Development and fundamental investigation of He plasma ionization detector (HPID) for gas chromatography using DC glow discharge
Received
26th February 2013
, Accepted 27th September 2013
First published on 24th October 2013
Abstract
In analytical chemistry, gas chromatography (GC) has been widely used because of the short measurement time and the low running costs. High-sensitivity detection is required for further improvement of the analytical performance. To date, several types of detectors have been developed. Thermal conductivity detectors (TCDs) are the most common GC detectors, and they are applicable for almost all samples. However, element-specific detection is impossible, and the limit of detection (LOD), which is on the order of 100 ppm, is not good. In this study, a new type of GC detector using atmospheric-pressure He plasma was developed. He has the highest ionization (24.58 eV) and metastable (19.82, 20.62 eV) energies among the elements. This means that He plasma can effectively ionize and excite all elements. In the helium plasma ionization detector (HPID), DC-powered He plasma and rod-like electrodes were utilized for ionization of the samples. For an ionization detector, the generation of very stable plasma is important. Therefore, we used DC He plasma. The sample was ionized when it was mixed into the He afterglow plasma. The concentration of each sample was then measured by the detection of the ion or electron current. When a 2 mL mixture of 20 ppm H2, N2, O2, CH4 and CO was introduced for GC, a LOD of 21–67 ppb was achieved. Thus, the detection ability of HPID was more than 1000 times better than that of TCD.
1. Introduction
Basic research on GC detectors using gas plasma began in the 1950s and 1960s. Modern gas chromatography began with the discovery by Pitkethly1 of a linear relationship between the glow discharge current and the concentration of butane introduced to the discharge. Lovelock is another well-recognized pioneer for research on GC detectors using gas discharge for sample ionization.2 These studies led to the development of the photoionization detector (PID), which utilizes ultraviolet rays emitted from the gas discharge for sample excitation and as an ionization source, and of the electron capture detector (ECD), which utilizes electrons generated from the discharge for the ionization of electrophilic compounds (subsequently, the ECD was replaced by a high-temperature filament or radiation source).3
Meanwhile, Braman and Dynako developed the atomic emission detector (AED), which measures spectra of ionized organic compounds in the DC He discharge.4 More recently, inductively coupled plasma-mass spectrometry (ICP-MS) and microwave induced plasma-optical emission spectrometry (MIP-OES) have been utilized as detectors for GC.5 GC-ICP-MS is widely used since it offers the simultaneous detection of multiple elements at sub-ppt levels and a dynamic range of up to eight orders of magnitude. However, the cost of running ICP-MS is very high due to the consumption of electric power and plasma gas at more than 800 W and 20 L respectively.
In this study, we have developed a GC detector that utilizes microplasma as an ionization source. We named this detector the He Plasma Ionization Detector (HPID). By using microplasma, we could downsize the device and reduce the maintenance costs due to the low consumption of electric power (a few watts) and plasma gas (a few tens of milliliters per minute).6 Simple rod-shaped electrodes and DC-powered glow discharge were used for microplasma generation because prolonged existence and the environmental stability of the plasma are very important for its analytical use.7,8 He was used as the plasma gas because its ionization (24.58 eV) and excitation (19.82, 20.62 eV) energies are the highest of all the elements, and thus, He plasma can effectively ionize and excite all elements, including the nonmetallic and halogen elements.9,10 In this paper, fundamental investigations of the HPID are reported.
2. Experimental
2.1 Configuration and detection principle of the HPID
The configuration of the HPID is shown in Fig. 1. The HPID consists of a discharge region, ionization region and collector electrode. He was introduced from the end of the tube into the discharge region. In the discharge region, two rod-shaped 0.5 mm-diameter electrodes are fixed at a distance of 1 mm from each other. The distance between the electrodes was adjusted by considering the analytical sensitivity and stability of the plasma. He plasma is ignited by a high DC voltage and metastable He atoms, which have a high excitation energy (19.8 eV), and He ions, which have the highest ionization energy among all the elements (24.6 eV), are generated. These metastable atoms and ions reach the ionization region due to the plasma flow, and they are mixed with the carrier gas which contains the sample. The carrier gas and plasma gas flow rates were optimized (carrier gas: 30 mL min−1, plasma gas: 30 mL min−1) to maintain the stable plasma. Then the samples were ionized by the collision of these high-energy species. It is estimated that this Penning ionization is the main ionization process in the detector. Vacuum ultraviolet radiation from the He plasma may also contribute to the sample ionization. After the samples were ionized, an ion current passed through the collector electrode, and the current was recorded by a recorder including a preamp (PD202, J-SCIENCE LAB Co., Ltd., Kyoto, Japan). The sample concentration was calculated from the intensity of the ion current.
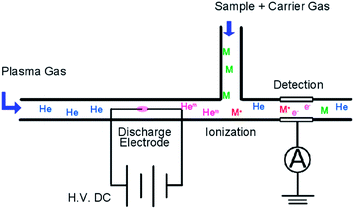 |
| Fig. 1 Schematic diagram of the He plasma ionization detector. | |
2.2 Stabilized high-voltage power supply
A laboratory-made stabilized high-voltage power supply was used. In this power supply, the output current was monitored by measuring the applied voltage to the shunt resistor (200 Ω cement resistor installed on the emitter side of IGBT IXGH32N170H) and was controlled by comparing the applied voltage to the shunt resistor and the TL431 voltage reference. An insulated-gate bipolar transistor (IGBT) was used as a current control element because it can control the high voltage. This power supply was designed for utilizing a maximum voltage of about 600 V and a maximum current of about 50 mA. The voltage between the electrodes was monitored constantly by directly placing a digital multimeter on the electrodes. The value of the voltage applied to the shunt resistor was divided by 200 Ω, and this was considered to be the discharge current of the plasma.
2.3 Investigation of the electrode materials
Superior device durability and stability are very important for developing commercial products from a study model. From this point, optimum materials for the electrodes were investigated. By considering the stability of the plasma, Mo, Th–W (Th 2%), La–W (La 2%), Ce–W (Ce 2%), Pt and Pt–Rh alloys were tested as potential electrode materials. Mo and W were predicted to be durable in the plasma due to their high melting points. Th, La and Ce improved the electron emission of the electrode, and the constant electron emission was expected to maintain a stable discharge. First, the Mo electrode was tested. The configuration of the electrode and the generated plasma are shown in Fig. 2. The generated plasma was a stable atmospheric glow discharge. However, the surface of the electrode changed to a dark color after the discharge. The dark surface material can be removed by washing with sodium hydroxide solution, but this prevents a stable discharge. Th–W, La–W and Ce–W were also tested in the same way. The results showed that the Pt and Pt–Rh alloys are better than the others in terms of chemical stability. Hence, the Pt and Pt–Rh alloy electrodes were utilized.
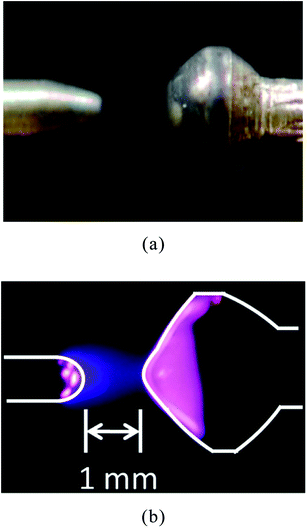 |
| Fig. 2 Structure of electrode (left side: anode, right side: cathode). (a) Magnified view. (b) State of discharge. | |
2.4 Fundamental investigations of the microplasma
The fundamental properties of plasma are defined by the particle density of the atoms, ions and electrons, and their energy.11–17 These are called the plasma parameters, and they are controlled by external conditions such as the gas species, pressure and power input. It is important to measure the relationship between the plasma current and the electrode voltage since it helps to determine the discharge mode of plasma and the optimal conditions necessary for generating a stable plasma. The method for measuring the electrical characteristics is described in 2.1, and the spectroscopic measurement method for determining the excitation temperature is described in this section. To observe the emission from the plasma, an optical fiber was placed close to the gas outlet on the side of the collector electrode. Measurements were performed on a multi-channel spectrometer (HR4000, 424–507 nm, Ocean Optics Inc., FL, USA). The excitation temperature of the plasma was derived from the Boltzmann population distribution among the excitation energy levels. For the He excitation temperature, three emission lines of He1 447.15 nm, 492.193 nm and 501.57 nm were selected. Another multi-channel spectrometer (HR2000, 255–704 nm, Ocean Optics Inc., FL, USA) was used for observing the whole visible light spectrum.
2.5 Analysis of the mixed standard gas sample
A mixture of standard gases, N2, O2, CH4, CO and H2, which is often used in thermal conductivity detector (TCD) analysis, was measured using the HPID. The sample details and analytical conditions are listed in Table 1. An example chromatogram of each gas in the sample mixture obtained using the HPID is shown in Fig. 3.
Table 1 Analytical conditions for the mixed gas sample
GC |
GC Labostage, J-SCIENCE LAB Co., Ltd. |
Column |
MS-5A 60/80 mesh 3 mm (id) × 2.0 m (SUS) |
Column temperature |
60 °C |
Carrier gas flow |
He, 30 mL min−1 |
Volume of sample introduced |
2.0 mL |
Sample |
H2, O2, N2, CH4 and CO 20 ppm/He |
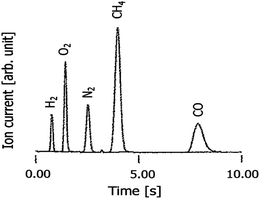 |
| Fig. 3 Chromatogram of H2, O2, N2, CH4 and CO using the HPID. | |
3. Results and discussion
3.1 Electrical characteristics
The voltage–current characteristics of a rod-type electrode discharge are shown in Fig. 4. The electrode voltage did not change much with an increase in the discharge current. Thus, it was hypothesized that the glow discharge was generated in the HPID18,19 when the discharge current was varied from 5 to 30 mA. When the current was above this range, the heat melted the Pt electrode. Hence, the discharge current was limited to less than 30 mA for the HPID analysis.
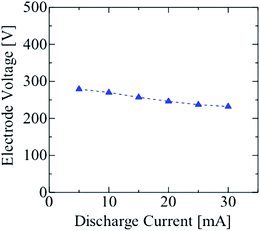 |
| Fig. 4
I–V characteristics of the He microplasma. | |
3.2 Spectroscopic characteristics
A spectrum of the He microplasma at an electrode voltage of 260 V and a discharge current of 15 mA is shown in Fig. 5. Atomic emission from He and H, and molecular emission from OH and N2, could be readily observed. Fig. 6 shows photos of the discharge at different discharge currents. The observations showed that the volume of plasma increased with an increase in the discharge current. The emission intensities of the He atomic lines were measured at different discharge currents, and from these the excitation temperatures were calculated. The results are shown in Fig. 7. From this figure, it can be seen that not only the plasma volume but also the emission intensity and excitation temperature increased with an increase in the discharge current. Hence, it can be expected that the analytical sensitivity improves by increasing the discharge current.
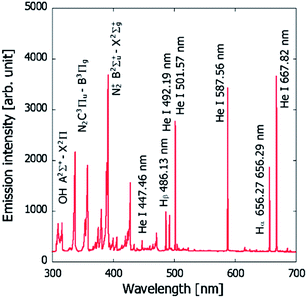 |
| Fig. 5 Emission spectrum of the He microplasma at an electrode voltage of 260 V and a discharge current of 15 mA. | |
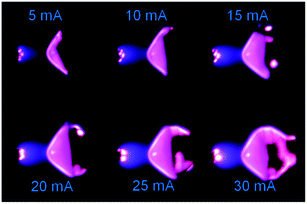 |
| Fig. 6 Photographs of the microplasma generated by a DC power supply. | |
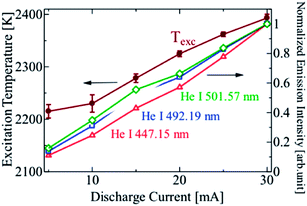 |
| Fig. 7 Dependence of the emission intensity and excitation temperature on the discharge current. | |
3.3 Evaluation of the analytical sensitivity
3.3.1 Effect of the discharge current on analytical sensitivity.
The effect of the discharge current on the analytical sensitivity was investigated. The limit of detection (LOD) of each species at different discharge currents is shown in Fig. 8. LODs were calculated based on S/N = 2, in accordance with the “Guideline for gas chromatography performance descriptions” established by Japan Analytical Instruments Manufacturers' Association (JAIMA).
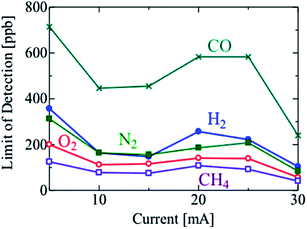 |
| Fig. 8 Limit of detection vs. discharge current. | |
The LODs improved with the increasing discharge current in the range of 5 to 15 mA. However, the LODs decreased at a discharge current of 20 mA, and then started to increase again on increasing the current above 25 mA. To investigate this effect, the signal and noise of the sample chromatograms used for calculating the LODs were plotted separately in Fig. 9 and 10, respectively. The signal intensities of the sample species generally increased with the increase in the discharge current, and were highest at 20 or 25 mA. The bright part of the discharge close to the cathode surface, i.e. the cathode glow discharge, moved frequently on the cathode surface with the fluctuation in the discharge channel, which is the discharge path formed in the gas. The variation in the signal intensity was caused by this fluctuation, and further investigation is needed to reveal the cause of the fluctuation. In the range of 5 to 15 mA, the development of the discharge channels is facilitated by the increasing density of the excited He atoms, due to the increasing output power. Thus, the sample species were ionized more effectively, and the LODs improved. However, the discharge formation becomes unstable at discharge currents above 15 mA. The discharge channel moves around behind the cathode electrode ball, and the position of the discharge channels fluctuates temporarily, thereby causing an increase in the noise. With a further increase in the discharge current, the whole surface of the cathode electrode ball becomes covered with the discharge channel, and the noise decreased; therefore, the LODs improved. The I–V characteristics are not changed by the change of discharge channels or the discharge current, as shown in Fig. 4, because the area of the discharge region on the electrode is constant under certain conditions if the discharge channels fluctuate, and increases smoothly with an increase in the discharge current. It was found that the lifetime of the electrodes decreased because the electrodes were excessively heated at 30 mA. It was confirmed that a stable discharge can be maintained without damaging the electrodes even after operation for several days, at a discharge current of <10 mA. Thus, the optimal discharge current for practical use is 10 mA. To improve the sensitivity further, the discharge current and the cathode surface need to be larger to prevent the discharge channel from moving behind the cathode electrode.
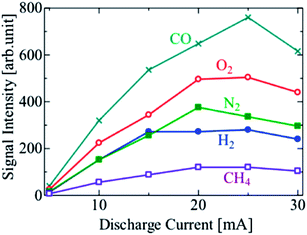 |
| Fig. 9 Signal intensity vs. discharge current. | |
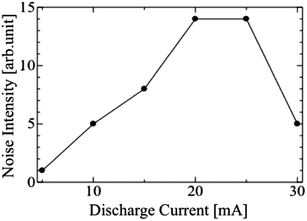 |
| Fig. 10 Noise intensity vs. discharge current. Rapid vertical baseline fluctuation was evaluated by calculating standard deviation and this was regarded as the noise. | |
3.3.2 Linearity of the calibration curve.
The linearity of the calibration curve is an important factor for evaluating the analytical ability of the GC detector. N2, O2, CH4, CO and H2 were analyzed by GC at a range of 10 to 1000 ppm, and a calibration curve was plotted for each of the sample species. Linear calibration curves for the sample species, with correlation coefficients R2 = 0.996–0.999, were obtained. As an example, the calibration curve of H2 is shown in Fig. 11.
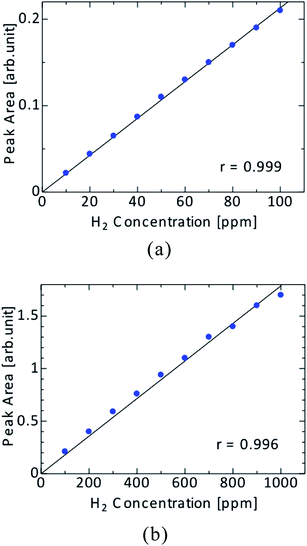 |
| Fig. 11 Linearity of hydrogen. (a) 10–100 ppm. (b) 100–1000 ppm. | |
4. Conclusion
In this study, to realize a high-sensitivity and versatile GC detector that does not exhibit the disadvantages of conventional detectors, we developed the HPID which utilizes DC glow discharge for sample ionization using simple rod-type electrodes. The HPID consumes little power (a few watts) and the discharge is quite stable. The fundamental properties of the DC glow discharge were investigated by spectroscopic methods, and it was found that the emission intensity of He and the excitation temperature, which is related to the analytical sensitivity, increased with the increasing discharge current. The analysis of a sample mixture of standard gas was performed by the HPID, and the LODs for H2, O2, N2, CH4 and CO were 42 ppb, 21 ppb, 37 ppb, 20 ppb and 67 ppb, respectively. The HPID’s sensitivity was more than 1000 times higher than that of the conventional TCD (LOD about 100 ppm). The HPID can detect all gas compounds very easy and overcomes the disadvantage of TCD due to its high sensitivity. Moreover, the calibration curves of each sample species were made with enough linearity. The effectiveness of the HPID for GC was demonstrated by these results. To further improve the analytical ability, the use of a pulse power supply to HPID is recommended because high-density plasma can be generated without the electrode overheating. However, increasing the input power is difficult because of the serious problems of depletion and erosion of the electrodes when using a DC power supply superimposed with the pulse. Therefore, a high-heat-sustaining electrode configuration, materials and power system should be investigated.
References
- R. C. Pitkethly, Anal. Chem., 1958, 30, 1309 CrossRef CAS.
- J. E. Lovelock, J. Chromatogr., A, 1958, 1, 35 CrossRef CAS.
-
R. Grob and E. F. Barry, in Modern practice of gas chromatography, J. Wiley & Sons, New York, 2004 Search PubMed.
- R. S. Braman and A. Dynako, Anal. Chem., 1968, 40, 95 CrossRef CAS.
- J. C. A. Wuilloud, R. G. Wuilloud, A. P. Vonderheide and J. A. Carso, Spectrochim. Acta, Part B, 2004, 59, 755 CrossRef PubMed.
- D. J. Sturges and H. J. Oskam, J. Appl. Phys., 1964, 35, 2887 CrossRef CAS.
- K. H. Schoenbach, R. Verhappen, T. Tessnow, F. E. Peterkin and W. W. Byszewski, Appl. Phys. Lett., 1996, 68, 13 CrossRef CAS.
- K. H. Schoenbach, A. El-Habachi, W. Shi and M. Clocca, Plasma Sources Sci. Technol., 1997, 6, 468 CrossRef CAS.
- R. H. Stark and K. H. Schoenbach, Appl. Phys. Lett., 1999, 74, 3770 CrossRef CAS.
- R. H. Stark and K. H. Schoenbach, Appl. Phys., 1999, 85, 2075 CAS.
- M. H. Adballah and J. M. Mermet, Spectrochim. Acta, Part B, 1982, 37, 391 CrossRef.
- G. R. Kornblum and L. de Galan, Spectrochim. Acta, Part B, 1974, 29, 249 CrossRef.
- W. L. Wiese, M. W. Smith and B. M. Glennon, Atomic transition probabilities (H through Ne – A critical data compilation), Natl. Stand. Ref. Data Ser., 1966 Search PubMed.
- Bur. Stand., NSRDS-NBS 4, 1, US Government Printing Office, Washington DC, 1966.
-
H. R. Griem, in Plasma Spectroscopy, McGraw-Hill, New York, 1964 Search PubMed.
-
H. R. Griem, in Plasma Spectral Line Broadening by Plasma, Academic Press, New York, 1974 Search PubMed.
- C. R. Vidal, J. Cooper and E. W. Smith, Astrophys. J., Suppl. Ser., 1973, 214(25), 37 CrossRef.
- S. Kanazawa, M. Kogoma, T. Morisawa and S. Okazaki, J. Phys. D: Appl. Phys., 1988, 21, 838 CrossRef CAS.
- D. Staack, B. Farouk, A. Gutsol and A. Fridman, Plasma Sources Sci. Technol., 2005, 14, 700 CrossRef CAS.
|
This journal is © The Royal Society of Chemistry 2014 |