DOI:
10.1039/C3FO60445D
(Paper)
Food Funct., 2014,
5, 149-157
Ameliorative effect of Pracparatum mungo extract on high cholesterol diets in hamsters
Received
25th September 2013
, Accepted 13th November 2013
First published on 13th November 2013
Abstract
This study was designed to test the lipid-lowering and antioxidative activities of Pracparatum mungo extract (PME), in comparison to its components berberine and glycyrrhizin in cholesterol-fed hamsters. The PME and berberine significantly lowered the atherogenic index compared to glycyrrhizin and the control (P < 0.05). The hepatic HMG-CoA reductase activity was significantly lower in the PME and berberine groups than in the glycyrrhizin group (P < 0.05), while the hepatic ACAT activity was significantly decreased by all treatments with respect to the high cholesterol fed group (P < 0.05). The overall potential of the antioxidant system was significantly enhanced by the PME, berberine and glycyrrhizin supplements as the plasma and hepatic TBARS levels were lowered while the hepatic superoxide dismutase activity and glutathione levels, HMG-CoA reductase, LDL receptor, PPAR, SREBP-2 and CYP7A1 mRNA expressions were increased by the treatments of PME and berberine in comparison with the high cholesterol fed hamsters (P < 0.05). Collectively, these results suggest that the supplementation of PME, berberine and glycyrrhizin increased antioxidant activity in hamsters. Furthermore, we observed that PME and berberine groups promoted the excretion of neutral and acidic sterols (P < 0.05), that could contribute to explain the lower plasma and hepatic cholesterol levels found in the treated animals.
1. Introduction
Cholesterol conversion into 7α-hydroxylated bile acids is the principle pathway of cholesterol catabolism and secretion from the body. Cholesterol 7α-hydroxylase (CYP7A1) initiates and is the rate-determining enzyme of the “classic pathway” of bile acid synthesis.1,2 Complete deficiency of CYP7A1 activity results in significant elevation of total and low-density lipoprotein (LDL) cholesterol (T-C and LDL-C, respectively) and substantial accumulation of cholesterol in the liver.3 Increased levels of cholesterol and triglycerides are known to be risk factors for developing coronary artery diseases. Lipid-lowering agents that inhibit HMG coenzyme A reductase are now prominent among the drugs of choice for treating hypercholesterolemia. As we know, serum cholesterol homeostasis is regulated via cholesterol biosynthesis, catabolism and output, and serum cholesterol clearance. 3-Hydroxy-3-methylglutaryl-CoA reductase (HMG-CoA reductase) is the control point for cholesterol biosynthesis4 while the LDL receptor also plays an important role in clearance of cholesterol from blood.5 Hence, the relationship of HMG-CoA reductase, CYP7A1 and LDL receptor gene expressions are often discussed in hypolipidemic studies.
Sterol responsive element binding proteins (SREBPs) serve as master regulators of cellular lipid synthesis. For instance, SREBP-1c independently regulated fatty acid synthesis, whereas SREBP-2 regulates cholesterol synthesis.6,7 The proteolytic activation of SREBPs and the consequent production of cholesterol and fatty acids is inhibited by elevated intracellular free cholesterol and enhanced by a reduced intracellular free cholesterol concentration.8,9 Peroxisome proliferator-activated receptor (PPAR)-α is highly expressed in the liver, muscle, kidney and heart where it regulates the expression of genes involved in the uptake, binding, transport, cellular retention, and catabolism of fatty acids.10–12 A PPAR-α deficiency has been shown to accelerate dyslipidemia, glomerular matrix expansion, inflammatory cell infiltration and proteinuria in animal models with diabetic nephropathy.13,14 Many foods have one or more substances with physiological and biochemical functions which benefit human health. Pracparatum mungo is a traditional and functional food in Chinese medicine that is made from mung bean (Phaseolus mungo L.) through natural fermentation, soaking in an aqueous Chinese herbal medicine mixture, and then drying at room temperature. The P. mungo extract (PME) contains berberine and glycyrrhizin as major compounds, the amounts of berberine and glycyrrhizin in the PME were calculated to be 23.99 and 80.01 μg g−1 extract,15 respectively. Berberine is a major form of an isoquinoline alkaloid derived from medicinal herbs such as Hydrastis canadensis (goldenseal), Cortex phellodendri (huangbai), and Rhizomacoptidis (huanglian), showing antibacterial and antipyretic activities.16 A recent study has demonstrated the beneficial effect of berberine on suppressed proinflammatory responses through AMP-activated protein kinase (AMPK) activation in macrophages.17 Glycyrrhizin, a triterpene glycoside and a conjugative compound of enoxolone and glucuronic acid as a major active constituent of licorice (Glycyrrhiza glabra) root, has various pharmacological effects including anti-inflammatory, anti-viral, antioxidative, anti-liver cancer, immunomodulatory and hepatoprotective activities.18,19 However, except for the research by Kuo et al.15 that found that PME has a protective effect against acute hepatotoxicity in rat, there exists no research reported on P. mungo. Hence, the present study was undertaken to investigate the lipid-lowering and antioxidative activities of glycyrrhizin and berberine in comparison to PME, in male Golden Syrian hamsters fed a high cholesterol diet in a hamster model.
2. Methods and materials
2.1. Preparation of P. mungo
P. mungo was prepared by a liquid fermentation system. Mung beans were used as raw material and inoculated with Bacillus subtilis var. natto and Rhizopus microsporus var. oligosporus with an incubation temperature at 25 ± 2 °C (Hsien Lu Pharmaceutical Co., Ltd., Tainan, Taiwan). Fermented mung beans (5 kg) were dried at room temperature, washed clean and soaked in boiled-herbal medicine (10 L) mixture including Coptis chinensis FRANCH, Glycyrrhiza uralensis Fisch, Taraxacum monogolicum Hand.-Mazz, Imperata cylindrical (L.), Smilax glabra Roxb., Gardenia jasminoides Ellis, Poria cocus (SchW.) Wolff, Cornus officinalis Sieb.et Zucc., Fritillaria cirrhosa D. Don and Rehmanni alutinosa (Gaertner) Libosch for a month, then dried as powders. The P. mungo were dissolved in normal saline prior to oral administration.15
2.2. Materials
Glycyrrhizin and berberine standards were purchased from Aldrich-Sigma Chemical Co. (St. Louis, MO, USA).
2.3. Animals
Male Golden Syrian hamsters, weighing 260–270 g, were purchased from the National Laboratory Animal Center. They were kept in an air-conditioned room (23 ± 1 °C, 50–60% humidity) and given light for 12 h per day (7 AM to 7 PM). Our Institutional Animal Care and Use Committee approved the protocols for the animal study, and the animals were cared for in accordance with the institutional ethical guidelines. After acclimatizing for 2 weeks with a commercial non-purified diet (rodent Laboratory Chow 5001, Purina Co., USA), 24 hamsters were divided into four groups of six hamsters each. The compositions of the control and treatment diets are listed in Table 1. In previous studies, a hypercholesterol diet induced an increase in cholesterol, TG and oxidative stress.20 The diets were synthesized as described previously by the American Institute of Nutrition (AIN)21 and included: control diet, PME diet (15% PME at doses of 250.05 mg kg−1), glycyrrhizin diet (15% glycyrrhizin at doses of 250.05 mg kg−1) and berberine diet (15% berberine at doses of 250.05 mg kg−1) for 8 weeks.
Table 1 Composition of the experimental diets for animal diet
Ingredient |
Diets |
Control (%) |
PME (%) |
Berberine (%) |
Glycyrrhizin (%) |
Minerals per 100 g diet: NaCl 7.4 g, K2C6H5O7 H2O 22 g, K2SO4 5.2 g, CaHPO4 50 g, MgO 2.4 g, FeC6H5O7·5H2O 0.6 g, MnCO3 0.35 g, CuCO3 30 mg, CrK(SO4)2·12H2O 55 mg, CoCl2·6H2O 10 mg, KI 1 mg, ZnCO3 160 mg.
Vitamin per 100 g diet: thiamine 100 mg, riboflavin 150 mg, pyridoxine HCl 100 mg, nicotinamide 1000 mg, D-panthenate 500 mg, folic acid 50 mg, vitamin B12 0.1 mg, vitamin A 2.5 × 105 IU, vitamin E 100 mg, calciferol 2 × 104 IU, vitamin C 3.7 × 103 mg.
|
Casein |
20 |
20 |
20 |
20 |
Methionine |
0.3 |
0.3 |
0.3 |
0.3 |
Cellulose |
5 |
5 |
5 |
5 |
Corn oil |
2 |
2 |
2 |
2 |
Cholesterol |
2 |
2 |
2 |
2 |
PME |
0 |
15 |
0 |
0 |
Berberine |
0 |
0 |
15 |
0 |
Glycyrrhizin |
0 |
0 |
0 |
15 |
Choline |
0.2 |
0.2 |
0.2 |
0.2 |
Mineral mixa |
3.5 |
3.5 |
3.5 |
3.5 |
Vitamin mixb |
1 |
1 |
1 |
1 |
Corn starch |
31 |
16 |
16 |
16 |
Sucrose |
35 |
35 |
35 |
35 |
Hamsters were fasted for 10 h before the experiment, blood was obtained by tail vein puncture and the hamsters were weighed and euthanized (with diethyl ether) on the 8th week. The cholesterol, HDL-cholesterol and triglyceride (TG) levels in the plasma were determined by enzymatic assay using commercially available enzymatic kits with Selectra Analyser (Merck Co. Ltd, Germany). Livers and kidneys were quickly excised and weighed. The livers were stored at −40 °C for enzymatic glutathione (GSH) and TBARS determinations as specified below.
2.4. Hepatic HMG-CoA reductase and ACAT activities
The liver cell suspension was homogenized in 0.25 M sucrose solution and was centrifuged to obtain a microsome fraction. Microsomal HMG-CoA reductase activity was measured using [14C] HMG-CoA and an NADPH regenerating system as previously described.22 Each reaction mixture contained 0.1 mg of protein, as determined by a biuret protein assay, and was incubated for 10 min. The product [14C] mevalonate was converted to the lactone and isolated by thin layer chromatography. Enzyme activity was expressed as nmol min−1 mg−1 of microsomal protein.22 The activity of ACAT was determined by the formation of cholesteryl [14C] oleate from [14C] oleoyl-CoA and endogenous cholesterol as previously described.23 The radioactivity was expressed as PSL (photo stimulated luminescense). Cholesterol in the liver microsomal fractions was extracted with a chloroform–methanol (2
:
1 v/v) mixture and measured enzymatically.24
2.5. Fecal sterols
Animals were placed in individual cages in order to collect stools from each of them on the 8th week, analysis of fecal neutral sterols was carried out in a Hewlett Packard Gas Chromatograph (GC) Model 873 equipped with a hydrogen flame ionization detector and a dual pen recorder. A 6 ft long (3 mm i.d.) U-shaped column was silanized and packed with 3% SE-30 on 100–120 mesh Supelcoport. Helium was used as the carrier gas at a flow rate of 40–50 mL min−1 and an inlet pressure of 40 psi. Operating temperatures for the column, inlet and detector were 260, 280 and 280 °C, respectively. Daily neutral sterol excretion was calculated based on the amount of cholesterol, coprostanol and coprostanone in each sample. The fecal acid sterols were determined using a Hitachi Liquid Chromatograph (Hitachi, Ltd, Tokyo) consisting of a Model L-6200 pump, a Rheodyne Model 7125 syringe loading sample injector, a Model L-4000 UV-Vis detector set at 210 nm and a Model D-2500 Chromato-integrator. A Lichrospher 100 RP-18 reverse-phase column (5 μm, 25 = 0.3 cm2 i.d., E. Merck) was used for separation. A mixed solvent of 0.3% ammonium carbonate solution–acetonitrile was used as the mobile phase. The system of the mobile phase was as follows: the ratio of both solutions from 73
:
27 for 10 min, 68
:
32 for 10 min and then to 50
:
50 for 10 min. The flow rate was 0.8 mL min.25 The daily acid sterol excretion was calculated based on the amount of cholic acid, deoxycholic acid, lithocholic acid and glycocholic acid in each sample.
2.6. Antioxidant activities
Appropriate liver tissues were dissected, weighed, immersed in liquid N2 generally within 60 s from death, and kept frozen at −70 °C. Prior to enzyme determinations, thawed tissue samples were homogenized in 20 volumes of ice cold 50 mM phosphate buffer (pH 7.4), centrifuged at 3200g for 20 min at 5 °C. The supernatant fraction was used for below antioxidant enzyme determinations.
2.6.1. CAT activity.
The mitochondria pellet (centrifuged at 9400g for 10 min) was dissolved in 1.0 mL of a 0.25 M sucrose buffer. 10 μL of the mitochondria solution was added to a cuvette containing 2.89 mL of a 50 mM potassium phosphate buffer (pH 7.4), then the reaction was initiated by adding 0.1 mL of 30 mM H2O2 to make a final volume of 3.0 mL at 25 °C. The decomposition rate of H2O2 was measured at 240 nm for 5 min on a spectrophotometer. A molar extinction coefficient of 0.041 mM−1 cm−1 was used to determine the CAT activity. The activity was defined as the μmole H2O2 decrease per mg protein per min.26
2.6.2. SOD activity assays.
100 μL of the cytosol supernatant was mixed with 1.5 mL of a Tris–EDTA–HCl buffer (pH 8.5) and 100 μL of 15 mM pyrogallol, and then incubated at 25 °C for 10 min. The reaction was terminated by adding 50 μL of 1 N HCl, the activity was measured at 440 nm. One unit was determined as the amount of enzyme that inhibited the oxidation of pyrogallol by 50%. The activity was expressed as units per mg protein.27
2.6.3. Measurement of GSH levels.
Glutathione levels were measured using the glutathione assay kit (Calbiochem, San Diego, CA). An equal volume of ice cold 10% metaphosphoric acid was added to the liver preparations. Supernatants were collected after centrifugation and analysed for total GSH as per the manufacturer's instructions. Total GSH in the samples were normalised with protein.28
2.7. TBARS concentration
The lipid peroxidation level in the heart and erythrocyte homogenates was estimated by measuring thiobarbituric acid reactive substances (TBARS) and was expressed in terms of malondialdehyde (MDA) content, which is the end product of lipid peroxidation. In brief, 125 μL of the supernatants were homogenized by sonication with 50 μL of TBS, 125 μL of TCA–BHT in order to precipitate proteins and centrifuged (1000g, 10 min, 4 °C). 200 μL of the obtained supernatant was mixed with 40 μL of HCl (0.6 M) and 160 μL of TBA dissolved in Tris, and the mixture was heated at 80 °C for 10 min. The absorbance of the resultant supernatant was read at 530 nm. The amount of TBARS was calculated by using an extinction coefficient of 156 × 105 mM−1 cm−1.
2.8. Atherogenic index
The atherogenic index (AI) was calculated using the following formula: AI = (total cholesterol − HDL-cholesterol)/HDL-cholesterol.29
2.9. Protein and Western blot analysis
The hepatic protein was extracted using commercial kits. Protein levels in the supernatants were determined using the BCA assay kit (Pierce Biotechnology, Inc., Rockford, IL, USA). Samples (60 μg each) were separated by denaturing SDS–PAGE and transferred to a PVDF membrane (Roche Diagnostics Corporation, Indianapolis, IN, USA) by electrophoretic transfer (Bio-Rad Laboratories, Inc., USA). The membrane was preblocked with 5% non-fat milk and 0.1% Tween-20 in Tris-buffered saline (TBST), incubated overnight with the primary antibody low-density lipoprotein receptor (LDL-R), CYP7A1, PPAR-α, SREBP-2 (Santa Cruz Biotechnology, CA, USA) and 3-hydroxy-3-methylglutaryl-CoA reductase (HMG-CoA reductase) (Upstate USA, Lake Placid, NY, USA). Each membrane was washed three times for 15 min and incubated with the secondary horseradish peroxidase-linked antibodies (Santa Cruz Biotechnology, CA, USA and Upstate USA, Lake Placid, NY, USA, respectively). Quantitative analysis of detected bands was performed with the Scion Image analysis software (Scion Co., Frederick, MD, USA). To prove equal loading, the blots of HMG-CoA reductase, CYP7A1, PPAR-α, SREBP-2 and LDL-R were analyzed for β-actin expression using an anti-β-actin antibody (Cell Signaling Technology, Beverly, MA, USA). Each density was normalized using each corresponding β-actin density as an internal control and averaged from three samples.
2.10. Statistical analysis
Statistical analysis for differences among experimental groups was performed by the one-way analysis of variance procedure and Duncan's new multiple range tests.30 All statistical analyses were performed using the statistical software SPSS 11.0 (SPSS Ltd., Surrey, UK). A P value <0.05 was considered statistically significant.
3. Results
3.1. Body weight, liver and kidney weight to body weight ratios, food intake
The supplementation of PME and berberine significantly lowered the body weight compared to the glycyrrhizin and control group (P < 0.05), there was no significant difference in the liver and kidney to body weight ratios among the various groups and food intake (P > 0.05) (Table 2).
Table 2 Effect of PME, berberine and glycyrrhizin supplementation on food intake, body weight, liver and kidney to body weight ratios in high cholesterol diet-fed hamstersa
Groups |
Control |
PME |
Berberine |
Glycyrrhizin |
mean ± S.E.; a–c, means in same row not sharing a common superscript are significantly different among groups (P < 0.05); NS, not significantly different (P > 0.05) among groups.
|
Food intake (g per day) |
26.23 ± 0.25NS |
26.35 ± 0.21 |
26.18 ± 0.25 |
26.31 ± 0.33 |
Body weight (g) |
373 ± 13c |
336 ± 15a |
339 ± 13a |
351 ± 13b |
Liver weight/body weight ratio |
2.33 ± 0.13NS |
2.38 ± 0.11 |
2.27 ± 0.12 |
2.26 ± 0.175 |
Kidney weight/body weight ratio |
2.62 ± 0.16NS |
2.66 ± 0.17 |
2.66 ± 0.15 |
2.63 ± 0.18 |
3.2. Plasma and hepatic lipids
The supplementation of PME and berberine significantly lowered both the plasma cholesterol and triglyceride concentrations compared to the glycyrrhizin and control groups (P < 0.05) (Table 3). The HDL-cholesterol level and HDL-C/total-C ratios in the plasma were significantly increased in the three supplement groups compared to the control group and was significantly higher in the PME and berberine supplement groups (P < 0.05) than in the groups treated with glycyrrhizin and the control. In addition, the atherogenic index was significantly lower in the PME and berberine group than in the glycyrrhizin and control group (P < 0.05). In contrast to the plasma lipid (TG) levels of PME, the glycyrrhizin and berberine supplementation groups were shown to be significantly different and the plasma lipid levels of the PME and berberine supplementation groups were significantly lower compared to the other two supplementation groups (P < 0.05) (Table 3). However, the hepatic lipid levels of the PME and berberine supplementation groups were significantly lowered compared to the control and glycyrrhizin group (P < 0.05) (Table 3).
Table 3 Effect of PME, berberine and glycyrrhizin supplementation on plasma and hepatic lipids in high cholesterol diet-fed hamstersa
Groups |
Control |
PME |
Berberine |
Glycyrrhizin |
Mean ± S.E; a–c, means in same row not sharing a common superscript are significantly different among groups (P < 0.05); atherogenic index: (total cholesterol − HDL-cholesterol)/HDL-cholesterol.
|
Plasma
|
Total-cholesterol (mmol L−1) |
4.32 ± 0.35b |
2.77 ± 0.32a |
2.75 ± 0.35a |
4.28 ± 0.28b |
HDL-cholesterol (mmol L−1) |
1.59 ± 0.18a |
2.66 ± 0.13b |
2.53 ± 0.13b |
1.62 ± 0.19a |
HDL-C/Total-C (%) |
36.3 ± 2.5a |
49.8 ± 2.2b |
48.6 ± 2.5b |
35.8 ± 1.8a |
TG (mmol L−1) |
1.62 ± 0.11b |
1.36 ± 0.07a |
1.35 ± 0.06a |
1.57 ± 0.06b |
Atherogenic index |
1.36 ± 0.15b |
0.73 ± 0.06a |
0.76 ± 0.05a |
1.35 ± 0.07b |
|
Liver
|
Cholesterol (mmol g−1) |
0.98 ± 0.06b |
0.37 ± 0.03a |
0.35 ± 0.08a |
0.96 ± 0.06b |
TG (mmol g−1) |
0.38 ± 0.035b |
0.22 ± 0.033a |
0.23 ± 0.032a |
0.37 ± 0.038b |
3.3. Hepatic HMG-CoA reductase and ACAT activities
The hepatic HMG-CoA reductase activity was significantly decreased by the PME and berberine supplement compared to the glycyrrhizin supplement and control group (P < 0.05) (Fig. 1). However, the hepatic ACAT activity was slightly but significantly decreased by all treatments. No differences among the treatments were observed.
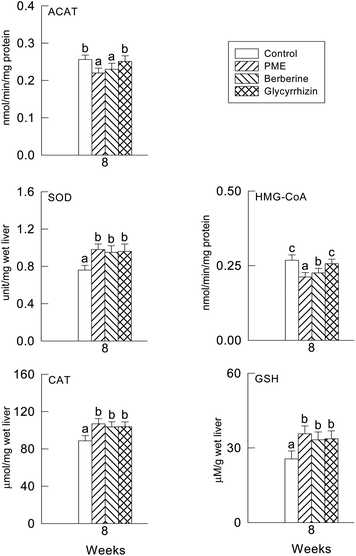 |
| Fig. 1 Effect of PME, berberine and glycyrrhizin supplementation on hepatic HMG-CoA reductase, ACAT, SOD and CAT activities, and GSH levels in high cholesterol diet-fed hamsters. (a–c): values in the same week with different superscripts are significantly different (P < 0.05). | |
3.4. Hepatic antioxidant enzyme activities and plasma and hepatic TBARS levels
The activities of CAT, SOD and the GSH levels were not significantly different in the three supplement groups (P > 0.05) (Fig. 1), though there were significant differences between the supplement groups and the control group (P < 0.05). In fact, the levels of the plasma and hepatic TBARS were significantly lower in the PME treated group (P < 0.05), it seems in Fig. 2 that the TBARS ratio of the three groups to the control group is higher in the plasma than in the liver, therefore the PME, berberine and glycyrrhizin supplements were more effective in lowering the plasma TBARS level than in the liver.
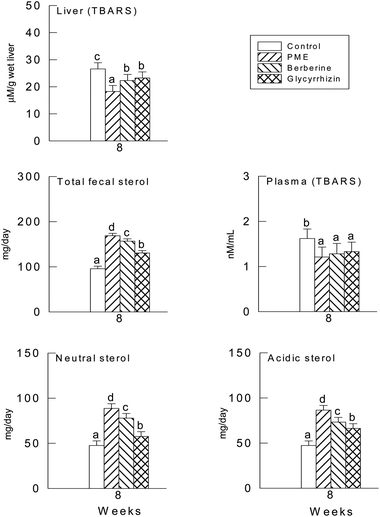 |
| Fig. 2 Effect of PME, berberine and glycyrrhizin supplementation on the plasma and hepatic TBARS, and fecal sterol concentration in high cholesterol diet-fed hamsters. (a–c): values in the same week with different superscripts are significantly different (P < 0.05). | |
3.5. Fecal sterols
The daily excretion of fecal sterols is shown in Fig. 2. When compared with the control group, the PME, berberine and glycyrrhizin supplements induced a significant increase in the excretion of neutral, acidic and total fecal sterols (P < 0.05).
3.6. Hepatic mRNAs
The relative quantities of mRNA were determined by the southern hybridization of PCR-amplified PPARα cDNA, LDL-R cDNA, SREBP-2 cDNA, HMG-CoA reductase cDNA and CYP7A1 cDNA in the hamster liver. The values of PPARα, LDL-R, SREBP-2, HMG-CoA reductase, CYP7A1 and SREBP-2 mRNAs were normalized to the value of GAPDH mRNA. Values from the liver samples of the hamsters fed with PME and berberine were expressed relative to the average values of the control group, which were normalized to 100. The PPARα, LDL-R, SREBP-2, HMG-CoA reductase, CYP7A1 and SREBP-2 mRNA levels were higher in both the PME and berberine groups compared with the glycyrrhizin group (Fig. 3).
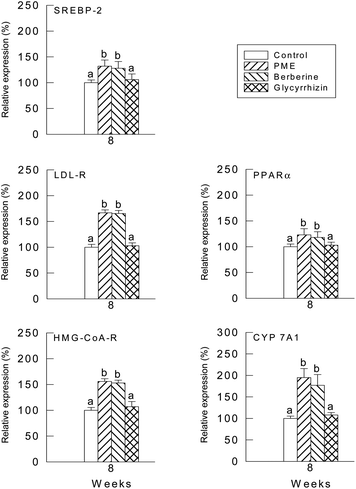 |
| Fig. 3 Effect of PME, berberine and glycyrrhizin supplementation on HMG-CoA reductase, LDL receptor, PPAR-α, SREBP-2 and CYP7A1 mRNA expressions in high cholesterol diet-fed hamsters. (a–d): values in the same week with different superscripts are significantly different (P < 0.05). | |
4. Discussion
The present study investigated the effects of dietary PME, berberine and glycyrrhizin to determine their possible role in a high-cholesterol fed state. The results suggest that the plasma lipid-lowering and antioxidative effects of PME, berberine and glycyrrhizin supplements were exhibited in the high cholesterol diet-fed hamsters.
The liver TG level was significantly lower for the PME supplement group than the control group. The liver is the major site for the synthesis and net excretion of cholesterol, and hepatic HMG-CoA reductase activity is normally inhibited under high-cholesterol feeding conditions by means of a negative feedback control.31 In the current study, the plasma cholesterol concentration was lowered by PME and berberine supplements to the same extent, although PME exhibited a more potent biological effect on lowering hepatic HMG-CoA reductase activity than berberine. The hepatic TG level was significantly lower in the PME supplement group than in the control group. The reduced ACAT activity in these groups may have led to less cholesteryl ester being available for VLDL packing, thereby resulting in a reduction of VLDL secretion from the liver.32
According to the results of the current study, although PME and berberine inhibited the hepatic HMG-CoA reductase activity, it would appear that the decrease in the plasma cholesterol concentration, resulting from the PME, berberine and glycyrrhizin supplements, may have been due to the increased fecal sterol. The action of berberine in the cholesterol-lowering mechanism appeared to be similar to that of PME, except with regard to the HMG-CoA reductase activity, which is known to be down-regulated by the body cholesterol and can be inhibited by HMG-CoA reductase inhibitors. Dietary PME, glycyrrhizin and berberine did have a significant effect on the two hepatic antioxidant systems, SOD and GSH, along with the plasma and hepatic TBARS levels. SOD converts superoxide radicals into hydrogen peroxide, which is then converted to water by both CAT and GSH. As a result, CAT, SOD and GSH can prevent damage by detoxifying the reactive oxygen species. The plasma and hepatic TBARS levels in the PME, glycyrrhizin and berberine treated animals showed a significant reduction, thereby indicating a decreased rate of lipid peroxidation. A concomitant increase in the antiperoxidative activities, namely SOD and GSH, was observed in the PME, glycyrrhizin and berberine groups, and SOD and GSH play important roles in the scavenging of toxic intermediates of incomplete oxidation in the body. The significant elevation of SOD activity also suggests that the free-radical scavenging activity of this enzyme is only effective when it is accompanied by an increase in the activity of catalase and/or GSH. This is because SOD generates hydrogen peroxide as a metabolite, which is more toxic than oxygen radicals in cells and needs to be scavenged by catalase or GSH.33 As such, a concomitant increase in catalase and/or GSH activity is essential if a beneficial effect from the high SOD activity is to be expected. A cholesterol diet may induce lipid peroxidation and reduce diet palatability in hamsters. TBARS is an end-product of lipid peroxidation. The level of TBARS in the liver was increased when the hamsters were fed cholesterol. This means that the oxidative stress caused by feeding with cholesterol was due to the induction of the lipid peroxidation of liver cells. The level of TBARS in the liver of hamsters was significantly reduced when the hamsters were fed with the supplement of PME, glycyrrhizin and berberine. This result is the same as that reported previously.34 Therefore, it is reasonable to assume that PME, glycyrrhizin and berberine may act as a good scavenger in reducing the production of lipid peroxidation.35 Meanwhile, the function of GSH to protect biological organisms from xenobiotic injuries is well known.36–38 Lipid peroxidation is a chemical mechanism capable of disrupting the structure and function of the biological membranes that occurs as a result of free radical attack on lipids. When reactive oxygen species (ROS) begin to accumulate, hepatic cells exhibit a defensive mechanism by various antioxidant activities. The main detoxifying systems for peroxides are catalase and GSH.39 Catalase is an antioxidant enzyme, which destroys H2O2 that can form a highly reactive hydroxyl radical in the presence of iron as a catalyst.40 By participating in the glutathione redox cycle, GSH together with GSH-Px converts H2O2 and lipid peroxides to non-toxic products. Reduced activity of one or more antioxidant systems due to the direct toxic effect of cholesterol leads to increased lipid peroxidation, oxidative stress, and hepatotoxicity, a cholesterol depleted GSH reservoir and reduced catalase and GSH-Px (glutathione peroxidase) activities. These results are in harmony with other investigations.41 For example, cholesterol induced hepatotoxicity was exacerbated by GSH depletion, the depletion of the GSH reservoir can account for the inhibition of GSH-Px activity. In addition, high levels of peroxides may explain catalase activity inhibition.42 PME, berberine and glycyrrhizin supplementation in our study significantly mitigated cholesterol induced oxidative stress. In addition, PME, berberine and glycyrrhizin inhibited lipid peroxidation, diminished the decrease in catalase and GSH-Px activities, and abrogated GSH depletion induced by cholesterol. PME, berberine and glycyrrhizin has been demonstrated to function as a direct antioxidant that scavenges or quenches oxygen free radicals, thus inhibiting lipid peroxidation, and as an indirect antioxidant that prevents the increase in membrane permeability resulting from oxidant injury in many tissues including liver and plasma.43 Moreover, PME, berberine and glycyrrhizin might lessen cholesterol induced oxidative injury may be capable of lowering or slowing down oxidative-stress.44 The effects PME, berberine and glycyrrhizin on gene expressions of cholesterol homeostasis, i.e., PPARα, LDL-R, SREBP-2, HMG-CoA reductase and CYP7A1 in high cholesterol fed hamsters. The LDL receptor plays a central role in the reduction of cholesterol levels and the prevention of coronary artery heart disease.45 HMG-CoA reductase plays an important role in the control of cholesterol biosynthesis.4 The synthesis of bile acids from cholesterol is regulated by the CYP7A1 gene and CYP7A1 is also associated with metabolic disorders of cholesterol and bile acids.46 PPARα, a fatty acid decomposition related gene, plays a role in the clearance of circulating or cellular cholesterol via the regulation of bile acid biosynthesis by increasing CYP7A1 gene expression in the liver, and fatty acid transport and peroxisomal and mitochondrial fatty acid β-oxidation.47,48 Hence, upregulated LDL-receptor gene expression by PME and berberine groups could explain the lower serum TC in high cholesterol fed hamsters. SREBP-2 has been identified as a transcription factor responsible for the transcription activation of HMG-CoA reductase and LDL-R.49,50 In summary, the data presented in this paper show that administration of PME, glycyrrhizin and berberine is most likely the treatments are not toxic and effective way of lowering cholesterol. This study identified a decrease in the plasma lipid and hepatic cholesterol levels, an increase in the fecal sterols and enhanced antioxidative capacity in hamsters supplemented with PME, berberine and glycyrrhizin. Accordingly, these results indicate that PME, berberine and glycyrrhizin supplements all decreased the cholesterol absorption in high cholesterol fed hamsters. Since the cholesterol intake was about the same for all groups, the supplementation of these compounds appeared to promote an increase in the fecal sterol. It is also worth mentioning that the PME and berberine groups exhibited a greater inhibitory effect on hepatic HMG-CoA reductase and increase of HDL than glycyrrhizin. The PME and berberine groups up-regulated PPARα, LDL-R, SREBP-2, HMG-CoA reductase, CYP7A1 and SREBP-2 gene expressions. However, more studies are needed, with various animal models, to explain the lipid-lowering action and decreased absorption of cholesterol of these PME and berberine supplements. Also into why the supplementation of PME, berberine and glycyrrhizin increased antioxidant activity.
Acknowledgements
This study was supported by the Chung Shan Medical University (CSMU-INT-102-12) and Asia University (101-asia-49).
References
- J. Y. L. Chiang, Regulation of bile acid synthesis, Front. Biosci., 1998, 3, D176–D193 CAS.
- Z. R. Vlahcevic, W. M. Pandak and R. T. Stravitz, Regulation of bile acid biosynthesis, Gastroenterol. Clin. North Am., 1999, 28, 1–25 CrossRef CAS.
- M. S. Brown and J. L. Goldstein, How LDL receptors influence cholesterol and atherosclerosis, Sci. Am., 1984, 251, 58–66 CrossRef CAS PubMed.
- D. Kritchevsky, Inhibition of cholesterol synthesis, J. Nutr., 1987, 117, 1330–1334 CAS.
- J. M. Dietschy, Dietary fatty acids and the regulation of plasma low density lipoprotein cholesterol concentrations, J. Nutr., 1998, 128, 444S–448S CAS.
- M. S. Brown and J. L. Goldstein, The SREBP pathway: regulation of cholesterol metabolism by proteolysis of a membrane-bound transcription factor, Cell, 1997, 89, 331–340 CrossRef CAS.
- S. Ishii, K. Iizuka, B. C. Miller and K. Uyeda, Carbohydrate response element binding protein directly promotes lipogenic enzyme gene transcription, Proc. Natl. Acad. Sci. U. S. A., 2004, 101, 15597–15602 CrossRef CAS PubMed.
- W. Wang, R. Sato, M. S. Brown, X. Hua and J. L. Goldstein, SREBP-1, a membrane-bound transcription factor released by sterol-regulated proteolysis, Cell, 1994, 77, 53–62 CrossRef.
- M. S. Brown and J. L. Goldstein, A proteolytic pathway that controls the cholesterol content of membranes cells and blood, Proc. Natl. Acad. Sci. U. S. A., 1999, 96, 11041–11048 CrossRef CAS.
- O. Barbier, P. Torra, Y. Duguay, C. Blanquart, J. C. Fruchart, C. Glineur and B. Staels, Pleiotropic actions of peroxisome proliferator-activated receptors in lipid metabolism and atherosclerosis, Arterioscler., Thromb., Vasc. Biol., 2002, 22, 717–726 CrossRef CAS.
- Y. Guan, Peroxisome proliferator-activated receptor family and its relationship to renal complications of the metabolic syndrome, J. Am. Soc. Nephrol., 2004, 15, 2801–2815 CrossRef CAS PubMed.
- Y. Guan and M. D. Breyer, Peroxisome proliferator-activated receptors (PPARs): novel therapeutic targets in renal disease, Kidney Int., 2001, 60, 14–30 CrossRef CAS PubMed.
- C. W. Park, H. W. Kim, S. H. Ko, H. W. Chung, S. W. Lim, C. W. Yang, Y. S. Chang, A. Sugawara, Y. Guan and M. D. Breyer, Accelerated diabetic nephropathy in mice lacking the peroxisome proliferator-activated receptor alpha, Diabetes, 2006, 55, 885–893 CrossRef CAS PubMed.
- Y. Mori, T. Hirano, M. Nagashima, Y. Shiraishi, T. Fukui and M. Adachi, Decreased peroxisome proliferator-activated receptor alpha gene expression is associated with dyslipidemia in a rat model of chronic renal failure, Metab., Clin. Exp., 2007, 56, 1714–1718 CrossRef CAS PubMed.
- D. H. Kuo, W. H. Kang, P. C. Shieh, F. A. Chen, C. D. Chang, M. L. Tsai, A. C. Cheng, C. T. Ho and M. H. Pan, Protective effect of Pracparatum mungo extract on carbon tetrachloride-induced hepatotoxicity in rats, Food Chem., 2010, 123, 1007–1012 CrossRef CAS PubMed.
- T. Schmeller, B. Latz-Bruning and M. Wink, Biochemical activities of berberine, palmatine and sanguinarine mediating chemical defence against microorganisms and herbivores, Phytochemistry, 1997, 44, 257–266 CrossRef CAS.
- H. W. Jeong, K. C. Hsu, J. W. Lee, M. Ham, J. Y. Huh, H. J. Shin, W. S. Kim and J. B. Kim, Berberine suppresses proinflammatory responses through AMPK activation in macrophages, Am. J. Physiol.: Endocrinol. Metab., 2009, 296, E955–E964 CrossRef CAS PubMed.
- M. Kimura, T. Moro, H. Motegi, H. Maruyama, M. Sekine, H. Okamoto, H. Inoue, T. Sato and M. Ogihara,
In vivo glycyrrhizin accelerates liver regeneration and rapidly lowers serum transaminase activities in 70% partially hepatectomized rats, Eur. J. Pharmacol., 2008, 579, 357–364 CrossRef CAS PubMed.
- X. Y. Wan, M. Luo, X. D. Li and P. He, Hepatoprotective and antihepatocarcinogenic effects of glycyrrhizin and matrine, Chem.-Biol. Interact., 2009, 181, 15–19 CrossRef CAS PubMed.
- C. F. Su, I. M. Liu and J. T. Cheng, Improvement of insulin resistance by Hon-Chi in fructose-rich chow-fed rats, Food Chem., 2007, 104, 45–52 CrossRef CAS PubMed.
- American Institute of Nutrition (AIN), Report of the American Institute ad hoc committee on standards for nutritional studies, J. Nutr., 1977, 107, 1340 Search PubMed.
- G. C. Ness, L. C. Pendleton and A. S. Pendleton, Loss of NADPH during assays of HMG-CoA reductase: implications and approaches to minimize errors, Lipids, 1987, 22, 409–412 CrossRef CAS.
- A. Knecht, B. Cramer and H. U. Humpf, New Monascus metabolites: structure elucidation and toxicological properties studied with immortalised human kidney epithelial cells, Mol. Nutr. Food Res., 2006, 50, 314–321 CAS.
- T. Sekiya, S. Inoue, T. Shirasaka, C. Miyajima, H. Okushima, K. Suzuki, M. Kawai, M. Mitsika and K. Umezu, Syntheses and pharmacological activities of novel optically active inhibitors of acyl-CoA: cholesterol O-acyltransferase: EAB-309((R)-N-2-(1,3-benzodioxol-4-yl)heptyl-N′-2,6-diisopropylphenylurea) and its enantiomer, Chem. Pharm. Bull., 1994, 42, 586–591 CrossRef CAS.
- Y. H. Yeh and D. F. Hwang, High-performance liquid chromatography determination for bile components in fish, chicken and duck, J. Chromatogr., Biomed. Appl., 2001, 751, 1–8 CrossRef CAS.
-
H. Aebi, Catalase in Method of Enzymatic Analysis, Academic Press, New York, 1974 Search PubMed.
- S. Marklund and G. Marklund, Involvement of the superoxide anion radical in the autoxidation of pyrogallol and a convenient assay for superoxide dismutase, Eur. J. Biochem., 1974, 47, 469–474 CrossRef CAS.
- O. W. Griffith, Determination of glutathione and glutatione disulfide using glutathione reductase and 2-vinylpyridine, Anal. Biochem., 1980, 106, 207–212 CrossRef CAS.
- M. Dobiášová, Atherogenic index of plasma [log(triglycerides/HDL-cholesterol)]: theoretical and practical implications, Clin. Chem., 2004, 50, 1113–1115 Search PubMed.
-
S. C. Puri and K. Mullen, in Applied statistics for food and agricultural scientists, ed. G. K. Hall, Medical Publishers, Boston, 1980 Search PubMed.
- A. Honda, G. Salen, M. Honda, A. K. Batta, G. S. Tint, G. Xu, T. S. Chen, N. Tanaka and S. Shefer, 3-Hydroxy-3-methylglutaryl-coenzyme A reductase activity is inhibited by cholesterol and up-regulated by sitosterol in sitosterolemic fibroblasts, J. Lab. Clin. Med., 2000, 135, 174–179 CrossRef CAS PubMed.
- T. P. Carr, J. S. Parks and L. L. Rudel, Hepatic ACAT activity in African green monkeys in highly correlated to plasma LDL cholesteryl enrichment, and coronary artery atherosclerosis, Arterioscler., Thromb., Vasc. Biol., 1992, 12, 1274–1283 CrossRef CAS.
- E. Pigeolot, P. Corbisier, A. Houbion, D. Lambert, C. Michiels, M. Raes, M. D. Zachary and J. Ramacle, Glutathione peroxidase, superoxide dismutase and catalase inactivation by peroxide and oxygen derived radicals, Mech. Ageing Dev., 1990, 51, 283–297 CrossRef.
- M. Payá, B. Halliwell and J. R. S. Hoult, Peroxyl radical scavenging by a series of coumarins, Free Radical Res., 1992, 17, 293–298 CrossRef.
- I. Stupans, A. Kirlich, K. L. Tuck and P. J. Hayball, Comparison of radical scavenging effect, inhibition of microsomal oxygen free radical generation, and serum lipoprotein oxidation of several natural antioxidants, J. Agric. Food Chem., 2002, 50, 2464–2469 CrossRef CAS PubMed.
- V. L. Tatum, C. Changchit and C. K. Chow, Measurement of malondialdehyde by high performance liquid chromatography with fluorescence detection, Lipids, 1990, 25, 226–229 CrossRef CAS.
- E. Maellaro, A. F. Casini, B. D. Bello and M. Comporti, Lipid peroxidation and antioxidant systems in the liver injury produced by gluthathione depleting agents, Biochem. Pharmacol., 1990, 39, 1513–1521 CrossRef CAS.
- A. Meister and M. E. Anderson, Glutathione, Annu. Rev. Biochem., 1983, 52, 711–760 CrossRef CAS PubMed.
- A. Meister, Selective modification of glutathione metabolism, Science, 1983, 220, 472–478 CAS.
- J. M. C. Gutteridg, Lipid peroxidation and antioxidant as biomarkers of tissue damage, Clin. Chem., 1995, 14, 1819–1828 Search PubMed.
- A. Al Khader, M. Al Sulaiman, P. N. Kishore, C. Morais and M. Tariq, Quinacrine attenuates cyclosporine-induced nephrotoxicity in rats, Transplantation, 1996, 62, 427–435 CrossRef CAS PubMed.
- M. Ghadermarzi and A. A. Moosavi-Movahedi, Determination of the kinetic parameters for the “suicide substrate” inactivation of bovine liver catalase by hydrogen peroxide, J. Enzyme Inhib. Med. Chem., 1996, 10, 167–175 CrossRef CAS.
- E. Mosialou, G. Ekstrom, A. E. P. Adang and R. Morgenstern, Evidence that rat liver microsomal glutathione transferase is responsible for glutathione-dependent protection against lipid peroxidation, Biochem. Pharmacol., 1993, 45, 1645–1651 CrossRef CAS.
- Y. H. Yeh, Y. T. Lee, H. S. Hsieh and D. F. Hwang, Effect of red yeast rice on toxicity of oxidized cholesterol and oxidized fish oil in rats, e-SPEN, Eur. e-J. Clin. Nutr. Metab., 2010, 5, 230–237 CrossRef PubMed.
- D. A. White, A. J. Bennett, M. A. Billett and A. M. Salter, Genetic determinants of plasma lipoprotein levels and their dietary response, Prostaglandins, Leukotrienes Essent. Fatty Acids, 1997, 57, 455–462 CrossRef CAS.
- G. E. Bartley, W. Yokoyama, S. A. Young, W. H. Anderson, S. C. Hung, D. R. Albers, M. L. Langhorst and H. Kim, Hypocholesterolemic effects of hydroxypropyl methylcellulose are mediated by altered gene expression in hepatic bile and cholesterol pathways of male hamsters, J. Nutr., 2010, 140, 1255–1260 CrossRef CAS PubMed.
- M. C. Hunt, Y. Z. Yang, G. Eggertsen, C. M. Carneheim, M. Gafvels, C. Einarsson and S. E. H. Alexson, The peroxisome proliferator-activated receptor alpha regulates bile acid biosynthesis, J. Biol. Chem., 2000, 275, 28947–28953 CrossRef CAS PubMed.
- Y. Ma, L. L. Jiang, R. L. Shi and J. Liu, Effects of activation of liver X receptor and peroxisome proliferator-activated receptor alpha on bile acid synthesis in rats, Zhongguo Yi Xue Ke Xue Yuan Xue Bao, 2007, 29, 384–387 CAS.
- J. D. Horton, J. L. Goldstein and M. S. Brown, SREBPs: Activators of the complete program of cholesterol and fatty acid synthesis in the liver, J. Clin. Invest., 2002, 109, 1125–1131 CAS.
- S. M. Vallet, H. B. Sanchez, J. M. Rosenfeld and T. F. Osborne, A direct role of sterol regulatory element binding protein in activation of 3-hydroxy-3-methylglutaryl coenzyme A reductase gene, J. Biol. Chem., 1966, 271, 12247–12253 Search PubMed.
|
This journal is © The Royal Society of Chemistry 2014 |
Click here to see how this site uses Cookies. View our privacy policy here.