DOI:
10.1039/C3GC41997E
(Paper)
Green Chem., 2014,
16, 2174-2184
Biodegradability of 27 pyrrolidinium, morpholinium, piperidinium, imidazolium and pyridinium ionic liquid cations under aerobic conditions†
Received
24th September 2013
, Accepted 15th January 2014
First published on 15th January 2014
Abstract
The chemical and thermal stability of ionic liquids (ILs) makes them interesting for a large variety of applications in nearly all areas of the chemical industry. However, this stability is often reflected in their recalcitrance towards biodegradation, which comes with the risk of persistence when they are released into the environment. In this study we carried out a systematic investigation of the biodegradability of pyrrolidinium, morpholinium, piperidinium, imidazolium and pyridinium-based IL cations substituted with different alkyl or functionalised side chains and using halide counterions. We examined their primary degradability by specific analysis and/or their ultimate biodegradability using biochemical oxygen demand tests according to OECD guideline 301F. Biological transformation products were investigated using mass spectrometry. A comparison of the biodegradation potential of these ILs shows that for all five head groups, representatives can be found that are readily or inherently biodegradable, thus permitting the structural design of ILs with a reduced environmental hazard.
Introduction
Ionic liquids
Ionic liquids (ILs) are chemicals with an outstanding design potential. Their components – cationic and anionic molecular core structures with different side chains – can be combined in innumerable ways to create substances with tailor-made physico-chemical properties important for industrial applications. Most of the compounds possess a very low vapour pressure and non-flammability, which are key properties for improved operational safety in comparison with conventional volatile organic solvents.
Depending on the combination of cations and anions, they can be thermally stable, can be liquid over a wide temperature range and can have a large electrochemical window, which makes them useful for a variety of technical applications in different fields. Initially used as solvents for synthesis and catalysis in clean technologies, they have been increasingly investigated during the last 15 years.1–3 Several industrial processes have been established in which the use of ILs is highly advantageous with respect to the productivity of the processes.4,5 Their range of potential applications extends from the already-mentioned usage as solvents and catalysts to media for electrochemical applications and biosensors.6–9 ILs have also been considered for utilisation in analytical10–13 or supercritical fluid applications,14 gel production15 or as pharmaceutical ingredients.16,17 Their application as agents for recovery, extraction and purification purposes18–20 or as lubricants21 and hypergolic rocket fluid22 is also being discussed.
Sustainability considerations
The variety of ILs available for an individual process together with their high operational safety is not only advantageous for the performance and handling of the process, but also for sustainable development.23 In this context the environmental compatibility of a chemical also plays a major role. The production of substances that simultaneously possess low toxicity and environmental non-persistence is a demanding task for chemists dedicated to sustainable chemistry.24 Nevertheless, its fulfilment appears feasible in the context of the enormous design potential of ILs.25–27 The structural aspects that influence the toxicity of ILs have already been identified and have been replaced by less toxic arrangements in one application.28
Biodegradable structural elements
Many different studies of ILs for the identification of structural elements which support their overall biological degradation have recently been reviewed.3,27,29–31
The biodegradation potential of ILs ranges widely from very good to very poor. Good primary biodegradability has been observed in ILs with longer alkyl side chains (>C6) at the cationic core, whereas the same head group with shorter side chains is only poorly biodegradable: compare, for example, 1-octyl-3-methyl-1H-imidazolium (C8mim) with 1-ethyl-3-methyl-1H-imidazolium (C2mim) combined with halide anions (Cl, Br).32,33 Imidazolium-based ILs in general exhibit reduced biodegradability with respect to their core structure,32,34 whereas 1-alkyl-3-methylpyridinium-based ILs have an elevated biodegradation potential,32,35 and many compounds have been classified as readily biodegradable. Apart from the (bio)degradability of the cation, the behaviour of the anion also has to be taken into account. Anions such as PF6− and BF4− are sensitive to hydrolytic processes,36 and organic anions such as acetate, and ethyl and octyl sulphate, are readily biodegradable.37,38 In contrast, anions such as N(CN)2−, B(CN)4− and (CF3SO2)2N− are known to be neither hydrolytically degradable nor biodegradable under environmental conditions.39,40 The choice of inoculum using pure cultures, such as Corynebacterium sp. and Sphingomonas paucimobilis, and the presence or absence of degraders in mixed cultures additionally influence the results towards an enhanced biodegradability of the ionic liquid cation.41–43
Thus, a broad biodegradability range of ILs can be created depending (i) on the structural composition with respect to the cationic head group, side chain and counterion, and (ii) on the experimental procedure used for the determination of biodegradability data, more specifically the detection either by a specific analytical procedure or sum parameters, the chosen environmental condition and the choice of microorganisms.
The focus of the study is on the influence of the structural composition and the design potential of ILs.
Missing data
The identification of the biodegradable substructures of ILs has been a mammoth task. Although many commercially available pyridinium and imidazolium-based ILs have been tested, some relevant data for a systematic investigation of other head groups are still missing. To date only N-alkyl-N-methylmorpholinium and 4-benzyl-4-methylmorpholinium have been examined, head groups that are not readily biodegradable.44 A few phosphonium ILs with alkyl and functionalised side chains were also refractory to biological degradation under ready biodegradability test conditions.45,46
As yet, no systematic investigation of the biodegradability of other head groups has been undertaken. We therefore examined a set of 27 ILs with differently substituted head groups – pyrrolidinium, morpholinium and piperidinium ILs – together with some pyridinium and imidazolium ILs (Tables 1–6). We screened the primary biodegradability of IL cations, tracking the course of degradation using liquid chromatography, determined (where possible) transformation products with mass spectrometry, and investigated the extent of mineralisation of selected ILs by applying the sum parameter biochemical oxygen demand (BOD). Several of the IL cations under investigation were fully degraded and some of them match the criteria for being classified as readily biodegradable. In experiments with a prolonged standard test duration (>28 d) some more compounds underwent biodegradation, showing the importance of microbial adaptation. Even during the degradation of one IL, microbial adaptation occurred as diauxic growth when head groups and side chains were successively biodegraded.
Table 1 Structural formula and results of pyrrolidinium-based ionic liquid cations obtained from primary and BOD experiments
Cationic head group |
Side chain R |
Anion |
Substance identifier |
Classification OECD and biotic hydrolysis |
Primary degradation in % (28 d) (n = 2) |
Relative oxygen demand BOD/ThOD in % (28 d) (n = 2) |
Reference |
|
|
Cl− |
C8mpyr Cl |
Readily biodegradable |
100 |
69 ± 1 |
This study |
|
Cl− |
C3OHmpyr Cl |
Readily biodegradable |
100 |
67 ± 3 |
This study |
|
Br− |
C1COO2mpyr Br |
Inherently, ultimately biodegradable |
100 |
28 d: 34 ± 11 (n = 4) |
This study |
|
|
|
|
38 d: 74 ± 0.1 (n = 4) |
|
|
Br− |
C4mpyr Br |
Inherently, ultimately biodegradable |
— |
28 d: 14 ± 13 42 d: 70 ± 18 |
This study |
|
Cl− |
C1CNmpyr Cl |
Biotic hydrolysis of the cyano group |
Qualitative analysis by mass spectrometry |
2 ± 2 |
This study |
|
I− |
C2OHmpyr I |
Not readily, but hints of being inherently |
28 d: 8 ± 5 |
28 d: 6 ± 9 (n = 4) |
This study |
|
|
|
43 d: 25 ± 21 |
60 d: 42 ± 39 (n = 4) |
|
|
CH3CH2OSO3− |
C2mpyr C2OSO3 |
Not readily biodegradable |
28 d: −2 ± 3 |
— |
This study |
|
|
|
40 d: −3 ± 8 |
|
|
Table 2 Structural formula and results of morpholinium-based ionic liquid cations obtained from primary and BOD experiments
Cationic head group |
Side chain R |
Anion |
Substance identifier |
Classification OECD (…biodegradable) and biotic hydrolysis |
Primary degradation in % (28 d) (n = 2) |
Relative oxygen demand BOD/ThOD in % (28 d) (n = 2) |
Reference |
|
|
Cl− |
C3OHmmor Cl |
Inherently |
28 d: 12 ± 8 |
28 d: 31 ± 16 |
This study |
|
|
|
|
41 d: 59 ± 10 |
|
|
Cl− |
C1CNmmor Cl |
Biotic hydrolysis of the cyano group |
54 ± 13 & Qualitative analysis by mass spectrometry |
0 |
This study |
|
I− |
C2OHmmor I |
Not readily |
28 d: 17 ± 1 |
28 d: −1 ± 1 (n = 4) |
This study |
|
|
|
|
60 d: 4 ± 1 (n = 4) |
|
|
Cl− |
C1O2mmor Cl |
Not readily |
−3 ± 4 |
— |
This study |
|
Cl− |
C2O1mmor Cl |
Not readily |
1 ± 4 |
— |
This study |
|
Br− |
C2O2mmor Br |
Not readily |
0 ± 8 |
— |
This study |
|
Br− |
C4mmor Br |
Not readily |
−3 ± 4 |
— |
This study |
Table 3 Structural formula and results of piperidinium-based ionic liquid cations obtained from primary and BOD experiments
Cationic head group |
Side group R |
Anion |
Substance identifier |
Classification OECD (…biodegradable) and biotic hydrolysis |
Primary degradation in % (28 d) (n = 2) |
Relative oxygen demand BOD/ThOD in % (28 d) (n = 2) |
Reference |
Not within a 10-day window.
|
|
|
Cl− |
C3OHmpip Cl |
Inherently and ultimate |
— |
79 ± 2a |
This study |
|
Cl− |
C2OHmpip Cl |
Inherently |
— |
28 d: 29 ± 3 |
This study |
|
|
|
|
60 d: 85 ± 1 |
|
|
Cl− |
C1CNmpip Cl |
Biotic hydrolysis of the cyano group |
Qualitative analysis by mass spectrometry |
5 ± 2 |
This study |
|
Cl− |
C1O2mpip Cl |
Not readily |
−2 ± 2 |
— |
This study |
|
Cl− |
C2O1mpip Cl |
Not readily |
1 ± 4 |
— |
This study |
|
(CF3SO2)2N− |
C3mpip (CF3SO2)2N |
Not readily |
— |
3 ± 2 |
This study |
|
Br− |
C4mpip Br |
Not readily |
— |
−4 ± 3 |
This study |
Table 4 Structural formula and results of pyridinium-based ionic liquid cations obtained from primary and BOD experiments. The data from this study are complemented with data from the literature
Cationic head group |
Side chain R |
Anion |
Substance identifier |
Classification OECD (…biodegradable) and biotic hydrolysis |
Primary degradation in % (28 d) (n = 2) |
Relative oxygen demand BOD/ThOD in % (28 d) (n = 2) |
Reference |
|
|
I− |
C2OHpy I |
Inherently and ultimately |
100 |
65 ± 10 |
This study |
|
Cl− |
C3OHpy Cl |
Inherently |
— |
51 ± 0 |
This study |
|
Cl− |
C1CNpy Cl |
Biotic hydrolysis of the cyano group |
Qualitative analysis by mass spectrometry |
−2 ± 0 |
This study |
|
Cl− |
C2py Cl |
Not readily |
0 |
— |
33
|
|
Br− |
C3py Br |
Not readily |
— |
1 ± 0 |
This study |
|
Br− |
C4py Br |
Not readily |
— |
2 |
34
|
Table 5 Structural formula and results of imidazolium-based ionic liquid cations (functionalised side chains and an octyl side chain) obtained from primary and BOD experiments. The data from this study are complemented with data from the literature
Cationic head group |
Side chain R |
Anion |
Substance identifier |
Classification OECD (…biodegradable) and biotic hydrolysis |
Primary degradation in % (28 d) (n = 2) |
Relative oxygen demand BOD/ThOD in % (28 d) (n = 2) |
Reference |
|
|
Cl− |
C8mim Cl |
Inherently |
100 |
— |
33
|
|
Br− |
C1COO2mim Br |
Inherently |
— |
21 |
67
|
|
Cl− |
C1CNmim Cl |
Biotic hydrolysis of the cyano group |
0 |
— |
33
|
|
|
|
Qualitative analysis by mass spectrometry |
0 |
This study |
|
I |
C2OHmim I |
Not readily |
0 |
— |
33
|
|
Cl− |
C3OHmim Cl |
Not readily |
0 |
— |
33
|
|
Cl− |
C1O2mim Cl |
Not readily |
0 |
— |
33
|
|
Cl− |
C2O1mim Cl |
Not readily |
0 |
— |
33
|
|
Br− |
C2O2mim Br |
Not readily |
0 |
— |
33
|
Table 6 Structural formula and results of imidazolium-based ionic liquid cations (alkyl side chains) obtained from primary and BOD experiments. The data from this study are complemented with data from the literature
Cationic head group |
Side chain R |
Anion |
Substance identifier |
Classification OECD (…biodegradable) and biotic hydrolysis |
Primary degradation in % (28 d) (n = 2) |
Relative oxygen demand BOD/ThOD in % (28 d) (n = 2) |
Reference |
|
|
Cl− |
C2mim Cl |
Not readily |
0 |
— |
33
|
|
PF6− |
C3mim PF6 |
Not readily |
— |
2 ± 1 |
This study |
|
Cl− |
C4mim Cl |
Not readily |
0 |
— |
33,38,46,68
|
Our systematic studies of the biodegradability of ILs are addressed to the users of ILs in different fields of application to facilitate the selection of environmentally favourable structural elements and hence to contribute to the design of inherently safer ILs.
Materials and methods
Chemicals
All the tested ILs as well as the salts for the mineral salt medium and the standard eluent for ion chromatographic analysis were obtained from Merck KGaA (Darmstadt, Germany). Acetonitrile (HPLC grade) for the ion chromatographic measurements was obtained from VWR International GmbH (Darmstadt, Germany).
Biodegradation tests
1 Manometric respirometry.
The manometric respirometry test was performed according to OECD guideline 301F.47 The biological oxygen demand (BOD) of the substance was determined for 28 d (but prolonged as soon as a significant increase in oxygen consumption had been detected) using a BOD measurement system (OxiTop©, thermostatically controlled from WTW GmbH, Weilheim, Germany) with two replicates for each test substance. The extent of mineralisation could be inferred from this test: if 60% biodegradation was exceeded within a certain time frame, the compound was classified as “readily biodegradable”.
The activated sludge was taken from the municipal wastewater treatment plant in Delmenhorst (Germany), filtered through grade 1288 filter discs (Sartorius AG, Göttingen) and aerated for ten days to remove organic substances from sewage treatment before inoculation. The medium was prepared with 8.5 mg L−1 KH2PO4, 28.5 mg L−1 K2HPO4·3H2O, 33.4 mg L−1 Na2HPO4·2H2O, 0.5 mg L−1 NH4Cl, 36.4 mg L−1 CaCl2·2H2O, 22.5 mg L−1 MgSO4·7H2O and 0.25 mg L−1 FeCl3 (pH 7.4). An additional 1.16 mg L−1 allylthiourea has been added in order to inhibit nitrification. The bacteria suspension was set up using 20% supernatant from the aerated activated sludge and 80% mineral salt medium solution. The concentrations of the test substances were chosen according to their expected oxygen demand, which were in a non-inhibitory concentration, usually below 850 μmol L−1.48 Moreover, blank samples (inoculated media without test substance) and controls (inoculated media with benzoic acid) were also prepared. In this test a bacteria number of 104 cells L−1 was applied (determined using a Paddle Tester; Hach Europe, Düsseldorf). Sodium hydroxide was used to absorb the evolved carbon dioxide inside the sample vessels. The vessels were closed with gas-tight stoppers and stored in the dark at 20 ± 0.5 °C. The oxygen consumption was determined manometrically. Biodegradation of the test substance was calculated by the biological oxygen uptake (BOD) for the test substance (corrected by the oxygen demand of the blank samples) with respect to the theoretical oxygen demand (ThOD) of the substance and the amount of substance present in the sample. For the calculation of ThOD the cation was considered without including its inorganic halide anion. The chemical formula can be given as CcHhNnOoPp (eqn (1)):
|  | (1) |
The reference substance benzoic acid was always degraded within 10 d, consuming around 80% of the ThOD and showing that the test was working reliably.
2 Primary biodegradation.
The stock solutions of the test substances were prepared in deionised water. Each test substance was used at a final concentration of 50 μmol L−1 in each of the two replicates of the sample vessels. The medium and sample vessels were prepared as described above. Additionally, one sample vessel was prepared for each experimental set up with aniline as positive control at a final concentration of 1.07 mmol L−1 (100 mg L−1). Aniline was used as a reference substance for positive biodegradability, since aniline is known to be biodegradable under the chosen test conditions within 14 d.69 In this study aniline was fully biodegraded within ten days (limit of detection <5 μmol L−1, data not shown), indicating the general biological activity of the inoculum. The vessels were stirred continuously in the dark with a magnetic bar. To keep the volume constant, evaporated water was frequently replaced with an equal volume of deionised water.
The concentration of the test substance was determined by ion chromatography. The primary biodegradation was then calculated from the concentration of the substance on a specific day in comparison to that on day zero. The maximum deviation was calculated from the standard deviations of the ion chromatographic measurement according to common error propagation.
Examination of the analyte concentration and metabolites
Sampling and sample preparation for instrumental analysis.
The samples for the specific analysis were taken on the first day and thereafter at short time intervals for 28 d. On each testing day, 6 mL of the sample was centrifuged at approx. 3000g (Labofuge 400R, Heraeus Instruments GmbH, Germany) in 15 mL polypropylene centrifuge tubes (Sarstedt AG & Co., Germany). The samples were stored in a freezer at −20 °C until all of the samples had been taken. The final specific analysis of the IL cation was then conducted by ion chromatography.
1 Ion chromatography.
The ion chromatograph used was a “Metrohm 881 Compact IC pro” equipped with a “Metrohm 850 Conductivity Detector” connected to a “Metrohm 863 Compact Autosampler” (Metrohm AG, Switzerland). The “Metrosep C 4 50” cation exchange column with a “Metrosep C 4 50 Guard” pre-column was kept at 30 °C in a column oven. The analytical method was developed on the basis of previous studies.49
5 mmol L−1 nitric acid (HNO3) with 25% acetonitrile (ACN) were used as an eluent. The device was run at a flow rate of 0.9 mL min−1 and the injection volume of each sample was 20 μL. The analytical method was developed to analyse most of the IL cations with one eluent solution. The data were evaluated using standard “Magic Net 1.1” software. An external standard for each substance was run during each measurement sequence to identify the IL peak and to check the consistency of the analytical method. Analytical quality parameters have already been published for other IL cations – these are generally in the range of <0.33 μM and <1 μM for the limit of detection and limit of quantification, respectively.49
2 Liquid chromatography–mass spectrometry.
In order to analyse potential degradation products, liquid chromatographic separation combined with mass spectrometric analysis was subsequently undertaken if necessary. The samples were diluted in methanol and deionised water (50
:
50) and analysed using an HP 1100 HPLC coupled to a Bruker Esquire ESI-MS ion trap detector. The chromatograph was run with 55% of 5 mmol L−1 sodium formate pH 3.4 and 45% ACN at a flow rate of 0.5 mL min−1. Column: Multohigh 150 × 4 mm, 100 Si-5 μ Hilic (CS-Chromatographie Service GmbH, Langerwehe, Germany).
Mass spectra for cations were acquired in the positive ion mode in the scan range of m/z+ 50–200. The ESI source conditions were set with a drying gas flow rate of 11 L min−1, a drying gas temperature of 350 °C and the nebulizer at 70 psi. Generally, all samples after the biodegradation experiments have been investigated with LC–MS to identify (if applicable) transformation products.
3 Interpretation of the data.
The following effects that can lead to a false interpretation of the data were excluded:
(1) Excluding false positive results: the declaration of an IL as biodegradable although it is not may be due to the adsorption of the analyte on the bacteria and glass vessels, as well as to systematic deviations of the analytical test system. However, adsorption effects would only be observed within the first few days50 and often in samples with a high organic load. As we used a negligible amount of sewage sludge flocs in the filtered test solutions, no adsorption effects were observed in our experiments. Systematic deviations in the analytical measurements were reduced by adjusting the results so as to relate them to the external standards run with each analytical sequence. Substances detected as being primarily biodegradable were further examined for their full biodegradability to confirm the results of the first run.
(2) Excluding false negative results: apart from the stringent test conditions, inhibition effects can also lead to a false classification of the data as “non-primarily biodegradable”. However, these can be excluded at the concentrations used in the experiments. In a recent study the inhibitory potential towards activated sludge organisms of a wide structural variety of ILs was found to be low with inhibitory concentrations usually higher than 1500 μM.48 At a concentration of 50 μM in the primary biodegradation tests and below 850 μmol L−1 in BOD tests, inhibitory effects are therefore unlikely to be observed.
Results
The primary biodegradation of the test substances under aerobic conditions was monitored for 28 d. Specific analysis by ion chromatography (IC) using a conductivity detector yielded information on the concentration of the IL cation at a specific time of the experiment. If an IL cation was not degraded we assumed that it was “not readily biodegradable” in accordance with OECD 301. If primary biodegradation was observed, we used WTW OxiTop® devices measuring the biochemical oxygen demand (BOD) to determine the full mineralisation of the IL. In this full mineralisation test a compound was classified as readily biodegradable if it exhibited >60% degradation in relation to the theoretical oxygen demand (ThOD) within 28 d and an exponential growth phase of 10 d. In this study this result was attributable solely to the cation, because most of the IL anions investigated contained inorganic moieties (“halides”) (Tables 1–6) that were irrelevant to biodegradation tests based on the measurement of oxidisable carbon in the molecule. The same held true for PF6− and the non-biodegradable anion (CF3SO2)2N−.
The results of the primary biodegradation and full mineralisation experiments are given in Tables 1–6. For some ILs the standard test duration of 28 d was prolonged (in total up to 60 d) to investigate their long-term behaviour.
The results from primary degradability, the degradation curves derived from BOD measurements as well as more detailed information on the used classification are given in the ESI† file.
Biodegradability of head groups
1 Pyrrolidinium compounds.
In primary and mineralisation experiments the biodegradation rate of pyrrolidinium compounds varied between 0 and 100% and was dependent on the substituted side chain (Table 1). No biodegradation was found for the ethyl (C2mpyr C2OSO3) derivative, whereas C3OHmpyr Cl and C8mpyr Cl could be classified as readily biodegradable, exhibiting >60% degradation within 28 d. C1COO2mpyr Br was completely primarily degraded within one week, but although 20% of the ThOD was attained after just 5 d, no further degradation took place until the end of the standard experiment (28 d). The test procedure was prolonged, however, and after 38 d the whole compound was fully mineralised (74%) (Fig. 1).
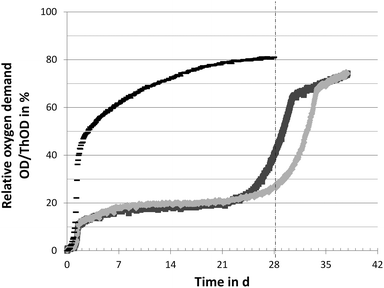 |
| Fig. 1 Biodegradation curves (duplicates in grey: and ) derived from BOD measurements for Pyr11COO2 Br. The black line (—) represents the reference compound benzoic acid. | |
The MS measurements of samples taken from the BOD experiment showed that after 5 and 28 d no parent compound could be detected any more, but that a degradation product with m/z+ = 144 and the corresponding sodium and potassium adducts (m/z+ = 144 + 23 − H+ = 166 and m/z+ = 144 + 39 − H+ = 182) were formed (data not shown). This suggests that the ester bond was enzymatically hydrolysed (in abiotic samples the compound remained stable; data not shown) and C1COOHmpyr (m/z+ = 144) was released and fully mineralised after an additional adaptation period of 20 d (Fig. 1). Degradation rates below 25% and strong deviations between replicates were observed for C2OHmpyr I and C4mpyr Br; however, prolonging the test to 60 d enabled both compounds to be mineralised. According to OECD, C1COO2mpyr Br and C4mpyr Br could be classified as “not readily” but “inherently biodegradable”, i.e. the chemicals have potential for biodegradation under aerobic conditions. For C2OHmpyr I the deviations between multiple runs and experiments were strong even after prolonging the test duration (around 40%) so that this compound can only be classified as “not readily”, but with hints of being inherently biodegradable. Primary degradation, but no BOD was observed for C1CNmpyr Cl; similar findings have been noted for other head groups with a cyanomethyl side chain (Tables 1–6) and will be presented in a separate paragraph.
2 Morpholinium compounds.
None of the investigated morpholinium compounds showed significant primary biodegradation apart from the C3OHmmor and the C1CNmmor cation (Table 2). At the end of the primary biodegradation with C3OHmmor a slight decrease in concentration (12 ± 8%) was observed, so an additional BOD experiment of prolonged duration was performed. In these experiments oxygen consumption began after 25 d and reached levels from 20 to 42% biodegradation (deviation between replicates). After 41 d total biodegradation of 59 ± 10% degradation was observed, leading to the classification “inherently biodegradable”.
3 Piperidinium compounds.
Among the piperidinium compounds tested only C3OHmpip Cl and C2OHmpip Cl were fully mineralised (Table 3). Pip13OH Cl was degraded up to 79 ± 2% after 28 d. The 60% pass level was exceeded, but not within a ten day window. The compound was thus classified as “inherently, ultimately biodegradable” rather than “readily biodegradable”. The biodegradation of C2OHmpip Cl did not exceed the 60% pass level after 28 d, but after a prolongation of the test duration to 60 d more than 80% degradation was observed, leading to the classification “inherently biodegradable”.
4 Pyridinium compounds.
The N-alkylpyridinium cations (C2py, C3py and C4py) did not exhibit significant levels of biodegradation, whereas the hydroxylated compounds (C2OHpy and C3OHpy) showed good biodegradation rates of 65 ± 10% and 51 ± 0%, respectively, permitting classification of “inherently biodegradable” (Table 4).
5 Imidazolium compounds.
The corresponding imidazolium-based ILs with similar side chains as used for the experiments described above have already been examined by different working groups (Table 5). In this study the biodegradability of imidazolium cations with propyl side chains was investigated additionally, but no biodegradation was observed for this compound (Table 6).
6 Degradation of the cyanomethyl side chain.
Regardless of the head group, compounds with a cyanomethyl side chain (C1CNmpyr Cl, C1CNmmor Cl, C1CNmpip Cl, C1CNpy Cl, and C1CNmim Cl) exhibited primary degradation within 28 d, but no oxygen consumption in BOD measurements. LC–MS analysis of the 28 d samples revealed the occurrence of several mass-to-charge ratios suggesting the hydrolysis of the CN group. The corresponding carboxamide (m/z+ = 159) and carboxylic acid detected as sodium (m/z+ = 182) and potassium adducts (m/z+ = 198) were observed in the mass spectrum (Fig. 2).
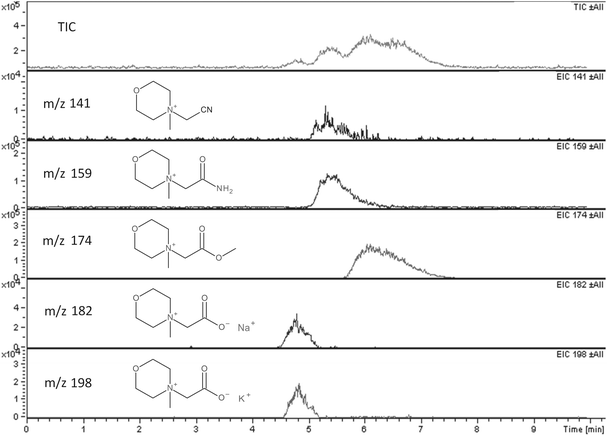 |
| Fig. 2 The total ion current (TIC) over time from LC–MS measurements of the 28 d sample from the BOD biodegradation experiment on C1CNmmor Cl. The extracted ions presumably belong to the molecular structures shown. | |
The methylated cation R+–CH2–C(
O)O–CH3 (Fig. 2) is formed by an esterification reaction of the free acid and with the methanol solvent under analytical conditions (pH 3.5). The same detection pattern was observed for all analogous IL cations: C1CNpy, C1CNmpyr, C1CNmmor, C1CNmim and C1CNmpip. In the standard solutions no such transformation products could be found, indicating that microorganisms are involved in the cleavage. In the case of C1CNmpyr the corresponding acid (C1COOHmpyr) formed was the same transformation product as that obtained in the experiments with C1COO2mpyr. C1COOHmpyr was found to be fully mineralised in a prolonged biodegradation test.
Discussion
Structure–biodegradability relationships
Most of the degradable compounds are among the pyrrolidinium cations. When substituted with hydroxyl-containing side chains and when enough time is allowed, full mineralisation (side chain and core) takes place. The biodegradability of 1-alkyl-1-methylpyrrolidinium compounds increased with lengthening side chain, changing from “not readily biodegradable” (ethyl) to “inherently biodegradable” (butyl) and “readily biodegradable” (octyl). A similar trend has been reported for 1-alkyl-3-methylimidazolium and 1-alkyl-3-methylpyridinium cations.32,33 Unfortunately, the influence of the side chain could not be investigated for the other non-aromatic head groups, since these compounds with an octyl side chain were unavailable.
Good biodegradation rates of IL compounds with substituted hydroxyl side chains were not only obtained for pyrrolidinium cations, but also for morpholinium, piperidinium and pyridinium ones. Several of these compounds were fully mineralised, especially when a hydroxypropyl or even a hydroxyethyl residue was attached. The improved biodegradability of compounds containing alcohol groups – a potential target of enzymatic degradation – has been reported for several other substance classes26 for instance, imidazolium29 and ammonium-based ILs.51 Apart from the above-mentioned hydroxylated compounds, none of the other investigated morpholinium, piperidinium and pyridinium compounds were mineralised. In comparison with the other head groups the imidazolium group exhibited the lowest biodegradation potential. None of the investigated functionalised methyl imidazolium compounds were degraded and only C8mim Cl was primarily degraded. The core proved to be recalcitrant towards biodegradation in former studies and only the side chain was degraded via β-oxidation, yielding 1-(3-carboxyethyl)-3-methyl-imidazolium.33
In this study one odd-numbered alkyl side chain was investigated to check whether β-oxidation then leads to products such as 1-(3-carboxypropyl)-3-methylimidazolium that might be further degraded (full mineralisation of the ring). This hypothesis was not confirmed and the propyl compound showed no biodegradation potential within 28 d.
With respect to the side chains a microorganism enabled primary degradation of the cyanomethyl side chain, and the identified transformation products suggest enzymatic hydrolysis via nitrile degrading enzymes such as nitrilases or nitrile hydratases together with amidase. These enzymes are commonly applied as catalysts in organic synthesis for the hydrolysis of nitrile groups in the pharmaceutical industry and for bioremediation purposes, amongst others.52–55 Recently, IL anions such as N(CN)2−, C(CN)3− and B(CN)4− were hydrolysed to their corresponding amides with isolated nitrile hydratase.56
Variability and adaptation time
The data presented in this study show some inconsistencies in comparison with the literature data, and in some cases there were evident discrepancies between our independently performed experiments (e.g. C2OHmpyr I and C3OHmpyr Cl). Although the rapidly biodegradable reference compound benzoic acid was always fully degraded within the first week, the biodegradation of the chosen xenobiotics seems to be more dependent on the biological variability. In particular, the nature of the inoculum in terms of spatial and temporal variations is a very variable factor in the assessment of biodegradability.57 Hence, during every experiment and within each test vessel a unique microorganism community is present, which may result in completely different degradation results, especially under the stringent test conditions of the OECD guideline.58 For example, we found no primary degradation of the C1CNmim cation in our previous study,33 but this did occur in the present study. The same holds true for observations made for pyridinium compounds. In studies performed independently by Docherty et al.59 and Pham et al.,60 vastly different biodegradation rates and different degradation pathways were found for one IL cation (N-butyl-3-methylpyridinium). In the first study there was no biodegradation within 43 d,32 but after re-examination59 by the same group, mineralisation was nearly complete (88% within 41 d); this was corroborated in primary biodegradation studies by Pham et al.60 In the present study N-butylpyridinium, for example, was investigated and no biodegradation was observed, but whether a different substitution pattern of the pyridinium core or the microorganisms was responsible for degradation/recalcitrance is a question that remains unanswered. The results obtained for C1COO2mpyr suggest that the microbial composition changes during the test (Fig. 1). In the first step the ester is degraded enzymatically, after which there is a long lag phase of around 20 d, followed in the second step by additional exponential growth and another lag phase. It can be assumed that other microorganisms or enzymes are involved in the degradation of the side chain and the subsequent decomposition of the core. Such a symbiosis of different microorganisms from a test community has been described for the biodegradation of aliphatic and aromatic hydrocarbons.61 As a consequence of different substrate availabilities, the subsequent activation of enzymes resulting in the diauxic growth of microorganisms has been successfully modeled for different sugars and organic anions.62
For several compounds (C1COO2mpyr Br, C2OHmpyr I, C4mpyr Br, C2OHmpip Cl, C3OHmmor Cl) the prolonged test duration leads to an increased biodegradation rate. Long-term exposure of microorganisms to ILs is presumably associated with adaptation processes, such as the induction of specific enzymes, genetic mutation or horizontal gene transfer, which enhance the degradative capacity of the entire community or change the population in terms of the selective growth of certain strains.63 Such long-term adaptation processes and an increased biodegradation potential (including the complete degradation of the imidazolium ring) were recently observed for C8mim, too.64 Moreover, cultures of axenic bacterial strains, such as Corynebacterium sp. and Sphingomonas paucimobilis, or isolated enzymes have successfully enhanced the biodegradability of certain IL cations41,42 or IL anions,56 and a wide range of IL structures can be generally targeted for microbial degradation.
All in all, for the screening purposes of this study, those stringent test conditions make it easier to identify ILs with improved biodegradation potential. For the final determination of persistency, further tests need to be conducted.
Conclusion
A comparison of the biodegradation potential shows that for all the five head groups investigated, compounds can be found that are readily or inherently biodegradable. It is highly likely that chemicals classified as “readily biodegradable” are biodegradable in the environment with just a low risk of persistence, or even none at all. This cannot generally be assumed for inherently biodegradable chemicals, although they do have a reduced risk of being persistent. From the structural design point of view, both the type of head group as well as the substituted side chain influence the degradability of the IL cation. When the same side chain is substituted, pyrrolidinium and pyridinium cores generally display better degradation rates in comparison to piperidinium and morpholinium cations. Imidazolium appears to be the most refractory head group. The general ability of microorganisms to adapt to IL cations again reduces the risk of persistence. The degree of mineralisation, however, also seems to depend strongly on the inoculum and the test/environmental conditions. The underlying science behind IL biodegradation, namely the microbiological processes and the microorganisms involved, is not yet well understood, so detailed investigations are needed. The environmental risk of poorly degradable ILs can be eliminated when these are used in closed systems or can be reduced when residues are removed from waste water by separation techniques such as nanofiltration65 or advanced oxidation processes.33,66
Acknowledgements
We would like to thank the UFT Team, especially Carla Sernow for conducting the BOD experiments. We would also like to acknowledge the financial support from the German Federal Foundation for the Environment (Deutsche Bundesstiftung Umwelt (DBU), Osnabrück/Germany), the Foundation of the Universität Bremen (Stiftung der Universität Bremen) and the German Academic Exchange Service (DAAD).
References
- C. Yue, D. Fang, L. Liu and T.-F. Yi, J. Mol. Liq., 2011, 163, 99–121 CrossRef CAS PubMed.
- T. Welton, Chem. Rev., 1999, 99, 2071–2084 CrossRef CAS PubMed.
- M. Petkovic, K. R. Seddon, L. P. N. Rebelo and C. Silva Pereira, Chem. Soc. Rev., 2011, 40, 1383–1403 RSC.
-
A. Kokorin, Ionic liquids: Applications and Perspectives, InTech, Rijeka, Croatia, 2011 Search PubMed.
- N. V. Plechkova and K. R. Seddon, Chem. Soc. Rev., 2008, 37, 123–150 RSC.
- T. Tsuda, K. Kondo, T. Tomioka, Y. Takahashi, H. Matsumoto, S. Kuwabata and C. L. Hussey, Angew. Chem., Int. Ed., 2011, 50, 1310–1313 CrossRef CAS PubMed.
- W. Sun, C. X. Guo, Z. Zhu and C. M. Li, Electrochem. Commun., 2009, 11, 2105–2108 CrossRef CAS PubMed.
- D. Wei and A. Ivaska, Anal. Chim. Acta, 2008, 607, 126–135 CrossRef CAS PubMed.
- V. V. Singh, A. K. Nigam, A. Batra, M. Boopathi, B. Singh and R. Vijayaraghavan, Int. J. Electrochem., 2012, 2012, 1–19 Search PubMed.
- Z. Tan, J. Liu and L. Pang, TrAC, Trends Anal. Chem., 2012, 39, 218–227 CrossRef CAS PubMed.
- P. Sun and D. W. Armstrong, Anal. Chim. Acta, 2010, 661, 1–16 CrossRef CAS PubMed.
- A. Berthod, M. J. Ruiz-Angel and S. Carda-Broch, J. Chromatogr., A, 2008, 1184, 6–18 CrossRef CAS PubMed.
- R. Zhang, N. Li, C. Wang, Y. Bai, R. Ren, S. Gao, W. Yu, T. Zhao and H. Zhang, Anal. Chim. Acta, 2011, 704, 98–109 CrossRef CAS PubMed.
- S. Keskin, D. Kayrak-Talay, U. Akman and Ö. Hortaçsu, J. Supercrit. Fluids, 2007, 43, 150–180 CrossRef CAS PubMed.
- K. M. S. Meera, R. M. Sankar, S. N. Jaisankar and A. B. Mandal, Colloids Surf., B Biointerfaces, 2011, 86, 292–297 CrossRef CAS PubMed.
- M. Moniruzzaman and M. Goto, J. Chem. Eng. Jpn., 2011, 44, 370–381 CrossRef CAS.
- W. L. Hough, M. Smiglak, H. Rodríguez, R. P. Swatloski, S. K. Spear, D. T. Daly, J. Pernak, J. E. Grisel, R. D. Carliss, M. D. Soutullo, J. H. Davis, Jr. and R. D. Rogers, New J. Chem., 2007, 31, 1429–1436 RSC.
- R. Vijayaraghavan, N. Vedaraman, M. Surianarayanan and D. R. MacFarlane, Talanta, 2006, 69, 1059–1062 CrossRef CAS PubMed.
- M. G. Freire, A. F. M. Cláudio, J. M. M. Araújo, J. A. P. Coutinho, I. M. Marrucho, J. N. Canongia Lopes and L. P. N. Rebelo, Chem. Soc. Rev., 2012, 41, 4966–4995 RSC.
- P. J. Carvalho and J. A. P. Coutinho, Energy Environ. Sci., 2011, 4, 4614–4619 CAS.
- F. Zhou, Y. Liang and W. Liu, Chem. Soc. Rev., 2009, 38, 2590–2599 RSC.
- S. Schneider, T. Hawkins, Y. Ahmed, M. Rosander, L. Hudgens and J. Mills, Angew. Chem., Int. Ed., 2011, 50, 5886–5888 CrossRef CAS PubMed.
- M. Deetlefs and K. R. Seddon, Green Chem., 2010, 12, 17–30 RSC.
- P. Anastas and N. Eghbali, Chem. Soc. Rev., 2010, 39, 301–312 RSC.
- B. Jastorff, K. Mölter, P. Behrend, U. Bottin-Weber, J. Filser, A. Heimers, B. Ondruschka, J. Ranke, M. Schaefer, H. Schröder, A. Stark, P. Stepnowski, F. Stock, R. Störmann, S. Stolte, U. Welz-Biermann, S. Ziegert and J. Thöming, Green Chem., 2005, 7, 362–372 RSC.
- R. S. Boethling, E. Sommer and D. DiFiore, Chem. Rev., 2007, 107, 2207–2227 CrossRef CAS PubMed.
- J. Ranke, S. Stolte, R. Störmann, J. Arning and B. Jastorff, Chem. Rev., 2007, 107, 2183–2206 CrossRef CAS PubMed.
- N. V. Ignat'ev, U. Welz-Biermann, A. Kucheryna, G. Bissky and H. Willner, J. Fluorine Chem., 2005, 126, 1150–1159 CrossRef CAS PubMed.
- S. Stolte, S. Steudte, A. Igartua and P. Stepnowski, Curr. Org. Chem., 2011, 15, 1946–1973 CrossRef CAS.
- T. P. T. Pham, C.-W. Cho and Y.-S. Yun, Water Res., 2010, 44, 352–372 CrossRef CAS PubMed.
- D. Coleman and N. Gathergood, Chem. Soc. Rev., 2010, 39, 600–637 RSC.
- K. M. Docherty, J. K. Dixon and C. F. Kulpa Jr., Biodegradation, 2007, 18, 481–493 CrossRef CAS PubMed.
- S. Stolte, S. Abdulkarim, J. Arning, A.-K. Blomeyer-Nienstedt, U. Bottin-Weber, M. Matzke, J. Ranke, B. Jastorff and J. Thöming, Green Chem., 2008, 10, 214–224 RSC.
- J. R. Harjani, R. D. Singer, M. T. Garcia and P. J. Scammells, Green Chem., 2009, 11, 83–90 RSC.
- T. P. T. Pham, C.-W. Cho, J. Min and Y.-S. Yun, J. Biosci. Bioeng., 2008, 105, 425–428 CrossRef CAS PubMed.
- R. P. Swatloski, J. D. Holbrey and R. D. Rogers, Green Chem., 2003, 5, 361–363 RSC.
- J. R. Harjani, J. Farrell, M. T. Garcia, R. D. Singer and P. J. Scammells, Green Chem., 2009, 11, 821–829 RSC.
- M. T. Garcia, N. Gathergood and P. J. Scammells, Green Chem., 2005, 7, 9–14 RSC.
- S. Steudte, J. Neumann, U. Bottin-Weber, M. Diedenhofen, J. Arning, P. Stepnowski and S. Stolte, Green Chem., 2012, 14, 2474–2483 RSC.
- J. Neumann, C.-W. Cho, S. Steudte, J. Köser, M. Uerdingen, J. Thöming and S. Stolte, Green Chem., 2012, 14, 410–418 RSC.
- C. Zhang, H. Wang, S. V. Malhotra, C. J. Dodge and A. J. Francis, Green Chem., 2010, 12, 851–858 RSC.
- S. Kumar, W. Ruth, B. Sprenger and U. Kragl, Chem. Today, 2006, 24, 70–72 Search PubMed.
-
J. B. Wesnigk, M. Keskin, W. Jonas, K. Figge and G. Rheinheimer, in The Handbook of Environmental Chemistry Vol. 2, Part K: Biodegradation
and Persistence, ed. B. Beek, Springer-Verlag, Berlin, Heidelberg, 2001, vol. 2, pp. 253–290 Search PubMed.
- C. Pretti, M. Renzi, S. E. Focardi, A. Giovani, G. Monni, B. Melai, S. Rajamani and C. Chiappe, Ecotoxicol. Environ. Saf., 2011, 74, 748–753 CrossRef CAS PubMed.
- F. Atefi, M. T. Garcia, R. D. Singer and P. J. Scammells, Green Chem., 2009, 11, 1595–1604 RSC.
- A. S. Wells and V. T. Coombe, Org. Process Res. Dev., 2006, 10, 794–798 CrossRef CAS.
-
Organisation for Economic Co-operation and Development (OECD), OECD guideline for testing of chemicals 301 - Ready Biodegradability, 1992 Search PubMed.
- M. Markiewicz, M. Piszora, N. Caicedo, C. Jungnickel and S. Stolte, Water Res., 2013, 47, 2921–2928 CrossRef CAS PubMed.
- S. Stolte, S. Steudte, A. Markowska, J. Arning, J. Neumann and P. Stepnowski, Anal. Methods, 2011, 3, 919–926 RSC.
- M. Markiewicz, C. Jungnickel, A. Markowska, U. Szczepaniak, M. Paszkiewicz and J. Hupka, Molecules, 2009, 14, 4396–4405 CrossRef CAS PubMed.
- B. Peric, J. Sierra, E. Martí, R. Cruañas, M. A. Garau, J. Arning, U. Bottin-Weber and S. Stolte, J. Hazard. Mater., 2013, 261, 99–105 CrossRef CAS PubMed.
- A. Banerjee, R. Sharma and U. C. Banerjee, Appl. Microbiol. Biotechnol., 2002, 60, 33–44 CrossRef CAS PubMed.
- M. Kobayashi and S. Shimizu, Nat. Biotechnol., 1998, 16, 733–736 CrossRef CAS PubMed.
- P. K. Mascharak, Coord. Chem. Rev., 2002, 225, 201–214 CrossRef CAS.
- R. Singh, R. Sharma, N. Tewari and D. S. Rawat, Chem. Biodiversity, 2006, 3, 1279–1287 CAS.
- J. Neumann, M. Pawlik, D. Bryniok, J. Thöming and S. Stolte, Environ. Sci. Pollut. Res. Int., 2013 DOI:10.1007/s11356-013-2341-2.
- G. Vázquez-Rodríguez, R. I. Beltrán-Hernández, C. Coronel-Olivares and J.-L. Rols, Anal. Bioanal. Chem., 2011, 401, 1127–1137 CrossRef PubMed.
- A. K. Goodhead, I. M. Head, J. R. Snape and R. J. Davenport, Environ. Sci. Pollut. Res. Int., 2013 DOI:10.1007/s11356-013-2064-4.
- K. M. Docherty, M. V. Joyce, K. J. Kulacki and C. F. Kulpa, Green Chem., 2010, 12, 701–712 RSC.
- T. P. T. Pham, C.-W. Cho, C.-O. Jeon, Y.-J. Chung, M.-W. Lee and Y.-S. Yun, Environ. Sci. Technol., 2009, 43, 516–521 CrossRef CAS.
- L. G. Whyte, L. Bourbonnière and C. W. Greer, Appl. Environ. Microbiol., 1997, 63 Search PubMed.
- B. W. Brandt, F. D. L. Kelpin, I. M. M. van Leeuwen and S. A. L. M. Kooijman, Water Res., 2004, 38, 1003–1013 CrossRef CAS PubMed.
- M. Devers, N. Rouard and F. Martin-Laurent, Environ. Microbiol., 2008, 10, 676–684 CrossRef CAS PubMed.
- M. Markiewicz, S. Stolte, Z. Lustig, J. Łuczak, M. Skup, J. Hupka and C. Jungnickel, J. Hazard. Mater., 2011, 195, 378–382 CrossRef CAS PubMed.
- J. F. Fernandez, J. Neumann and J. Thöming, Curr. Org.
Chem., 2011, 15, 1992–2014 CrossRef CAS.
- P. Stepnowski and A. Zaleska, J. Photochem. Photobiol., A, 2005, 170, 45–50 CrossRef CAS PubMed.
- N. Gathergood, M. T. Garcia and P. J. Scammells, Green Chem., 2004, 6, 166–175 RSC.
- A. Romero, A. Santos, J. Tojo and A. Rodríguez, J. Hazard. Mater., 2008, 151, 268–273 CrossRef CAS PubMed.
- OECD Guideline for Testing of Chemicals No. 302B - Zahn-Wellens/EMPA Test, 1992.
Footnote |
† Electronic supplementary information (ESI) available. See DOI: 10.1039/c3gc41997e |
|
This journal is © The Royal Society of Chemistry 2014 |
Click here to see how this site uses Cookies. View our privacy policy here.