of them is reduced to Au(0) then the gel will collapse.
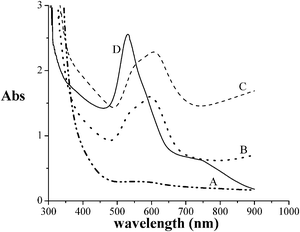 |
| Fig. 5 UV-vis spectra for (A) chitosan–3 mM HAuCl4 at 24 h (B) chitosan–3 mM HAuCl4 at 36 h (diluted by two) (C) chitosan–3 mM HAuCl4 at 48 h (diluted by two) and (D) chitosan–1 mM HAuCl4 at 48 h (diluted by two). The area under the peaks for (C) and (D) are the same. This indicates that the amount of Au as nanoparticles in (C) and (D) are the same. | |
3.7. Small angle neutron scattering studies
In a different study involving small angle neutron scattering (SANS), one of the authors (Ramasamy) has shown that the radius of gyration for chitosan was found to be 6.0 ± 0.5 nm, by fitting a model of a Gaussian polymer (communicated elsewhere). Assuming that in a gel, the radius of gyration is nearly the same as in solution (we can make this assumption as no change was observed in the volume of the chitosan–gold solution after it became a gel) and that the chitosan molecules do not cross each other, we can fill the gel space with boxes of dimensions 12 × 12 × 12 nm3, with each box having one chitosan unit. The number of chitosan molecules per cm3 is then ∼5.8 × 1017/cm−3. For the same volume the number of Au(III) in 3 mM HAuCl4 is ∼18.0 × 1017/cm−3. This shows that in a chitosan–3 mM HAuCl4 gel the ratio of chitosan to Au(III) is 1
:
3.1. This indicates that 3 Au(III) ions are associated with a chitosan molecule for it to be a gel. Both the analysis involving UV-vis and SANS show that for the gel to form, three Au(III) ions are required per chitosan molecule and that when one out of the three Au(III) ions gets reduced, then the gel will collapse. This is a collective phenomenon that involves many particles. Hence, the reduced Au(0) particles form gold NPs, with chitosan as their nucleating sites.
3.8. Dependence upon concentration of chitosan and other additives
It was also observed that the formation of the gel depended upon the concentration of the chitosan solution. To further prove this, to two different concentrations of a chitosan (0.25% and 1%) solution in 1.5% acetic acid, HAuCl4 was added to have final concentration of 5 mM. The results show that 0.25% of chitosan solution did not form gels (Fig. S6 of the ESI†) and a critical minimum concentration of chitosan was need for the formation of the gel. The studies further reveal the responsive nature of the gel to external conditions. The studies show that the formation of gel, the rate of gel formation, viscosity of the gel, the rate of formation of nanoparticles and collapse of the gel are largely dependent on the reaction environment, like the reactant concentration, pH and temperature. This responsive nature of the composite could be the reason for the absence of gel formation and the formation of gold NPs under ambient conditions, reported in previous studies by Boufi et al., 201317 and Huang and Yang, 2004.16
In order to test if other chemicals (instead of gold) could also yield chitosan gels, various chemicals were added in equal amounts (5 mM). The chemicals added include acetic acid, NaOH, NiCl2, ZnCl2, FeCl3, and AgNO3. The results reveal an interesting phenomenon which is specific to gold. The gel formation and subsequent collapse of the gel was found to be unique to HAuCl4 under the experimental conditions studied.
3.9. Application in drug delivery
To check if the chitosan–gold self collapsing gels can be used for drug delivery, the following studies were performed. Human insulin (biphase isophane insulin injection I.P. 40 IU ml−1) did not show any characteristic peaks, however the absorbance values decreased with increasing wavelength and the absorbance value at any particular wavelength was linearly proportional to the concentration. The absorbance at 400 nm was used to calibrate the dependence of the absorbance upon concentration of the insulin. 1 ml of human insulin was included in 5 ml of the chitosan–5 mM HAuCl4. Once a gel was formed, the gel was placed inside a beaker containing 100 ml of water. The absorbance of the supernatant was measured as a function of time (Fig. S7A of ESI†). The absorbance value for 400 nm was noted. The absorbance of the insulin source (1 ml human insulin dissolved in 100 ml water) is also shown in the figure for reference. From the absorbance profile in Fig. S7A, ESI,† it was observed that 25%, 30% and 35% of the insulin had been released from the gel into the solution for times equal to 24 h, 48 h and 72 h, respectively. At 96 h, the gel had completely collapsed and the absorbance value became more than that of the insulin source. It is therefore clear that the insulin was gradually and slowly released, until the gel completely collapsed.
In another experiment, 10 mg of methyl orange (MO) which is a common dye, was included in 5 ml of the chitosan–5 mM HAuCl4. Once a gel was formed, the gel was placed inside a beaker containing 100 ml of water. MO has an absorption maximum at 450 nm. Fig. S7A and S7B of the ESI† show the dependence of the absorbance of the supernatant upon time. The absorbance at 450 nm was noted. The absorbance of the methyl orange source (20 mg dissolved in 100 ml water) is also shown in the figure for reference. From Fig. S7B, ESI† it was observed that 17%, 20% and 26% of the MO had been released from the gel into the solution for times equal to 24 h, 48 h and 72 h, respectively. These experiments clearly indicate that chitosan–gold gels can be used to encapsulate drugs and release them in a controlled way.
3.10. Model for formation of the self collapsing gels
Fig. 6 shows the model for the formation of the self collapsing gels when 1, 3 and 5 mM HAuCl4 are added to a solution containing 1 wt% chitosan, with respect to time.
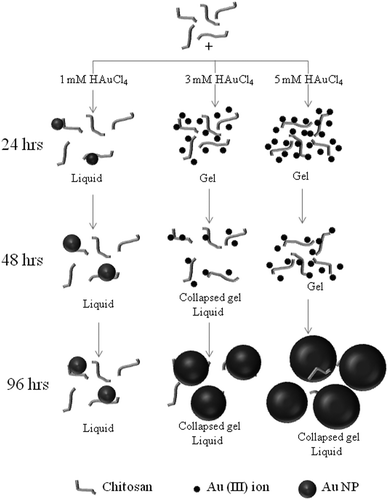 |
| Fig. 6 Model for the interaction between chitosan and HAuCl4. | |
24 h.
For times less than 24 h, chitosan solution containing 1 mM HAuCL4 forms gold nanoparticles, due to reduction of gold upon chitosan. The amount of gold is not sufficient to form gels as the ratio of the gold concentration to chitosan is less than 1
:
3.1 (from section 3.7. Small angle neutron scattering studies). For times less than 24 h, the chitosan solution containing 3 mM HAuCL4 and 5 mM HAuCL4 forms gels. This is due to the presence of Au(III) ions (dark small circles). Also, the ratio of gold concentration to chitosan is more than 1
:
3.1.
48 h.
In 48 h, the chitosan solution containing 1 mM HAuCL4 forms larger gold nanoparticles due to the further reduction of gold and it still remains as liquid. The chitosan solution containing 3 mM HAuCL4 collapses and becomes a liquid, since the ratio of Au(III) and Au (NPs) becomes less than 2
:
1 (from section 3.6. Estimation of ratio of gold(III) and gold NPs). The chitosan solution containing 5 mM HAuCL4 remains as a gel, as the ratio of Au(III) and Au (NPs) is greater than 2
:
1.
72 h.
In 72 h, the chitosan solution containing 1 mM HAuCL4 attains a saturation in the particle size. The chitosan solution containing 3 mM HAuCL4 has larger AuNPs, while the chitosan solution containing 5 mM HAuCL4 collapses as the ratio of Au(III) and Au (NPs) becomes less than 2
:
1 due to the reduction of the gold upon chitosan.
These indicate that, the electrostatic interaction between the (AuCl4)− and (NH3)+ on the protonated chitosan favors the formation of chitosan–gold–chitosan networks, leading to the formation of gels. In time, as more gold is reduced on the chitosan, the chitosan–gold–chitosan links breaks, leading to the collapse of the network.
4. Conclusions
Many studies have been reported explaining the gold–chitosan interaction. Several authors demonstrated the ability of chitosan to bind with gold ions and generate NPs. Huang et al.14 and Wang et al.20 reported that chitosan can act as both a metal stabilizing and a reducing agent. However, the reduction of gold was achieved by an external reducing agent, or heating, or by UV irradiation. It was hypothesized that the radical formed during the UV irradiation might act as the electron donor for the reduction. Another hypothesis says that the amino group on the chitosan acts as a nucleating site on which NPs grow using the hydroxyl groups as reducing agents. Our study supports the latter mechanism for NP formation, because Au(III) reduction was achieved without the influence of external chemical or physical agents.
This article describes the unique property of HAuCl4 to cross link chitosan and form a gel. The mechanism of formation of the hybrid gel and the self collapse with time is proposed. The formation of the gel is due to an electrostatic interaction between the gold precursor and the protonated chitosan. The collapse of the hybrid gel is attributed to the reduction of Au(III) and the subsequent formation of gold nanoparticles on the chitosan template. The formation and self collapse of the hybrid gel is found to be a function of external stimuli like pH, temperature and reactants concentrations. The ability of the chitosan–gel to release drugs has been demonstrated using insulin. These kinds of gels may therefore have potential applications in materials for controlled drug delivery, sensors, catalysis, etc.
Acknowledgements
The authors gratefully thank Prof. Abhijit P. Deshpande of the Polymer Engineering Laboratory, Chemical Engineering Department, Indian Institute of Technology Madras (IITM), India, for providing the facility for making the rheology measurements. The authors gratefully thank Prof. T. Pradeep of the DST unit of Nanoscience, Department of Chemistry and Sophisticated Analytical Instrument Facility, IITM, India, for providing the facility for making the UV-vis and SEM measurements. Prof. Ligy Philip, from the Department of Civil Engineering of IITM is acknowledged for providing the ATR-IR facility. This research was made possible due to funding provided by the BRNS (DAE) India Sanction No. 2011/37C/21/BRNS.
References
-
(a) I. Y. Kim, S. J. Seo, H. S. Moon, M. K. Yoo, I. Y. Park, B. C. Kim and C. S. Cho, Biotechnol. Adv., 2008, 26(1), 1–21 CrossRef CAS PubMed;
(b) J. Desbrieres, Biomacromolecules, 2002, 3(2), 342–349 CrossRef CAS PubMed;
(c) M. Rinaudo, Prog. Polym. Sci., 2006, 31(7), 603–632 CrossRef CAS PubMed.
-
(a) A. L. Andrady and P. Xu, J. Polym. Sci., Part B: Polym. Phys., 1997, 35, 517 CrossRef CAS;
(b) M. Morimoto, H. Saimoto, H. Usui, Y. Okamoto, S. Minami and Y. Shigemasa, Biomacromolecules, 2001, 2, 1133 CrossRef CAS PubMed;
(c) H. Sashiwa, N. Kawasaki, A. Nakayama, E. Muraki, N. Yamamoto and S. Aiba, Biomacromolecules, 2002, 3, 1126 CrossRef CAS.
-
(a) T. Freier, H. S. Koh, K. Kazazian and M. S. Shoichet, Biomaterials, 2005, 26, 5872–5878 CrossRef CAS PubMed;
(b) G. P. Dillon, X. J. Yu and R. V. Bellamkonda, J. Biomed. Mater. Res., 2000, 51, 510–519 CrossRef CAS;
(c) C. Chatelet, O. Damour and A. Domard, Biomaterials, 2001, 22, 261–268 CrossRef CAS.
- G. F. Payne, W. Q. Sun and A. Sohrabi, Biotechnol. Bioeng., 1992, 40, 1011–1018 CrossRef CAS PubMed.
-
(a) J. M. Randall, V. C. Randall, G. M. McDonald, R. N. Young and M. S. Masri, J. Appl. Polym. Sci., 1979, 23, 727–732 CrossRef CAS;
(b) R. A. A. Muzzarelli, Carbohydr. Polym., 1983, 3, 53–75 CrossRef CAS;
(c) C. A. McKnight, A. Ku and M. F. A. Goosen, J. Bioact. Compat. Polym., 1988, 3, 334–355 CrossRef CAS PubMed;
(d) E. Kokufuta, W. Mataumato and I. Nakamura, Biotechnol. Bioeng., 1982, 24, 1591 CrossRef CAS PubMed.
-
(a) Y. W. Cho, Y. N. Cho, S. H. Chung, G. Yoo and S. W. Ko, Biomaterials, 1999, 20, 2139–2145 CrossRef CAS;
(b) O. Pillai and R. Panchagnula, Curr. Opin. Chem. Biol., 2001, 5, 447–451 CrossRef CAS;
(c) E. Khor and L. Y. Lim, Biomaterials, 2003, 24, 2339–2349 CrossRef CAS;
(d) S. Yuan and T. Wei, J. Bioact. Compat. Polym., 2004, 19, 467–479 CrossRef PubMed.
-
(a) T. Matsumoto, M. Kawai and T. Masuda, Biopolymers, 1991, 31, 1721–1726 CrossRef CAS;
(b) R. H. Chen, J. H. Lin and M. H. Yang, Carbohydr. Polym., 1994, 24, 41–46 CrossRef CAS;
(c) H.-F. Guo, H.-L. Lin and T. L. Yu, J. Macromol. Sci., Part A: Pure Appl. Chem., 2002, A 39, 837–852 CrossRef PubMed;
(d) R. H. Chen and M. L. Tsaih, Int. J. Biol. Macromol., 1998, 23, 135–141 CrossRef CAS.
-
(a) A. Denuziere, D. Ferrier, O. Damour and A. Domard, Biomaterials, 1998, 19, 1275–1285 CrossRef CAS;
(b) J. Gong, L. Wang, K. Zhao and D. Song, Electrochem. Commun., 2008, 10, 123–126 CrossRef CAS PubMed.
- M. Karg, Colloid Polym. Sci., 2012, 290, 673–688 CAS.
- D. S. dos Santos Jr., P. J. G. Goulet, N. P. W. Pieczonka, O. N. Oliveira Jr. and R. F. Aroca, Langmuir, 2004, 20, 10273–10277 CrossRef PubMed.
-
(a) J. Lin, C. He, L. Zhang and S. Zhang, Anal. Biochem., 2009, 384(1), 130–135 CrossRef CAS PubMed;
(b) D. Feng, F. Wang and Z. Chen, Sens. Actuators, B, 2009, 138, 539–544 CrossRef CAS PubMed;
(c) M. Mathew, S. Sureshkumar and N. Sandhyarani, Colloids Surf., B, 2012, 93, 143–147 CrossRef CAS PubMed.
-
(a) S. Ahn, S. Yong Jung, B. H. Kim and S. J. Lee, Acta Biomater., 2011, 7, 2139–2147 CrossRef CAS PubMed;
(b) P. Agrawal, G. J. Strijkers and K. Nicolay, Adv. Drug Delivery Rev., 2010, 62, 42–58 CrossRef CAS PubMed.
- E. Guibal, Prog. Polym. Sci., 2005, 30, 71–109 CrossRef CAS PubMed.
- H. Huang and X. Yang, Carbohydr. Res., 2004, 339, 2627–2631 CrossRef CAS PubMed.
- M. J. Hortigüela, I. Aranaz, M. C. Gutiérrez, M. Luisa Ferrer and F. del Monte, Biomacromolecules, 2011, 12, 179–186 CrossRef PubMed.
- H. Z. Huang and X. R. Yang, Biomacromolecules, 2004, 5, 2340–2346 CrossRef CAS PubMed.
- S. Boufi, M. R. Vilar, A. M. Ferraria and A. M. B. do Regoc, Colloids Surf., A, 2013 DOI:10.1016/j.colsurfa.2012.12.036.
- L. Huang, M. Zhai, J. Peng, L. Xu, J. Li and G. Wei, J. Colloid Interface Sci., 2007, 316, 398–404 CrossRef CAS PubMed.
- K. C. Remant Bahadur, S. Aryal, S. R. Bhattarai, N. Bhattarai, C. H. Kim and H. Y. Kim, J. Biomater. Sci., Polym. Ed., 2006, 17, 579–589 CrossRef CAS PubMed.
- B. Wang, K. Chen, S. Jiang, F. Reincke, W. J. Tong, D. Y. Wang and C. Y. Gao, Biomacromolecules, 2006, 7, 1203–1209 CrossRef CAS PubMed.
Footnotes |
† Electronic supplementary information (ESI) available. See DOI: 10.1039/c3nj00603d |
‡ Both the authors have contributed equally. |
|
This journal is © The Royal Society of Chemistry and the Centre National de la Recherche Scientifique 2014 |
Click here to see how this site uses Cookies. View our privacy policy here.