DOI:
10.1039/C4DT01634C
(Paper)
Dalton Trans., 2014,
43, 17248-17254
Selective conversion of alcohols in water to carboxylic acids by in situ generated ruthenium trans dihydrido carbonyl PNP complexes†
Received
3rd June 2014
, Accepted 27th June 2014
First published on 27th June 2014
Abstract
In this work, we present a mild method for direct conversion of primary alcohols into carboxylic acids with the use of water as an oxygen source. Applying a ruthenium dihydrogen based dehydrogenation catalyst for this cause, we investigated the effect of water on the catalytic dehydrogenation process of alcohols. Using 1 mol% of the catalyst we report up to high yields. Moreover, we isolated key intermediates which most likely play a role in the catalytic cycle. One of the intermediates was identified as a trans dihydrido carbonyl complex which is generated in situ in the catalytic process.
Introduction
Catalytic oxidation of alcohols is an essential industrial and natural process and leads to important intermediates or products such as aldehydes, ketones or carboxylic acids. Established methods usually require strong and toxic oxidants such as chromium or manganese oxides along with many additives.1–4 In some cases, the use of stoichiometric oxygen supplying reactants or even the presence of pure pressurized oxygen is required.5 In terms of synthesis of carboxylic acids, mostly the oxidation of aldehydes as the intermediates or starting materials is needed.6 The methods of direct oxidation of alcohols to carboxylic acids are still underdeveloped and do not meet today's requirements of a clean and efficient pathway without the need for aggressive and toxic oxidants and avoiding chemical waste products. Despite these disadvantages, only a small number of direct alcohol conversions into carboxylic acids have been reported.7 For example, Stark et al. reported a direct oxidation method of alcohols involving tetra-n-propylammonium perruthenate (TPAP) in the presence of N-methylmorpholine N-oxide (NMO) as a key additive to stabilise the aldehyde hydrate intermediate.8 A different way was obtained by the Grützmacher group; they reported a homogeneous catalytic transformation of alcohols to acids with high yields under very mild conditions applying a rhodium based catalyst with cyclohexanone as a hydrogen acceptor.9,10 With this similar concept, they also succeeded in converting alcohols into esters or amides. The latest method was reported by the Milstein group in 2013 by applying a bipyridine based PNN ruthenium carbonyl hydride catalyst 1 using only water as an oxygen source with no further additives (Fig. 1).11 Usually those pincer type ruthenium complexes bearing cooperative (and hemi-labile) pincer-backbones are known for dehydrogenative coupling of alcohols into esters and their reverse hydrogenation reactions into alcohols, and also for N-alkylation reactions from alcohols and amines.12–15 In the presence of water, catalyst 1 is highly active for catalytic conversion of different alcohols into their corresponding carboxylic acid salts.
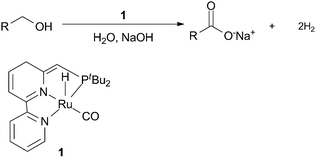 |
| Fig. 1 Direct oxidation of alcohols using a bipyridine ruthenium catalyst 1.11 | |
Other studies on alcohol dehydrogenation in aqueous solution at low temperature, in particular methanol16–18 and methanediol,19 also showed the possibility of acceptorless dehydrogenation. In these certain cases the dehydrogenation resulted in the formation of carbon dioxide and hydrogen gas.
Inspired by the latest achievements, we present a setup using ruthenium PNP pincer complexes [Ru(H2)H2(Me-PNP)] 220 and [RuH2(CO)(Me-PNP)] 3 for catalytic dehydrogenation of primary alcohols in the presence of water, respectively in the absence of any other oxidants (Fig. 2). Our reactions were conducted with aq. NaOH solution as the only additive to obtain the carboxylic acid salts in up to high yields. Furthermore, we isolated complex intermediates 3 and 4a–b separately and from the catalytic process (Fig. 2). In our system, complex 2 serves as a precursor which converts in situ via alcohol decarbonylation reaction into a trans dihydrido complex [RuH2(CO)(Me-PNP)] 3. Separately, complex 3 was used for catalytic alcohol dehydrogenation reactions in water. Based on achievements in earlier reports,12,13,21–25 we investigated the decarbonylation behaviour of a similar PNP pincer based ruthenium complex [Ru(H2)H(PNP)] 5. Complex 5 can be transformed into a carbonyl complex [RuH(CO)(PNP)] 6 and converted into a trans dihydride complex [RuH2(CO)(H-PNP)] 7 under a hydrogen atmosphere (Fig. 2). Those complexes are important intermediates for different transformations reported by others.26,27
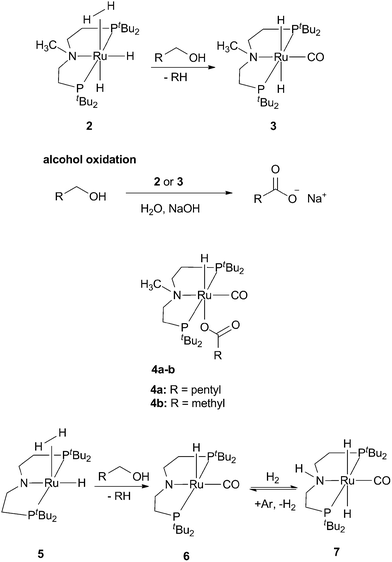 |
| Fig. 2 Ruthenium hydride [Ru(H2)H2(Me-PNP)] 2 and trans dihydrido carbonyl complex [RuH2(CO)(Me-PNP)] 3 for alcohol oxidation, complex intermediates 4a–b, [Ru(H2)H(PNP)] 5 and carbonyl complexes [RuH(CO)(PNP)] 6 and RuH2(CO)(H-PNP)] 7. | |
Results and discussion
Catalytic oxidation of alcohols
For the standard catalytic procedure, a mixture of 2 mL water, 5 mmol of alcohol, 5.5 mmol of NaOH and 1.0 mol% of [Ru(H2)H2(Me-PNP)] 2 or [RuH2(CO)(Me-PNP)] 3 was refluxed under continuous argon flow in an open system for 20 h at 120 °C. The addition of a base (NaOH) is necessary to obtain the carboxylic acid salt and to shift the reaction equilibrium towards the product. After the reaction time, the predominant single aqueous phase was treated with diethyl ether to extract the catalyst. The aqueous layer was then acidified to convert the carboxylic acid salt into its corresponding carboxylic acid which was subsequently extracted with ethyl acetate. Isolated yields of the carboxylic acids are presented in Table 1. In this catalytic oxidation of alcohols we tested a series of aliphatic alcohols along with benzyl alcohol. Best results using catalyst 2 were obtained with hexanol and pentanol yielding 88 and 71% (entries 1 and 2), while butanol gave a moderate yield of 63% (entry 3). Catalysing longer aliphatic chained alcohols (entries 4 and 5), the isolated yields dropped down to 33%. This is probably due to a lack of miscibility of these less polar long-chain aliphatic alcohols with water. Benzyl alcohol and cyclohexyl methanol gave yields between 59 and 65% (entries 6 and 7). After the reaction and extracting the complex with diethyl ether, the organic phase contained only traces of unreacted alcohol, but no ester as a by-product. A slight increase of the yields was obtained with complex 3. The reaction of hexanol to hexanoic acid gave similar yields (entries 1 and 8); for butanol and pentanol (entries 9 and 10) an increase of around 10% was obtained. Isolated yields for octanol and decanol (entries 11 and 12) remained unchanged. The oxidation of benzyl alcohol to benzoic acid improved from 65% yield to 85% (entry 13). In contrast, the conversion of cyclohexyl methanol dropped to 36%. With complexes 5 and 6 the yields for the hexanol oxidation were 53% and 61% (entries 15 and 16).
Table 1 Dehydrogenation of alcohols in the presence of water
Entrya |
Cat. |
Alcohol |
Product |
Yield |
Reaction at 120 °C, 20 h with 1 mol% cat. 2, 3, 5 or 6, 5 mmol alcohol, 5.5 mmol NaOH.
|
1 |
2
|
Hexanol |
Hexanoic acid |
88 |
2 |
2
|
Pentanol |
Valeric acid |
71 |
3 |
2
|
Butanol |
Butyric acid |
63 |
4 |
2
|
Octanol |
Caprylic acid |
42 |
5 |
2
|
Decanol |
Decanoic acid |
33 |
6 |
2
|
Benzyl alcohol |
Benzoic acid |
65 |
7 |
2
|
Cyclohexyl methanol |
Cyclohexyl carboxylic acid |
59 |
8 |
3
|
Hexanol |
Hexanoic acid |
92 |
9 |
3
|
Pentanol |
Valeric acid |
83 |
10 |
3
|
Butanol |
Butyric acid |
73 |
11 |
3
|
Octanol |
Caprylic acid |
45 |
12 |
3
|
Decanol |
Decanoic acid |
32 |
13 |
3
|
Benzyl alcohol |
Benzoic acid |
85 |
14 |
3
|
Cyclohexyl methanol |
Cyclohexyl carboxylic acid |
36 |
15 |
5
|
Hexanol |
Hexanoic acid |
53 |
16 |
6
|
Hexanol |
Hexanoic acid |
61 |
Formation of the active species and characterisation of the isolated complex intermediates
At the beginning of the catalysis, the trans dihydrido carbonyl complex [RuH2(CO)(Me-PNP)] 3 is formed through decarbonylation of the primary alcohol by [Ru(H2)H2(Me-PNP)] 2 (Scheme 1). Separately in another experiment, complex 3 was obtained by adding 3.5 equivalents of ethyl, pentyl or hexyl alcohols to [Ru(H2)H2(Me-PNP)] 2 in a closed system at 80 °C for 48 h, with very good yields, which is stable under an argon atmosphere at room temperature. Furthermore, a series of gas phase mass spectra were recorded to detect the fragmentations of the evolved aliphatic hydrocarbons from the decarbonylation reactions of the corresponding alcohols (ESI Fig. S1–2†). The gas phase MS analysis clearly showed the formation of methane and butane from ethanol, respectively pentanol. Using deuterated ethanol with these hydride catalysts, we observed the formation of CD3H confirming the interaction of the hydride-site with the substrate. Moreover, we confirmed the molar mass of complex 3via the LIFDI-MS technique (ESI Fig. S3†). Mechanistic investigations of decarbonylation reactions with ruthenium complexes were pioneered by Kubas and Caulton.25 Following these observations and other indications,28,29 Sabo-Etienne et al. reported the decarbonylation reaction of alcohol by a molecular dihydrogen ruthenium complex, whereby, similar to our system, the dihydrogen ligand is replaced by a CO ligand.22 Moreover, Foxman and Ozerov reported the CO functionalisation of a PNP type ruthenium pincer hydride complex obtained through decarbonylation of acetone.23 Based on previous reports by Milstein et al., we accordingly assume that a cis-[RuH2(CO)(Me-PNP)] complex is generated in the first step, which undergoes then, despite the high trans influence of the hydride ligands, a rapid cis–trans isomerisation into the thermodynamically more stable and sterically more favourable trans isomer 3.28,30–33 Furthermore, we observed no isomeric change in the 1H- and 31P{1H}-NMR after heating complex 3 at 80 °C for 10 h. [RuH2(CO)(Me-PNP)] 3 shows in the 1H-NMR spectrum at 300 MHz a multiplet assigned to two hydrides at −5.40 ppm. At higher frequencies of 600 MHz, the multiplet resolves into two clean triplet signals at −5.43 ppm (2JHP = 16.1 Hz) and −5.54 ppm (2JHP = 19.3 Hz). Two signals for two hydride signals next to each other in the chemical shifts with a 2JHP coupling constant between 16 and 24 Hz is similar to other reported trans dihydride PNP pincer complexes with aliphatic backbones by Gusev and Schneider.34–37 In the 13C-NMR spectrum, the CO signal was found at 210.8 ppm (t, 2JCP = 13.2 Hz), which was further confirmed by decarbonylation reactions of 13C labeled ethanol (ESI, Fig. S16†). The vCO band was detected at 1871 cm−1 while the comparable 13CO band was found with a Δ43 at 1828 cm−1. Vibration of the hydrides was found at 1642 cm−1 for complex 3 and 1640 cm−1 for the 13C labeled complex (ESI, Fig. S10†). This is in agreement with the case of a typical trans dihydride arrangement. The CO stretching mode is located in the typical range with higher wave numbers followed by the M–H vibrations as one single, sharp band at lower wave numbers.30,32,38 In contrast, for cis bonding modes of metal dihydrides, the hydride trans to a pincer backbone would have the highest wave number, followed then by the CO band and then with the lowest wave number the hydride trans to the carbonyl ligand.38
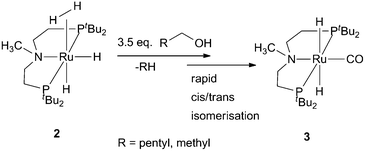 |
| Scheme 1 Decarbonylation of [Ru(H2)H2(Me-PNP)] 2 to [RuH2(CO)(Me-PNP)] 3via cis/trans isomerisation reaction. | |
Comparing the decarbonylation reactivity of 2, similar observations were made from the reaction of the analogue ruthenium complex [Ru(H2)H(PNP)] 5 whose synthesis was reported in earlier studies (Scheme 2).20,26 Decarbonylation reaction of ethanol by complex 5 gave the carbonyl complex [RuH(CO)(PNP)] 6 in excellent yields. In the 1H-NMR spectrum, the hydride ligand gives a triplet signal in the upfield at −20.87 ppm (2JHP = 16.3 Hz), which indicates the configuration of the hydride ligand cis to the pincer ligand.34 Experiments with 13C labeled ethanol resulted in a triplet signal at 208.8 ppm (2JCP = 10.5 Hz) for the CO ligand in the 13CAPT-NMR spectrum. IR signals were found at 1872 cm−1 for the non-labeled νCO vibration along with a weaker νRu–H band at 2052 cm−1 which are characteristic of pincer based carbonyl monohydride compounds.30 For the 13C labeled complex, the 13CO band was detected at 1830 cm−1 and with a νRu–H vibration around 2062 cm−1 (Fig. S13†). Pressurising complex 6 with 1.5 bar H2 gas showed around 79% conversion of 6 into trans dihydride 7, which exhibits, similar to complex 3, two triplet signals at −5.86 ppm (2JHP = 18.2 Hz) and −6.13 ppm (2JHP = 17.4 Hz). Isolation of complex 7 was not possible due to the rapid degeneration into 6.
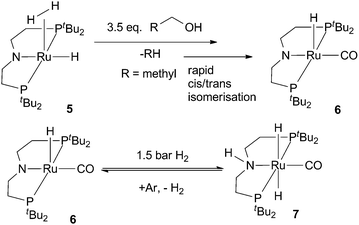 |
| Scheme 2 Decarbonylation of [Ru(H2)H(PNP)] 5 to [RuH(CO)(PNP)] 6via cis/trans isomerisation and the hydrogenation of 6 to [RuH2(CO)(HPNP)] 7. | |
Catalytic cycle and the isolation of intermediates 4a and b
Similar to the system reported by Milstein et al., we assume that complex 3 dehydrogenates the alcohol into an aldehyde intermediate complex. It is also possible that the aldehyde converts independently with water into an aldehyde hydrate intermediate. However, due to the rapid equilibrium between the aldehyde and the aldehyde hydrate intermediate,11 it seems more plausible that the reaction with water under basic conditions generates an aldehyde hydrate, stabilised as a geminial diolate complex, which can be dehydrogenated into the carboxylate complex 4a (Fig. 3).11 From there on, the carboxylate is salted out by sodium cations. In the presence of water, no formation of esters was observed since only unreacted alcohol residues were found in the reaction mixture after the appropriate reaction time. This observation confirms yet again that water suppresses the formation of ester.11 Compared to previous studies11 it is unclear whether the mechanism involves a metal–ligand cooperativity during the catalytic reaction. We achieved the conversion of alcohols to carboxylic acids using catalysts 2 and 3 bearing a “non-cooperative” Me-PNP-ligand. The experimental data show that a basic position as a proton acceptor/donor is not crucial for this reaction as no H/D exchange has been observed in the ligand backbone. The lack of H/D exchange in the ligand backbone lets us tentatively exclude cooperative effects of the ligand. It is likely that the acceptorless dehydrogenation and oxygen-transfer from water solely take place at the ruthenium core.
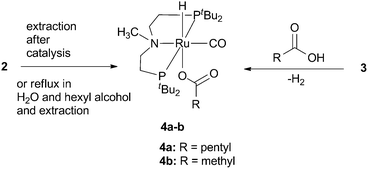 |
| Fig. 3 Isolation of complexes 4a and 4b. Complex 4a was obtained by extraction with toluene after the catalytic reaction with 2 or by refluxing 2 with hexyl alcohol in water. Adding acetic or hexanoic acid to complex 3 led directly to 4a and 4b. | |
The attempt to isolate the complex intermediate species after the reaction time led to the isolation of 4a which was extracted with toluene. Separate attempts led also to the isolation of 4a either by refluxing complex 2 in hexyl alcohol and water or by the reaction of 3 with hexanoic acid (Fig. 3). For the latter one, 4a was obtained in very good yields within minutes under hydrogen evolution. The analogue, complex 4b, was obtained by adding acetic acid to complex 3 (Fig. 3).
Both complexes almost do not differ in their chemical shifts in the 31P{1H} NMR showing singlets around 81.4 ppm (complex 4a) and 81.3 (complex 4b), while exhibiting triplet signals in the upfield at −17.08 ppm (2JPH = 20.7 Hz, complex 4a) and at −17.49 ppm (2JPH = 20.4 Hz, complex 4b). IR spectra show the νCO for both complexes at 1908 cm−1, while exhibiting the νC
O band at 1593 cm−1 (ESI, Fig. S11–12†). LIFDI-MS/MS analysis of complex 4a showed only a fragmentation with the mass value of 506 m/z, which can be explained by the loss of the hexanoate under MS conditions, showing only the carbonyl monohydride species (for more details see ESI, Fig. S5–6†). This observation is in full agreement with our recent experiments applying LIFDI-MS analysis to ruthenium pincer hydride complexes.20 During a soft ionisation process, a mixture of similar fragmentations can be detected with this kind of compound class, which can be explained by the loss of the hydride ligands (−Δ1–2 m/z) causing a shift towards lower mass values.20 In contrast to 4a, LIFDI-MS/MS analysis of 4b revealed the molar mass of 565 m/z, which is in good agreement with the simulated isotope pattern (in red) illustrated in Fig. 4. Compared to the simulated isotope pattern of [RuH(CO)(OOCCH3)(Me-PNP)] 565 m/z in red, the LIFDI-MS/MS pattern is slightly shifted towards lower mass value, which can be explained by the detection of a fragmentation of the subspecies [Ru(CO)(OOCCH3)(Me-PNP)] 564 which is generated during the ionisation process.
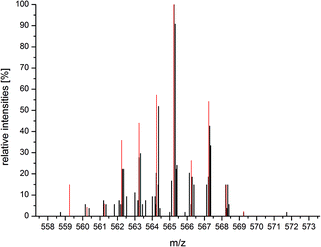 |
| Fig. 4 LIFDI-MS/MS analysis of [RuH(CO)(OOCCH3)(Me-PNP)] 565 4b in toluene. Isotope pattern area 558–570 (black) in comparison with the simulated isotope pattern of [RuH(CO)(OOCCH3)(Me-PNP)] 565 (red). | |
A single crystal structure of 4b was obtained from crystals grown as a red prism in a mixture of benzene and heptane at room temperature (Fig. 5, selected bond distances and angles are given in Table 2). The structure shows a distorted octahedral coordination of the ruthenium core, where both locations of the P-atoms of the P–Ru–P axis are twisted out-of-plane with a P1–Ru–P2 angle of 157.24°. The same applies for the trans-arrangement of the hydride and the carboxylate with an angle of 169.20° (H1–Ru–O2). The X-ray pattern allowed the localisation of the hydride H1 giving a Ru–H distance of 1.57(4) Å. Furthermore, the trans arrangement of the CO ligand to the PNP-ligand is confirmed, which was discussed earlier in this work. The N–Ru–CO angle is closer to 180° (176.16°); consequently the H1–Ru–CO angle is near orthogonal (92.20°).
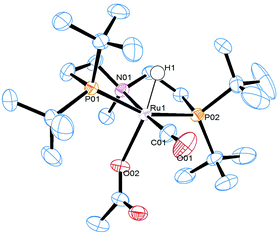 |
| Fig. 5 ORTEP diagram of the single crystal structure of complex 4b. Ellipsoids are illustrated at 50% probability. All hydrogen atoms are not depicted here except for H1 for clarity. | |
Table 2 Selected bond distancesa and anglesb of complex 4b
Distances are given in Å.
Angles are reported in degrees.
|
Ru1–P1 |
2.34(9) |
P1–Ru1–P2 |
157.24 |
Ru1–P2 |
2.34(6) |
N01–Ru1–C01 |
176.16 |
Ru1–C01 |
1.82(0) |
H1–Ru1–O02 |
169.30 |
Ru1–O02 |
2.21(9) |
H1–Ru1–C01 |
92.20 |
Ru1–H1 |
1.57(4) |
|
|
Ru1–N01 |
2.24(7) |
|
|
Conclusions
In summary, we presented an approach for catalytic dehydrogenation of primary alcohols in water yielding carboxylic acid salts using ruthenium hydride complexes. Moreover, we confirmed that complexes 2 and 5 convert in situ into carbonyl trans dihydride complexes 3 and 6 by decarbonylation reaction of alcohols. Complex intermediate 4a, which was isolated after the catalysis as well as synthesised in different ways, is believed to be one of the complex species taking part in the catalytic cycle.
Experimental section
Reactions were generally performed under an argon atmosphere using Schlenk techniques, flame-dried glassware and a Labmaster 200 glove-box from MBraun. High-pressure hydrogen reactions were performed in a Büchi Tinyclave (50 mL) glass autoclave. All solvents and reagents were purchased from Acros, Merck, Sigma-Aldrich, Fluka, or Strem or were acquired from the institute stock. Commercial anhydrous solvents and argon as-packed reagents were used as received and stored in the glove-box under argon. Non-anhydrous solvents were dried and distilled (under vacuum or argon) prior to use, applying standard procedures.‡
Analytical methods
1H-, 13C-, 31P-NMR spectra were recorded at 300 MHz (1H), 75 MHz (13C) and 121 MHz (31P) on a Bruker Avance II 300 and on a Bruker Avance II+ 600 spectrometer at 600 MHz (1H), 150 MHz (13C) and 242 MHz (31P) using deuterated benzene and toluene at room temperature. 1H shifts were reported in ppm (δH) downfield from TMS and were determined by reference to the residual solvent peaks (C6D6: 7.16 ppm, C7D8: 7.09 ppm.). Chemical shifts were reported as singlet (s), doublet (d), triplet (t), quartet (q) and multiplet (m). Coupling constants J were reported in Hz. For hydrogenation experiments, Young-Teflon capped NMR tubes from Wilmad were used. Infrared spectra (IR) were measured at room temperature with a Bruker Alpha spectrometer equipped with a Diamond-ATR IR unit. Data are reported as follows: absorption
[cm−1], weak (w), medium (m), strong (s). Mass-spectrometric investigations of the gas composition in the gas phase were conducted with a HPR-20 gas analysis system by Hiden Analytical and were directly connected to the reaction setup under an argon atmosphere. The HPR-20 QIC (Hiden Analytical) has a MS detection limit <0.09 ppm as xenon in air is detectable. Note that the MS has sensitivity down to partial pressures of 10−10 torr (note: the spectrometer specific unit is torr not MPa).
General catalytic procedure
For the standard catalytic procedure, 0.05 mmol of complex 2 or complex 3 were added to 5.5 mmol NaOH and 5 mmol of alcohol. After the addition of 2 mL degassed water, the content was refluxed at 120 °C for 20 h under constant argon flow in an open system. After the reaction time, the aqueous phase was extracted with diethyl ether to extract the catalyst and alcohol residues. The aqueous layer was then acidified with 20% aq. HCl and treated five times with 20 mL ethyl acetate. After the organic layers were combined and dried for 1 h over MgSO4, the solution was filtered and the solvent was removed under reduced pressure to obtain the isolated carboxylic acid. Yields are given in Table 1.
Synthesis of [RuH2(CO)(Me-PNP)] 3
In an argon flushed Büchi glass autoclave 100 mg (0.198 mmol) of [Ru(H2)H2(Me-PNP)] 2 were dissolved in 6 mL toluene. The synthesis of complex 2 is described in our previous report.20 After the addition of 3.5 eq. (0.693 mmol) of a primary alcohol (e.g. ethyl, pentyl, hexyl alcohol), the content was heated at 80 °C for 48 h. After the appropriate time, the solvent was removed in vacuo and the residue was washed twice with pentane. The grey powder was stored at −34 °C. Yield: 80%.
LIFDI-MS (argon collided): m/z 511.3 (2), 510.3 (19), 509.3 (55), 508.3 (33), 507.3 (100), 506.3 (73), 505.3 (74), 504.3 (65), 503.3 (30), 502.3 (17), 501.3 (22).
1H-NMR: (600 MHz, benzene-d6): δH [ppm] = 2.32 (m, 2H, CH2), 2.11 (s, 3H, NCH3), 1.93 (m, 2H, NCH2), 1.61 (m, 2H, PCH2), 1.55–1.52 (m, 2H, overlapped, PCH2), 1.50 (dt, 36H, 3JPH = 6.7 Hz, PC(CH3)3), −5.43 (t, 1H, 2JPH = 16.1 Hz, Ru–H), −5.54 (t, 1H, 2JPH = 19.4 Hz, Ru–H).
13CAPT-NMR: (75 MHz, benzene-d6): δC [ppm] = 210.8 ppm (t, 2JCP = 13.2 Hz, CO, data extracted from 13CO labeled probe), 65.8 (t, 2JCP = 5.1 Hz, NCH2), 52.9 (NCH3), 36.4 (t, 1JCP = 8.9 Hz, P(C(CH3)3)), 33.9 (t, 1JCP = 7.4 Hz, P(C(CH3)3)), 30.3 (t, 2JCP = 3.3 Hz, P(C(CH3)3), 30.1 (t, 2JCP = 2.9 Hz, P(C(CH3)3)), 24.4 (t, 1JCP = 5.3 Hz, PCH2).
31P{1H}-NMR: (121 MHz, benzene-d6): δP [ppm] = 106.3 (s).
IR:
[cm−1] = 2950–2864 (m), 1871 (s), 1640 (s), 1474 (m), 1458 (m), 1416 (w), 1383 (m), 1381 (m), 1351 (m), 1310 (w), 1208 (w), 1171 (m), 1049 (w), 1025 (m), 930 (w), 915 (w), 881 (m), 801 (m), 739 (m), 679 (m), 644 (w), 613 (m), 566 (m), 529 (w), 508 (w), 478 (m), 432 (m).
Isolation of [RuH(CO)(hexanolate)(Me-PNP)] 4a
In an argon flushed Schlenk flask equipped with a bubbler, 50 mg (0.1 mmol) of [RuH2(CO)(Me-PNP)] 3 were dissolved in 5 mL toluene. After the addition of 1.5 eq. (0.15 mmol) hexanoic acid, the content was stirred for 30 min under a constant stream of argon. The solvent was removed in vacuo and the product was washed twice with pentane. The grey powder, yielding 85%, was stored at −34 °C.
LIFDI-MS/MS (fragment 506): m/z 509.3 (13.1), 508.3 (33.3), 507.3 (16.7), 507.2 (9.5), 506.3 (100), 505.3 (97.6), 504.3 (47.6), 503.3 (16.7), 502.2 (16.7), 501.1 (4.8), 500.2 (9.5).
1H-NMR: (600 MHz, benzene-d6): δH [ppm] = 2.51 (t, 2H, 2JCH = 7.6 Hz, OOCCH2(CH2)3CH3), 2.17 (s, 3H, NCH3), 2.14 (m, 4H, NCH2), 1.70 (m, 2H, OOCCH2CH2(CH2)2CH3), 1.55–1.51 (m, 8H, overlapped, 4H PCH2 and 4H OOC(CH2)2(CH2)2CH3), 1.38 (t, 18H, 3JPH = 6.5 Hz, P(C(CH3)3), 1.23 (t, 18H, 3JPH = 6.1 Hz, P(C(CH3)3), 0.99 (t, 3H, 2JCH = 7.3 Hz, OOC(CH2)4CH3), −17.08 (t, 1H, 2JPH = 20.7 Hz, Ru–H).
13CDeptQ-NMR: (150 MHz, benzene-d6): δC [ppm] = 208.5 ppm (s, CO), 175.8 (s, CH3COO), 65.8 (s, NCH2), 45.6 (s, NCH3), 40.8 (s, OOCCH2(CH2)3CH3), 37.5 (t, 1JPC = 5.1 Hz, P(C(CH3)3)), 36.8 (t, 1JPC = 10.3 Hz, P(C(CH3)3)), 33.0 (s, OOCCH2CH2(CH2)2CH3), 30.6 (s, P(C(CH3)3), 30.5 (s, P(C(CH3)3)), 27.2 (s, OOC(CH2)2CH2CH2CH3), 23.7 (s, OOC(CH2)3CH2CH3), 23.4 (s, PCH2), 14.2 (s, OOC(CH2)4CH3).
31P{1H}-NMR: (121 MHz, benzene-d6): δP [ppm] = 81.4 (s).
IR:
[cm−1] = 2959–2868 (m), 2126–2075 (w), 1908 (s), 1595 (s), 1466 (m), 1429 (w), 1389 (m), 1369 (m), 1354 (m), 1175 (m), 1043 (m), 1024 (m), 958 (w), 933 (w), 907 (w), 879 (m), 828 (w), 807 (m), 736 (m), 680 (m), 643 (m), 609 (m), 570 (m), 546 (m), 531 (m).
Isolation of [RuH(CO)(OOCCH3)(Me-PNP)] 4b
In an argon flushed Schlenk flask equipped with a bubbler, 30 mg (0.06 mmol) of [RuH2(CO)(Me-PNP)] 3 were dissolved in 5 mL toluene. After the addition of 1.5 eq. (0.09 mmol) acetic acid, the content was stirred for 30 min under a constant stream of argon. The solvent was removed in vacuo and the product was washed twice with pentane. The grey powder, yielding 81%, was stored at −34 °C.
LIFDI-MS/MS: m/z 569.2 (1.9), 568.2 (14.8), 567.3 (42.6), 566.3 (18.5), 565.3 (100), 564.4 (51.9), 563.2 (27.8), 561.2 (7.4), 560.2 (5.6).
1H-NMR: (600 MHz, benzene-d6): δH [ppm] = 2.22 (s, 3H, OOCCH3), 2.13 (m, 4H, NCH2), 2.09 (s, 3H, NCH3), 1.63 (m, 2H PCH2), 1.49 (m, 2H, PCH2), 1.31 (t, 18H, 3JPH = 6.4 Hz, P(C(CH3)3), 1.18 (t, 18H, 3JPH = 6.2 Hz, P(C(CH3)3), −17.49 (t, 1H, 2JPH = 20.4 Hz, Ru–H).
13CDeptQ-NMR: (150 MHz, benzene-d6): δC [ppm] = 208.8 ppm (s, CO), 175.4 (s, CH3COO), 65.7 (s, NCH2), 45.2 (s, NCH3), 37.2 (t, 1JCP = 5.3 Hz, P(C(CH3)3)), 36.7 (t, 1JCP = 10.2 Hz, P(C(CH3)3)), 30.5 (s, P(C(CH3)3), 30.2 (s, P(C(CH3)3)), 23.6 (s, PCH2).
31P{1H}-NMR: (242 MHz, benzene-d6): δP [ppm] = 81.3 (s).
IR:
[cm−1] = 2956–2859 (m), 2145–2059 (w), 1906 (s), 1593 (s), 1464 (m), 1389 (m), 1368 (m), 1354 (m), 1259 (s), 1175 (m), 1087 (s), 1021 (s), 934 (w), 907 (w), 878 (m), 800 (s), 735 (m), 680 (m), 609 (m), 569 (m), 546 (w), 529 (w), 478 (m).
Synthesis of [RuH(CO)(PNP)] 6
In an argon flushed Büchi glass autoclave 100 mg (0.215 mmol) of [Ru(H2)H(PNP)] 5 were dissolved in 6 mL toluene. The synthesis of complex 5 is described in our previous report.20 After the addition of 3.5 eq. (0.753 mmol) of a primary alcohol (e.g. ethyl, pentyl, hexyl alcohol), the content was heated at 80 °C for 48 h. After the appropriate time, the solvent was removed in vacuo and the residue was washed twice with pentane. The orange powder was stored at −34 °C. Yield: 90%.
LIFDI-MS/MS: m/z 495.1 (1.0), 494.3 (15.9), 493.3 (46.1), 492.3 (14.6), 491.3 (100), 490.2 (34.7), 489.2 (39.6), 488.3 (28.9), 487.3 (4.6), 486.2 (2.4), 485.3 (15.5).
1H-NMR: (300 MHz, benzene-d6): δH [ppm] = 3.49 (m, 2H, CH2), 3.14 (m, 2H, CH2), 1.88 (m, 4H, PCH2), 1.26 (dt, 36H, 2JPH = 14.3 Hz, PC(CH3)3), −20.87 (t, 1H, 2JPH = 16.3 Hz, Ru–H).
13CAPT-NMR: (75 MHz, benzene-d6): δC [ppm] = 208.8 ppm (t, CO, 2JCP = 10.5 Hz, data extracted from 13CO labeled probe in toluene-d8), 63.5 (t, 2JCP = 7.1 Hz, NCH2), 35.4 (t, 1JCP = 7.7 Hz, P(C(CH3)3)), 33.9 (t, 1JCP = 7.4 Hz, P(C(CH3)3)), 29.7 (t, 2JCP = 3.0 Hz, P(C(CH3)3), 28.5 (t, 2JCP = 3.2 Hz, P(C(CH3)3)), 26.0 (t, 1JCP = 6.9 Hz, PCH2).
31P{1H}-NMR: (121 MHz, benzene-d6): δP [ppm] = 110.1 (s).
IR:
[cm−1] = 2943–2800 (m), 2706 (w), 2628 (w), 2068–2048 (m), 1869 (s), 1469 (m), 1454 (m), 1385 (m), 1358 (m), 1318 (w), 1262 (m), 1206 (m), 1178 (m), 1157 (w), 1106 (w), 1063 (m), 1017 (m), 967 (m), 936 (w), 806 (s), 773 (w), 729 (s), 695 (m), 674 (w), 611 (m), 579 (m), 536 (m), 471 (s).
Hydrogenation of [RuH(CO)(PNP)] 6 to [RuH2(CO)(HPNP)] 7
In a Young-Teflon capped NMR tube, 7 mg (0.014 mmol) [RuH(CO)(PNP)] 6 were dissolved in 0.5 mL deuterated benzene. The content was pressurised with 1.5 bar H2 gas. After 10 h, 79% conversion was detected via31P{1H}-NMR. Only hydride signals are clearly visible.
1H-NMR: (300 MHz, benzene-d6): δH [ppm] = −5.86 (t, 1H, 2JPH = 18.2 Hz, Ru–H), −6.13 (t, 1H, 2JPH = 17.4 Hz, Ru–H).
31P{1H}-NMR: (121 MHz, benzene-d6): δP [ppm] = 110.1 (s, 21%, complex 6), 108.9 (s, 79%, complex 7).
Acknowledgements
We acknowledge the Ministerium für Innovation, Wissenschaft und Forschung NRW (MIWF-NRW) for financial support within the Energy Research Program for the Scientist Returnee Award for M. H. G. Prechtl (NRW-Rückkehrerprogramm). For access to LIFDI-MS analysis, we gratefully acknowledge Prof. Dr T. Braun (Humboldt-University of Berlin, Germany). J.-H. Choi wants to thank Dr J. Neudörfl, T. Heidemann and A. Krest for helpful discussion. P. Kliesen is acknowledged for technical support for the XRD measurement.
Notes and references
- M. Zhao, J. Li, Z. Song, R. Desmond, D. M. Tschaen, E. J. J. Grabowski and P. J. Reider, Tetrahedron Lett., 1998, 39, 5323–5326 CrossRef CAS.
- B. C. Holland and N. W. Gilman, Synth. Commun., 1974, 4, 203–210 CrossRef CAS.
- R. J. Gritter and T. J. Wallace, J. Org. Chem., 1959, 24, 1051–1056 CrossRef CAS.
- M. Zhao, J. Li, E. Mano, Z. Song, D. M. Tschaen, E. J. J. Grabowski and P. J. Reider, J. Org. Chem., 1999, 64, 2564–2566 CrossRef CAS.
- T. Mallat and A. Baiker, Chem. Rev., 2004, 104, 3037–3058 CrossRef CAS PubMed.
-
G. Tojo, Oxidation of Primary Alcohols to Carboxylic Acids: A Guide to Current Common Practice (Basic Reactions in Organic Synthesis), Springer, 2010 Search PubMed.
- G.-J. t. Brink, I. W. C. E. Arends and R. A. Sheldon, Science, 2000, 287, 1636–1639 CrossRef PubMed.
- A.-K. C. Schmidt and C. B. W. Stark, Org. Lett., 2011, 13, 4164–4167 CrossRef CAS PubMed.
- T. Zweifel, J.-V. Naubron and H. Grützmacher, Angew. Chem., Int. Ed., 2009, 121, 567–571 CrossRef.
- S. Annen, T. Zweifel, F. Ricatto and H. Grützmacher, ChemCatChem, 2010, 2, 1286–1295 CrossRef CAS.
- E. Balaraman, E. Khaskin, G. Leitus and D. Milstein, Nat. Chem., 2013, 5, 122–125 CrossRef CAS PubMed.
- J. Zhang, M. Gandelman, L. J. W. Shimon, H. Rozenberg and D. Milstein, Organometallics, 2004, 23, 4026–4033 CrossRef CAS.
- J. Zhang, G. Leitus, Y. Ben-David and D. Milstein, J. Am. Chem. Soc., 2005, 127, 12429–12429 CrossRef CAS.
- C. Gunanathan, Y. Ben-David and D. Milstein, Science, 2007, 317, 790–792 CrossRef CAS PubMed.
- J. Zhang, M. Gandelman, L. J. W. Shimon and D. Milstein, Dalton Trans., 2007, 107–113 RSC.
- R. E. Rodriguez-Lugo, M. Trincado, M. Vogt, F. Tewes, G. Santiso-Quinones and H. Grutzmacher, Nat. Chem., 2013, 5, 342–347 CrossRef CAS PubMed.
- E. Alberico, P. Sponholz, C. Cordes, M. Nielsen, H. J. Drexler, W. Baumann, H. Junge and M. Beller, Angew. Chem., Int. Ed., 2013, 52, 14162–14166 CrossRef CAS PubMed.
- M. Nielsen, E. Alberico, W. Baumann, H. J. Drexler, H. Junge, S. Gladiali and M. Beller, Nature, 2013, 495, 85–89 CrossRef CAS PubMed.
- L. E. Heim, N. E. Schloerer, J.-H. Choi and M. H. G. Prechtl, Nat. Commun., 2014, 5, 3621, DOI:3610.1038/ncomms4621.
- J.-H. Choi, N. E. Schloerer, J. Berger and M. H. G. Prechtl, Dalton Trans., 2014, 43, 290–299 RSC.
- E. P. K. Olsen and R. Madsen, Chem. – Eur. J., 2012, 18, 16023–16029 CrossRef CAS PubMed.
- P. D. Bolton, M. Grellier, N. Vautravers, L. Vendier and S. Sabo-Etienne, Organometallics, 2008, 27, 5088–5093 CrossRef CAS.
- R. Çelenligil-Çetin, L. A. Watson, C. Guo, B. M. Foxman and O. V. Ozerov, Organometallics, 2005, 24, 186–189 CrossRef.
- Y.-Z. Chen, W. C. Chan, C. P. Lau, H. S. Chu, H. L. Lee and G. Jia, Organometallics, 1997, 16, 1241–1246 CrossRef CAS.
- L. S. Van der Sluys, G. J. Kubas and K. G. Caulton, Organometallics, 1991, 10, 1033–1038 CrossRef CAS.
- B. Askevold, J. T. Nieto, S. Tussupbayev, M. Diefenbach, E. Herdtweck, M. C. Holthausen and S. Schneider, Nat. Chem., 2011, 3, 532–537 CrossRef CAS PubMed.
- Z. B. Han, L. C. Rong, J. Wu, L. Zhang, Z. Wang and K. L. Ding, Angew. Chem., Int. Ed., 2012, 51, 13041–13045 CrossRef CAS PubMed.
- B. N. Chaudret, D. J. Cole-Hamilton, R. S. Nohr and G. Wilkinson, J. Chem. Soc., Dalton Trans., 1977, 1546–1557 RSC.
- T. M. Douglas and A. S. Weller, New J. Chem., 2008, 32, 966–969 RSC.
- B. Rybtchinski, Y. Ben-David and D. Milstein, Organometallics, 1997, 16, 3786–3793 CrossRef CAS.
- B. L. Shaw and M. F. Uttley, J. Chem. Soc., Chem. Commun., 1974, 918–919 RSC.
- H. Salem, L. J. W. Shimon, Y. Diskin-Posner, G. Leitus, Y. Ben-David and D. Milstein, Organometallics, 2009, 28, 4791–4806 CrossRef CAS.
- R. S. Paonessa and W. C. Trogler, J. Am. Chem. Soc., 1982, 104, 1138–1140 CrossRef CAS.
- M. Bertoli, A. Choualeb, A. J. Lough, B. Moore, D. Spasyuk and D. G. Gusev, Organometallics, 2011, 30, 3479–3482 CrossRef CAS.
- A. Friedrich, M. Drees, J. Schmedt auf der Günne and S. Schneider, J. Am. Chem. Soc., 2009, 131, 17552–17553 CrossRef CAS PubMed.
- M. Kaess, A. Friedrich, M. Drees and S. Schneider, Angew. Chem., Int. Ed., 2009, 48, 905–907 CrossRef CAS PubMed.
- A. Friedrich, M. Drees, M. Käss, E. Herdtweck and S. Schneider, Inorg. Chem., 2010, 49, 5482–5494 CrossRef CAS PubMed.
- S. M. Kloek, D. M. Heinekey and K. I. Goldberg, Organometallics, 2006, 25, 3007–3011 CrossRef CAS.
Footnotes |
† Electronic supplementary information (ESI) available. CCDC 1002031. For ESI and crystallographic data in CIF or other electronic format see DOI: 10.1039/c4dt01634c |
‡ For the Experimental section, limited spectral and crystallographic data, see ESI. |
|
This journal is © The Royal Society of Chemistry 2014 |
Click here to see how this site uses Cookies. View our privacy policy here.