The long-term effects of phage concentration on the inhibition of planktonic bacterial cultures†
Received
13th August 2013
, Accepted 22nd November 2013
First published on 3rd December 2013
Abstract
Since the early 1920s there has been an interest in using bacteriophages (phages) for the control of bacterial pathogens. While there are many factors that have limited the success of phage bio-control, one particular problem is the variability of outcomes between phages and bacteria. Specifically, there is a significant need for a better understanding of how initial phage concentrations affect long-term bacterial inhibition. In work reported herein three phages were isolated for Escherichia coli K12, Pseudomonas aeruginosa PAO1, as well as Bacillus cereus and bio-control experiments were performed with phage concentrations ranging from 105 to 108 plaque forming units per mL over the course of 72 h. For four of the nine phages isolated there was a linear relationship between inhibition and phage concentration, suggesting the effect of phage concentration is important at longer time scales. For three of the isolated phages, phage concentrations had no effect on bacterial inhibition suggesting that even at the lowest concentration the method of action was saturated and lower concentrations might still be effective. Additionally, a cocktail was created and was compared to the previously isolated phages. There was no statistical difference between the cocktail and the best performing phage highlighting the importance of selecting the appropriate phages for treatment. These results suggest that, for certain phages, there is a strong relationship between phage concentration and long-term bacterial growth inhibition and the initial phage concentration is an important indicator of the long-term outcome.
Environmental impact
The overuse of antibiotics has encouraged the emergence of antibiotic resistant pathogens in the environment. These environmental pathogens affect a wide variety of environmental systems and disrupt human health. While traditional disinfectants are effective in controlling pathogen growth, in many environmental cases, a broad disinfectant is undesirable because the indigenous microbiota are affected. Therefore, there is a specific need for alternative green disinfectants that are specific to pathogens of interest for environmental applications. The use of bacteriophages has been proposed as an alternative disinfectant to control environmental pathogens. However, an understanding of bacteriophage and bacteria long-term outcomes is needed to better elucidate the bacteriophage treatment potential. On this note, this paper investigates the long-term treatment efficiencies of bacteriophages.
|
1. Introduction
Our society faces problems with the emergence of antibiotic resistant pathogens in the environment. These issues stem from a variety of sources. For instance, the overuse of antibiotics in agriculture has created oxytetracycline and streptomycin resistant pathogens.1 Animal husbandry practices have been shown to promote the growth of bacteria resistant to the types of antibiotics used in humans.2 Finally, in hospitals, antibiotic resistant bacteria are responsible for many nosocomial infections3,4 and hospital waste effluents have been shown to contribute to the spread of antibiotic resistance in sewer systems and the environment.5 While it is clear that antibiotic resistant pathogens must be controlled, in many cases a strong wide-spectrum biocide cannot be applied because they may drastically harm the indigenous microbiota.
Bacteriophages (phages) have been proposed as a possible treatment method for the bio-control of bacterial contaminants affecting a variety of environmental technologies such as agriculture, animal husbandry, and the bacteria responsible for foaming in wastewater treatment systems.6–9 Phages are viruses that are specific to strains of bacteria and, because they require the host cell's cellular machinery to survive, they are classified as obligate parasites. Phages have potential for the bio-control of environmental pathogens because they are host specific and can target pathogens resistant to traditional disinfectants. Furthermore, because phages are self-replicating, the local phage concentration is sustained over time leading to continued bacterial growth inhibition.10 While there have been many studies demonstrating the effectiveness of phages for controlling pathogens,11–18 the adoption on phage technology is inhibited by the development of phage resistant mutants, biofilms penetration issues, and ultimately the variability of treatment efficiencies.19 Thus, there is a clear need to identify the conditions under which phages could be successfully applied and to develop a framework to model their treatment efficiency.
Many variables have been shown to affect treatment efficacy. For instance, others have shown the importance of the multiplicity of infection (MOI), the ratio of cells to phages, in predicting a successful treatment outcome. Abedon suggested that a MOI of 10, indicating 10 phages to every bacterium with sufficient time for replication is sufficient for treatment.20 In addition, temperate phage life cycles depend on MOI, which drastically affects treatment outcomes.21,22 Growth kinetics of the phage population are also affected by the initial bacterial concentration.20 However, no study has determined how varying the initial phage concentration affects the final treatment efficiency and whether there is a general treatment trend between phages. Finally, there is a general lack of long-term studies. Due to the challenges in measuring phage and cell concentrations over long time periods, most published studies have focused on shorter timer periods (e.g. less than 8 hours) while targeting only one or two different MOIs, limiting the utility of those studies in making predictive models for the application of phage bio-control.
To address the fundamental gaps described above, in the present study, nine phages were isolated, characterized and tested in long-term experiments (72 h) at MOIs ranging from 0.1 to 100. One model Gram-positive bacterium (Bacillus cereus), and two Gram-negative bacteria (Pseudomonas aeruginosa PA01 and Escherichia coli K12) were targeted with initial starting concentrations of 106 CFU mL−1. For each phage, linear models were used to construct relationships between bacterial growth inhibition and phage concentration.
2. Experimental
2.1 Bacterial strains and growth conditions
E. coli K12 (ATCC® 10798), P. aeruginosa PA01 (ATCC® 47085), and B. cereus strain Frankland and Frankland (ATCC® 14579) were grown in Luria-Bertani (LB) medium supplemented with 10 mM MgSO4.
2.2 Phage isolation
Environmental samples used for phage hunting were obtained from activated sludge samples from either the North or South Durham water reclamation plants as well as from soil samples around various locations on Duke University's campus (Durham, NC). Phages were isolated using a modified phage hunting procedure based on Sambrook and Russell's protocol for phage isolation and purification.23 All phages were purified three times to yield unique phages before creation of phage stocks. Briefly, 100 mL of SM buffer (100 nM NaCl, 10 mM MgSO4, 50 mM Tris-CL [pH 7.5], and 0.01% gelatin), 5 mL of LB medium, and 5 mL of late-log bacterial cultures were added to either the activated sludge or soil sample. The resulting mixture was incubated overnight at 37 °C on an orbital shaker at 100 rpm. After 24 h, samples were removed from the incubator and allowed to settle for 30 min. Five mL of supernatant was removed and filtered with a VWR® 25 mm syringe filter and a VWR 0.2 μm polypropylene membrane (Radnor, PA). Two hundred μL of the filtrate was plated with 200 μL of the respective late-log culture with 4 mL of molten soft agar on a LB plate. The plates were inverted after cooling and incubated overnight for 24 h at 37 °C. Single plaques were selected and serially diluted in SM buffer and re-plated using the soft agar method. The isolation procedure was repeated three times to yield pure phages. Phage stocks were created using the soft agar low-titer method and stocks were quantified and diluted to final concentrations of 109 plaque forming units (PFU) per mL.23 Stocks were stored at 4 °C and used within two weeks. Before each experiment stock concentrations were verified by plate counts.
To ensure each phage was unique, a genomic fingerprint was obtained for each phage. To this end, phage nucleic acids were first isolated by standard methods using previously published methods.23 Phage capsids were digested with 1% sodium dodecyl sulfate from Sigma-Aldrich (St. Louis, MO) and 100 μg mL−1 of Proteinase K from New England Biolabs (Ipswich, MA) for 1 h at 56 °C. Nucleic acids were extracted with phenol/chloroform and DNA was precipitated with ethanol and NaCl. A fingerprint was created for each phage using EcoRI, EcoRV, BamHI, XhoI, and BsaI. All restriction enzymes were obtained from New England Biolabs (Ipswich, MA). One thousand ng of phage nucleic acids were digested with 20 units per mL at 37 °C for 1 h. Since some phages did not yield digested fragments with the above restriction enzymes, possibly indicating RNA phages, plaque morphologies were also used in combination with the restriction digests to verify unique phages.
2.3 Experimental design
All bacteria were tested with three different phages and a phage cocktail of all three phages over a variety of concentrations. For all experiments the initial starting bacterial concentration was 106 CFU mL−1 and the phage concentrations tested were 105, 106, 107, and 108 PFU mL−1 for final MOI values of 0.1 to 100. Phage cocktails contained the same number of phages as regular stocks; however, each individual phage corresponded to 1/3 of the total phage population in the cocktail. All experiments were carried out at room temperature (21–23 °C). At the beginning of each experiment, bacterial cultures were started from frozen stocks, streaked on LB plates, transferred to liquid culture, and incubated overnight on an orbital shaker at 125 rpm at room temperature (21–23 °C). All experiments were performed with a final total volume of 10 mL in 25 mL Erlenmeyer flasks for 72 h. Growth was examined by removing 200 μL of solution from the experimental flask and by taking OD620 measurements with a transparent flat-bottom 96 well plate at the following time points of 0, 4, 8, 12, 20, 24, 48, and 72 h on a Thermo Electron Corporation Multiskan Ex spectrophotometer (Vantaa, Finland). Using calibration curves, all OD620 values were converted to CFU mL−1 (Fig. SI 1†). All experiments were performed in triplicate and repeated at least twice. SM buffer was used for the no-phage negative control.
2.4 Creation of the inhibition index
In order to differentiate between the phages that had either a short- or a long-term effect over the course of 72 h, an inhibition index was constructed. The inhibition index for each experiment was calculated by performing a discrete integral and calculating the area under the curve of the log inhibition from the start of the experiment to 72 h (Fig. SI 2†).
2.5 Statistical analysis
All data was analyzed with a one way analysis of variance (ANOVA) using R.24 To assess the effects of phage concentrations on culture growth a Tukey's post hoc test was used to construct a matrix used to compare the differences. Statistically significant values were with a (p < 0.05).
3. Results and discussion
3.1 Phage isolation
In order to represent common pathogens of concern, we selected two Gram-negative bacteria (E. coli and P. aeruginosa) and the Gram-positive bacterium B. cereus. To determine the amount of variability in treatment between different phages for the same bacteria, three unique phages were isolated for each organism, through multiple sampling events over the course of three months. All E. coli and P. aeruginosa phages were found in activated sludge samples. Unique locations generally yielded a unique phage; however, subsequent sampling in the same location and analysis revealed similar restriction patterns to previously isolated phages (Fig. SI 2†). The reoccurrence of similar restriction patterns at the same location over the course of three months suggests a relative stability of the phage populations in the environment; however, further work would be needed to verify these observations. No B. cereus phages were found in activated sludge samples; however, B. cereus phages were present in many of the soil samples isolated from Duke University's campus. The high frequency of B. cereus phages present on Duke's campus are perhaps indicative of the ubiquitous nature of this soil bacterium. Phages were named based on the bacterium they were capable of infecting. E. coli phages were named Ec1, Ec2, and Ec3; P. aeruginosa phages were named Pa1, Pa2, and Pa3; and B. cereus phages were named Bc1, Bc2, and Bc3. These results indicate that the isolation of novel phages is straightforward and therefore it should be relatively easy to isolate phages for a wide variety of organisms specific to the application of interest.
3.2 Phage inhibition of Gram-negative and Gram-positive bacteria
When E. coli, P. aeruginosa, and B. cereus are grown in LB broth at ambient room temperatures and exposed to various phage concentrations there is a very strong inhibition corresponding to 3–4 log over the first 24 h, which gradually decreases but remains statistically significant over the course of 72 h (Fig. 1, 2, and 3). The rich medium selected for this study, while allowing for increased growth, may have altered some of the phage/cell interactions, which may be observed in a more environmentally relevant medium. Coliphage λ has been shown to have different effects depending on the media richness.25 Over the course of the first 8 h there was minimal inhibition with log reductions ranging from 0 to 2.4, these results were probably understated due to the limit of our plate reader to detect low-densities of bacteria. However, for the time periods between 12 and 24 h, there was a strong inhibition with maximum inhibitions occurring at 20 h. Interestingly the E. coli phages Ec1, Ec2, and Ec3 were relatively ineffective after 24 h compared to the phages Pa3, Bc1, and Bc3, which had stronger inhibition past 24 h (Fig. 1, 2, and 3). Phages Pa2, and Bc2 were similar to the E. coli phages in that they were the most effective over the initial 24 h at inhibiting pathogen growth. These results suggest that phages can be used to inhibit pathogen growth for up to 24 h with inhibition values ranging up to 3.6 log CFU mL−1.
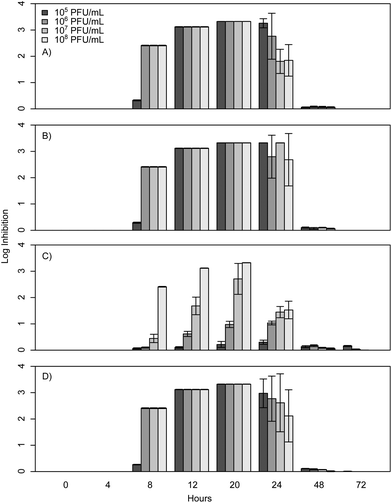 |
| Fig. 1
E. coli K12 phage challenge results with concentrations ranging from 105 to 108 PFU mL−1. Results are expressed as log inhibition in CFU mL−1 over 72 hours and error bars represent the sample standard deviation. (A) Results of Ec1, (B) results of Ec2, (C) results of Ec3, and (D) results of the cocktail. | |
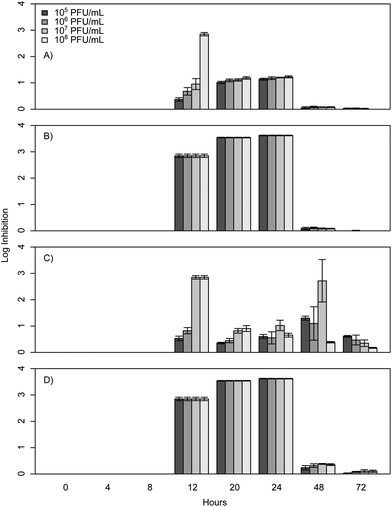 |
| Fig. 2
P. aeruginosa PAO1 phage challenge results with concentrations ranging from 105 to 108 PFU mL−1. Results are expressed as log inhibition in CFU mL−1 over 72 hours and error bars represent the sample standard deviation. (A) Results of Pa1, (B) results of Pa2, (C) results of Pa3, and (D) results of the cocktail. | |
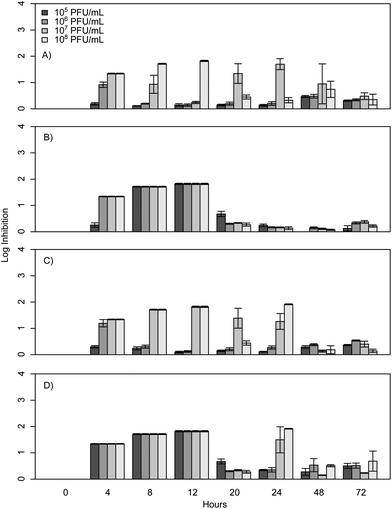 |
| Fig. 3
B. cereus phage challenge results with concentrations ranging from 105 to 108 PFU mL−1. Results are expressed as log inhibition in CFU mL−1 over 72 hours and error bars represent the sample standard deviation. (A) Results of Bc1, (B) results of Bc2, (C) results of Bc3, and (D) results of the cocktail. | |
In the present study, E. coli phage log inhibition ranged up to 3.3 over the course of 20 h and these values are consistent with previous values reported in the literature. These results are similar to work by Viazis et al. who conducted 24 h bacteriophage challenge tests with an enterohemorrhagic E. coli.12 Using a MOI of 100, those phages had between 1 and 6 log reductions over 5 h. However, time points beyond 24 h were not reported. O'Flynn et al. reported a maximum 7-log reduction compared to the control at 6 h at 37 °C with MOIs ranging from 1 to 100 with E. coli 0157:H7.26 However, similarly to Viazis et al. all phage challenged cultures rebounded at 7 h into the experiment at 37 °C. Interestingly, at 30 °C, complete inhibition was reported both with a phage cocktail and individual phages over the course of 8 h, suggesting a temperature dependent effect.
After 20 h, a significant decline was observed in the inhibition of bacterial growth as a result of phage insensitive mutants. For example, no significant inhibition (p > 0.05) was observed at 72 h for Ec1, Ec2, or Ec3. In contrast to these results Sharma et al. reported a 1.8 log reduction over the course of 4 days in planktonic cultures with a MOI greater than 1000 with E. coli O157:H7.16 The phage in the Sharma et al. study performed significantly better than the coliphages isolated for the experiments presented herein, which had 0.0 to 0.2 log reductions at 48 and 72 h. However, it is unclear how much of an effect the phage concentration had on this outcome, as the MOI was at least 10 times greater than the one reported herein or if the phage was more effective over a longer term.
The P. aeruginosa phages isolated herein had a maximum log inhibition of 3.6 and also had similar effects compared to previously reported outcomes. In comparison to results by Kay et al. who used λ W60 with E. coli and PB-1 with P. aeruginosa, all of our phages isolated for P. aeruginosa were more effective than theirs with a MOI of 10 at 24 h, as all of their planktonic cultures had less than 1 log reduction at 24 h.13 For longer time periods, P. aeruginosa phage Pa3 was more effective than PB-1, which suggests that the long-term phage effects are more a property of the phage itself and not as dependent on the concentration.
The results from the B. cereus phages isolated in this study were similar to work by Lee et al.27 However, it should be noted that Lee et al. used a MOI of 0.03 and 0.38 and growth experiments were conducted over 300 min. In contrast to the results reported herein, phage challenge tests with B. cereus in mashed potatoes resulted in a greater than 6 log reduction compared to our maximum of 1.8. However, the matrix was substantially different and may have contributed to the results.
3.3 Effect of phage concentration on bacterial inhibition
To improve the wide scale acceptance of phage bio-control, better methods are needed to predict phage outcomes. This study aims at addressing this by constructing statistical models relating phage concentration to bacterial inhibition for each phage isolated. In order to test if bacteria exhibited a dose response to phage concentration, bacterial cultures were exposed to a range of phage concentrations from 105 to 108 PFU mL−1, corresponding to MOI values of 10−1, 100, 101, and 102 and their growth was monitored for 72 h. Linear models were then fitted to describe inhibition levels at the 20 h time point when the maximum inhibition was observed. The data show that phages Ec3, Pa1, and Pa3 all demonstrated a linear dose response at 20 h into the experiment, whereby increasing phage concentrations had a linear increase in inhibition (Table 1 and Fig. 4). In contrast to the other phages isolated, there was no dose response effect for phages Ec1, Ec2, and Pa3 at 20 h. All phage concentrations led to similar bacterial inhibition (p > 0.05). A possible explanation for this fact is the inability of our plate reader to detect low concentrations of bacteria. Interestingly, at 20 h, B. cereus phages did not exhibit statistically significant relationships with respect to concentration and log inhibition.
Table 1 Intercept, slope, and R2 values for linear models describing inhibition as a function of phage concentration at 20 h
|
Intercept |
Slope |
R
2
|
Ec1 |
3.33 |
0.00 |
NA |
Ec2 |
3.33 |
0.00 |
NA |
Ec3 |
−5.38 |
1.11 |
0.95 |
Pa1 |
0.77 |
0.05 |
0.92 |
Pa2 |
3.54 |
0.00 |
NA |
Pa3 |
−0.69 |
0.20 |
0.89 |
Bc1 |
−0.80 |
0.21 |
−0.16 |
Bc2 |
1.17 |
−0.12 |
0.49 |
Bc3 |
−0.80 |
0.21 |
−0.18 |
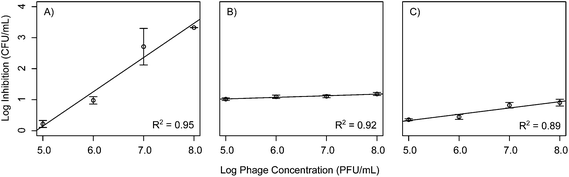 |
| Fig. 4 Linear models for phages (A) Ec3, (B) Pa1, and (C) Pa3 at 20 h. These phages all demonstrated a linear dose response at 20 h into the experiment, where increasing phage concentrations had a linear increase in inhibition. R2 values are noted in the bottom right of each graph. | |
In order to assess the effects of the initial phage concentration in the inhibition index linear models were then constructed comparing phage concentration to the inhibition index. When considering the 72 h inhibition index shown in Table 2, a shift in effect is observed between the phages. Phages Ec3, Pa1, and Bc3 all had linear dose responses when accounting for the total inhibition over the course of 72 h (Fig. 5). This is in contrast to the results for these same phages at 20 h suggesting that some phages might exhibit different dose responses on a variety of time scales. These observations suggest that, for some select phages, higher concentration of phages can be useful up to a certain threshold, at which point higher concentrations have no additive effect. This finding was similar to results by Zhang and Hu who reported on the effects of phage concentration on biofilm formation with chlorine.28 They found that increasing the phage concentration was effective up to a certain point beyond which it was no longer effective to add more phages. This could be due to the fact that at higher concentration of phages, some phages such as T4 are known to exhibit lysis inhibition, which leads to delayed phage release.29
Table 2 Intercept, slope, and R2 values for linear models describing inhibition as a function of phage concentration over the course of 72 h using the log inhibition index
|
Intercept |
Slope |
R
2
|
Ec1 |
112.54 |
−4.77 |
0.58 |
Ec2 |
88.44 |
0.33 |
−0.49 |
Ec3 |
−89.87 |
20.06 |
0.99 |
Pa1 |
−2.49 |
5.39 |
0.78 |
Pa2 |
93.49 |
−0.37 |
0.41 |
Pa3 |
42.38 |
3.04 |
−0.47 |
Bc1 |
−51.20 |
14.36 |
0.40 |
Bc2 |
26.14 |
0.94 |
−0.31 |
Bc3 |
−61.97 |
15.70 |
0.85 |
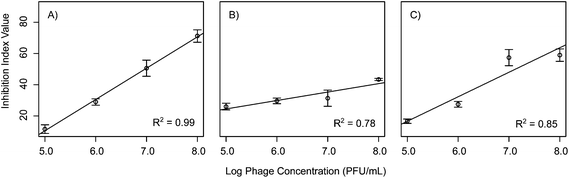 |
| Fig. 5 Linear models for phages (A) Ec3, (B) Pa1, and (C) Bc3 accounting for the total inhibition over 72 hours with the inhibition index. These phages all demonstrated a linear dose response when accounting for the total inhibition over 72 h, where increasing phage concentrations had a linear increase in inhibition. R2 values are noted in the bottom right of each graph. | |
Additionally, it is important to consider how the MOI ratio can affect phage life cycles and treatment outcome. While the phages studied herein all appeared virulent, we were unable to verify completely that they were not lysogenic as we did not carry out phage genomic sequencing, the only fail proof method to identify lysogens.30 For example, some of the isolated phages might be temperate in that a majority of the time (95%) they go through lytic cycles and the other 5% they go into lysogenic cycles. Work with coliphage λ demonstrated that, at higher MOIs, λ is likely to go into a lysogenic cycle, whereas with low MOIs there is a greater chance of lytic cycles.31 Therefore, it is possible at higher MOIs more phages were initially lysogenic, which will lyse later in their life cycle resulting in a latent decrease in culture optical density. However, this is an active area of research and much work is needed to accurately model these parameters.21,22
One additional advantage in screening phages at multiple concentration ranges is that it is possible to identify the lowest acceptable concentration range. For example, while it is relatively easy to obtain high titer 1010 PFU mL−1 phage stocks in the lab, it will be problematic to obtain large volumes of stocks needed for environmental applications in agriculture or animal husbandry. In our study, phages Ec1, Ec2, Pa2, and Bc2 were the most effective at the lowest concentrations and therefore likely to be the best candidates for further studies. These results suggest that by screening various phages, proper candidates can be identified for industrial applications using phage bio-control.
3.4 Effect of phage cocktails compared to phages in isolation
To determine if phage cocktails are more effective than phages in isolation, similar inhibition tests were carried out with cocktails of all three phages compared to each phage in isolation. The results demonstrate that the selected cocktails were only as strong as the most effective phage (Fig. 1, 2, and 3) and there was no statistical difference between the maximum effect of the phages in isolation compared to the cocktail (p > 0.05). The phages herein had max log inhibitions of 3.6. In agreement with our data, Viazis et al. reported that a phage cocktail was generally more effective than most of the individual phages with 5.28–5.53 log reductions; however, some of the phages in isolation, such as BEC8 and phage 39 were just as effective with 5.06 to 5.75 log reductions.12 These results indicate that phage cocktails might not be as effective as previously believed at controlling growth when compared to single phages in isolation, and the efficacy of most cocktails could be due to a single phage group of that cocktail.
4. Conclusions
One of the biggest challenges in phage research is predicting the efficiency of bacterial and phage treatments. This work explored the relationship between phage concentration and long-term bacterial growth inhibition. This study confirms that, for certain phages, phage concentration is an important indicator of treatment outcome, as four of the nine phages (Ec3, Pa1, Pa3, and Ba3) had linear relationships between phage concentration and bacterial inhibition. Unfortunately, due to the variability of outcomes between the phages, a more general model relating phage concentration to inhibition could not be constructed, which highlights the diversity and challenges of working with phages. Further work should be focused on better characterizing the long-term interaction and methods to inhibit the growth of phage insensitive mutants. In addition, follow up experiments should be performed investigating the effect of physical and chemical variability in the treatment waters on treatment efficiency.
Acknowledgements
Funding for this research was provided by the Environmental Protection Agency STAR Fellowship program. Although the research described in the article has been funded by the U.S. Environmental Protection Agency, it has not been subjected to any EPA review and therefore does not necessarily reflect the views of the Agency, and no official endorsement should be inferred.
References
- P. S. McManus, V. O. Stockwell, G. W. Sundin and A. L. Jones, Annu. Rev. Phytopathol., 2002, 40, 443–465 CrossRef CAS PubMed.
- I. Phillips, M. Casewell, T. Cox, B. De Groot, C. Friis, R. Jones, C. Nightingale, R. Preston and J. Waddell, J. Antimicrob. Chemother., 2004, 53, 28–52 CrossRef CAS PubMed.
- T. F. C. Mah and G. A. O'Toole, Trends Microbiol., 2001, 9, 34–39 CrossRef CAS.
- J. W. Costerton, P. S. Stewart and E. P. Greenberg, Science, 1999, 284, 1318–1322 CrossRef CAS.
- L. Guardabassi, A. Petersen, J. E. Olsen and A. Dalsgaard, Appl. Environ. Microbiol., 1998, 64, 3499–3502 CAS.
- J. A. Thomas, J. A. Soddell and D. I. Kurtboke, Water Sci. Technol., 2002, 46, 511–518 CAS.
- J. Choi, S. M. Kotay and R. Goel, Bioengineered bugs, 2011, 2, 214–217 CrossRef.
- J. Zhang, B. L. Kraft, Y. Pan, S. K. Wall, A. C. Saez and P. D. Ebner, Foodborne Pathog. Dis., 2010, 7, 1415–1419 CrossRef PubMed.
- L. D. Goodridge, Trends in biotechnology, 2004, 22, 384–385 CrossRef CAS PubMed.
- K. Mizoguchi, M. Morita, C. R. Fischer, M. Yoichi, Y. Tanji and H. Unno, Appl. Environ. Microbiol., 2003, 69, 170–176 CrossRef CAS.
-
B. K. Chan and S. T. Abedon, in Advances in Applied Microbiology, ed. A. I. Laskin, S. Sariaslani and G. M. Gadd, Elsevier Academic Press Inc, San Diego, 2012, vol. 78, pp. 1–23 Search PubMed.
- S. Viazis, M. Akhtar, J. Feirtag, A. D. Brabban and F. Diez-Gonzalez, J. Appl. Microbiol., 2011, 110, 1323–1331 CrossRef CAS PubMed.
- M. K. Kay, T. C. Erwin, R. J. C. McLean and G. M. Aron, Appl. Environ. Microbiol., 2011, 77, 821–829 CrossRef CAS PubMed.
- T. K. Lu and J. J. Collins, Proc. Natl. Acad. Sci. U. S. A., 2007, 104, 11197–11202 CrossRef CAS PubMed.
- J. J. Curtin and R. M. Donlan, Antimicrob. Agents Chemother., 2006, 50, 1268–1275 CrossRef CAS PubMed.
- M. Sharma, J. H. Ryu and L. R. Beuchat, J. Appl. Microbiol., 2005, 99, 449–459 CrossRef CAS PubMed.
- I. T. Kudva, S. Jelacic, P. I. Tarr, P. Youderian and C. J. Hovde, Appl. Environ. Microbiol., 1999, 65, 3767–3773 CAS.
- C. R. Merril, B. Biswas, R. Carlton, N. C. Jensen, G. J. Creed, S. Zullo and S. Adhya, Proc. Natl. Acad. Sci. U. S. A., 1996, 93, 3188–3192 CrossRef CAS.
- M. D. Eaton and S. Bayne-Jones, J. Am. Med. Assoc., 1934, 103, 1934–1939 CrossRef.
- S. T. Abedon, Foodborne Pathog. Dis., 2009, 6, 807–815 CrossRef PubMed.
-
I. Golding, in Annual Review of Biophysics, ed. D. C. Rees, K. A. Dill and J. R. Williamson, 2011, vol. 40, pp. 63–80 Search PubMed.
- L. Y. Zeng, S. O. Skinner, C. H. Zong, J. Sippy, M. Feiss and I. Golding, Cell, 2010, 141, 682–691 CrossRef CAS PubMed.
-
J. Sambrook, Molecular cloning: a laboratory manual, Cold Spring Harbor Laboratory Press, Cold Spring Harbor, N.Y., 2001 Search PubMed.
-
R Development Core Team, R Foundation for Statistical Computing, Vienna, Austria, 2012 Search PubMed.
- J. W. Little and C. B. Michalowski, J. Bacteriol., 2010, 192, 6064–6076 CrossRef CAS PubMed.
- G. O'Flynn, R. P. Ross, G. F. Fitzgerald and A. Coffey, Appl. Environ. Microbiol., 2004, 70, 3417–3424 CrossRef CAS PubMed.
- W. J. Lee, C. Billington, J. A. Hudson and J. A. Heinemann, Letters in Applied Microbiology, 2011, 52, 456–464 CrossRef CAS PubMed.
- Y. Zhang and Z. Hu, Biotechnol. Bioeng., 2013, 110, 286–295 CrossRef CAS PubMed.
- S. T. Abedon, J. Bacteriol., 1992, 174, 8073–8080 CAS.
- J. J. Gill, E. J. Summer, W. K. Russell, S. M. Cologna, T. M. Carlile, A. C. Fuller, K. Kitsopoulos, L. M. Mebane, B. N. Parkinson, D. Sullivan, L. A. Carmody, C. F. Gonzalez, J. J. LiPuma and R. Young, J. Bacteriol., 2011, 193, 5300–5313 CrossRef CAS PubMed.
- P. Kourilsky and A. Knapp, Biochimie, 1974, 56, 1517–1523 CrossRef CAS.
Footnote |
† Electronic supplementary information (ESI) available. See DOI: 10.1039/c3em00427a |
|
This journal is © The Royal Society of Chemistry 2014 |