DOI:
10.1039/C3BM60289C
(Paper)
Biomater. Sci., 2014,
2, 567-580
A nanopatterned cell-seeded cardiac patch prevents electro-uncoupling and improves the therapeutic efficacy of cardiac repair†
Received
13th November 2013
, Accepted 19th December 2013
First published on 24th January 2014
Abstract
The heart is an extremely sophisticated organ with nanoscale anisotropic structure, contractility and electro-conductivity; however, few studies have addressed the influence of cardiac anisotropy on cell transplantation for myocardial repair. Here, we hypothesized that a graft's anisotropy of myofiber orientation determines the mechano-electrical characteristics and the therapeutic efficacy. We developed aligned- and random-orientated nanofibrous electrospun patches (aEP and rEP, respectively) with or without seeding of cardiomyocytes (CMs) and endothelial cells (ECs) to test this hypothesis. Atomic force microscopy showed a better beating frequency and amplitude of CMs when cultured on aEP than that from cells cultured on rEP. For the in vivo test, a total of 66 rats were divided into six groups: sham, myocardial infarction (MI), MI + aEP, MI + rEP, MI + CM–EC/aEP and MI + CM–EC/rEP (n ≥ 10 for each group). Implantation of aEP or rEP provided mechanical support and thus retarded functional aggravation at 56 days after MI. Importantly, CM–EC/aEP implantation further improved therapeutic outcomes, while cardiac deterioration occurred on the CM–EC/rEP group. Similar results were shown by hemodynamic and infarct size examination. Another independent in vivo study was performed and electrocardiography and optical mapping demonstrated that there were more ectopic activities and defective electro-coupling after CM–EC/rEP implantation, which worsened cardiac functions. Together these results provide comprehensive functional characterizations and demonstrate the therapeutic efficacy of a nanopatterned anisotropic cardiac patch. Importantly, the study confirms the significance of cardiac anisotropy recapitulation in myocardial tissue engineering, which is valuable for the future development of translational nanomedicine.
1. Introduction
Tissue engineering is an emerging and promising approach aiming to reconstruct biometric substitutes and to replace damaged tissue.1–3 To achieve this goal, it relies on multidisciplinary principles and techniques, including engineering, materials science, chemistry and biology.4–7 By this we may solve the limitation of organ transplantation and regenerate impaired tissues and organs.2,3 To achieve this goal, an engineered construct should have several features: (i) nano-scale fibers rather than micro-scale fibers of the scaffold to mimic the natural extracellular matrix (ECM, 10–300 nm in diameter) and to provide more attach sites for cells, (ii) tissue-specific organizational structure, (iii) a vascular network to supply blood and nutrients from systemic circulation and (iv) sufficient mechanical strength to withstand physiological forces.
Heart disease is the leading cause of death worldwide,8 and myocardial tissue engineering may offer new opportunities to repair the failing heart.1,9 However, the organizational structure of the heart is particularly critical for rebuilding the myocardium because it holds the most sophisticated architecture in our body.10–12 The individual cardiac cell aligns in a specific direction at the microscopic level so that the myocardium reveals unsymmetrical mechano-electrical properties, which enable the heart to contract and pump blood at the macroscopic level. This unique feature is termed cardiac anisotropy and should be carefully addressed when mending the injured heart. For example, inappropriate transplantation methods may result in regional heterogeneity and myofiber orientation discordance between the host and grafted cells and thus induce lethal arrhythmia.13,14
To address the aforementioned concerns, an increasing number of studies have developed in vitro biomimetic cardiac constructs with anisotropic morphological and mechano-electrical characteristics using various advanced engineering techniques.15–20 Despite this enthusiasm, animal studies demonstrating the successful recapitulation of cardiac anisotropy via cell-seeded constructs continue to be relatively lacking.1,21 Two recent studies have demonstrated the therapeutic benefit of implanting cardiac progenitor cells on anisotropic scaffolds; however, both studies focused on the enhanced ability of the nanopatterned substrates to promote differentiation of the progenitor cells into cardiomyocytes (CMs) in culture.22,23 The sequential interaction of these differentiated CMs and the host heart remains unclear. In addition, there is not yet a study directly comparing the in vivo effects and contributions of anisotropic or unpatterned constructs seeded with CMs. Therefore, at present the importance and essentiality of the anisotropy of the cardiac construct is neglected, which should be crucial for future clinical application and realization.
Here, we conducted experiments to test whether the implantation of a cell-seeded nanopatterned patch leads to a better therapeutic outcome in a rat model of experimental MI. Electrospinning techniques have been developed to generate fibers ranging from tens to hundreds of nanometers in diameter that resemble fibers from the natural extracellular matrix.24,25 Consequently, applications using electrospinning to produce tissue engineering scaffolds, drug delivery vehicles, and medical implants have been developed.25–28 Another advantage of electrospinning is its versatility, which can be attributed to a multifarious selection of polymers to manipulate the physical and chemical properties and fulfill different needs, such as the mechanical strength, degradability, drug-carrying capacity and the orientation of the fibers.29–31 Subsequently, to prevent preconditioned anisotropy from being affected after material degradation, nondegradable polyacrylonitrile (PAN) was chosen to fabricate the aligned and randomly oriented nanofibrous electrospun patches (aEP and rEP, respectively).
CMs and endothelial cells (ECs) play an essential role in cardiac functions and are susceptible to ischemic damage.32 Furthermore, EC co-culture is reported to be beneficial for CMs in vitro and in vivo.33–35 In this study, CMs and ECs were seeded on aEP or rEP and the properties of the graft, including anisotropy of myofiber orientation, contractility, electro-conductivity and therapeutic efficacy in vitro and in vivo, were examined.
2. Materials and methods
2.1. Materials
The fabrication PAN electrospun nanofibers or microfibers were accomplished as previously described.36 The electrospinning apparatus consisted of a glass syringe, a syringe pump, a high-voltage power supply and a grounded collector. The polymer solution was prepared by dissolving PAN (Scientific Polymer Products) in 99.8% N,N-dimethylformamide (Tedia) at a concentration of 8% or 12% (w/w) for yield of nanofibers or microfibers, respectively. The solution was then loaded into a glass syringe with a stainless steel nozzle and continuously forced through the nozzle using a syringe pump (KD Scientific) at a constant rate of 1 ml per hour. A voltage of 20 kV was applied over a distance of 15 cm between the nozzle and the grounded collector. The PAN polymer solution formed a Taylor cone, and when the electric force overcame the surface tension of the droplet from the apex of the cone, a charged fluid jet was ejected. Once the solvent evaporated, the PAN nanofibers or microfibers deposited on the collector formed non-woven mats. An aligned orientation of the fibers was achieved using 2 trips of ferrite magnet collectors separated by a gap. The electrospun patch (EP) was vacuum-dried at room temperature overnight. The structure and morphology of the electrospun nanofibers were observed using a field emission scanning electron microscope (JSM-6700F; JEOL). The fiber orientation was determined from digital images using Image J software. In brief, a reference line was drawn on each image, and the acute angle formed between the reference and the principal axis of individual fibers was recorded.
2.2. Hydrophilic and hydrophobic testing of the EPs
The liquid–gas surface tension was measured using a tensiometer (FTA 1000B; First Ten Angstroms). The contact angles were measured for each sample using 10 μl water droplets. Interfacial tension measurements of a suspended water drop were performed prior to experimentation to verify the water purity and precision of the focused image. The contact angles were determined by drop shape analysis from a series of images collected at a rate of 2 frames per s.
2.3. Cell isolation
Rat neonatal CMs and adult ECs were isolated from Sprague–Dawley rats modified as previously described.33,34 Briefly, CMs were isolated from 1- to 2-day-old rats, and the cardiac ventricles were dissected, minced, and digested with 1 mg ml−1 trypsin (Sigma-Aldrich) in HBSS (Gibco) for 3 hours at 4 °C. The digested tissues were further incubated in 0.8 mg ml−1 collagenase type II (Worthington) at 37 °C. The tissue extract was filtered through a 40 μm mesh and then pre-plated for 30 minutes twice on a 10 cm cell culture dish to allow fibroblasts to attach. The unattached cells, in which more than 99% were CMs, were used in subsequent experiments.
ECs were isolated from the fat tissue of adult Sprague–Dawley rats by collagenase type I digestion (Worthington). The collected tissue extract was first pre-plated for 90 minutes on a 10 cm cell culture dish to allow fibroblasts to attach. Then the cells were suspended and incubated with anti-rat PECAM-1-coated Dynal beads (BD Biosciences). A magnetic separator was used to recover the bead-bound cells. The cells were washed and then cultured in gelatin-coated tissue culture flasks in Medium 199 (M199; Gibco) supplemented with 15% fetal bovine serum (FBS; Hyclone), 5% heparin (Sigma-Aldrich), 1% penicillin–streptomycin (Gibco) and 1% endothelial cell growth supplement (Biomedical Technologies). The purity of ECs was confirmed to be more than 99% by flow cytometry, using vWF staining. ECs at passages 4–10 were used for subsequent experiments.
2.4. Cell culture
The patches were autoclaved and placed in a 24 well plate immersed in 1% gelatin for 20 minutes before cell seeding. The ECs and CMs were seeded onto the patch at densities of 2 × 104 and 2 × 105 cells per well, respectively. The doubling time of cultured ECs is ∼1 day, while the freshly isolated CMs are almost impossible to proliferate. We used the chosen cell-seeding density to allow the patch to be covered by ECs and CMs with a ratio 1 to 1 after 3 days’ culture. This is the best ratio for ECs and CMs that has been demonstrated in previous studies.33,34 ECs were cultured in medium M199 with 15% FBS, and CMs were cultured in low-glucose DMEM with 10% FBS. For co-culture, the ECs were pre-seeded for 1 day and then the freshly isolated CMs were added. At the same time, the medium was replaced with a mixture of the M199/DMEM media at a ratio of 1
:
1. The medium was refreshed every 2 days, and the cultures were maintained in a tissue culture incubator at 37 °C with 95% air and 5% CO2.
2.5. Cell adhesion assay
ECs and CMs were cultured for 1 hour on aligned or randomly oriented EPs in 24 well plates at a density of 2 × 105 cells per well. The samples were fixed in 4% paraformaldehyde at room temperature for 2 hours and rinsed twice with PBS. The nuclei were then counterstained with 0.1 μg ml−1 DAPI (Sigma-Aldrich) in PBS for 10 minutes and rinsed with PBS. Fluorescence images were acquired and analyzed using a High Throughput Screening Microscope (ImageXpress; Molecular Devices). The obtained data were analyzed by cell counting (MetaXpress; Molecular Devices).
2.6. Cell viability assay
For cell viability assays, ECs and CMs were cultured on aligned or randomly oriented EPs in 24 well plates at densities of 2 × 104 and 2 × 105 cells per well, respectively, for up to 5 days. At the end of the incubation, 40 μl of a 3-(4,5-dimethylthiazol-2-yl)-2,5-diphenyltetrazolium bromide (MTT) solution and 500 μl of the M199 medium were added to each well and incubated for 2 to 4 hours until a purple precipitate was visible. Following the removal of the MTT solution, 1 ml of dimethyl sulfoxide (DMSO; Invitrogen) was added to each well and pipetted up and down to dissolve the crystals. Then, 200 μl of the medium was transferred to a 96 well plate reader, and the absorbance was measured at 570 nm.
2.7. Immunocytochemical staining
Samples were fixed in 4% paraformaldehyde at room temperature for 2 hours and rinsed twice with PBS. Typical H&E staining was performed on EC cultures. Immunofluorescent staining was performed for CM cultures and CM–EC co-cultures. In brief, samples were treated with 0.1% Triton X-100 for 1 hour at room temperature to permeabilize the cell membranes for antibody binding and washed 3 times with PBS for 5 minutes. After incubation with a blocking buffer (5% goat serum and 5% FBS) for 1 hour at room temperature, the samples were incubated with isolectin (Invitrogen), cardiac tropomyosin (CH1; DSHB) or connexin 43 (Abcam) primary antibodies in a blocking buffer at 4 °C overnight. Subsequently, the samples were washed 3 times with PBS for 5 minutes and incubated with the secondary antibodies (Invitrogen). Finally, the nuclei were counterstained with 0.1 μg ml−1 DAPI (Sigma-Aldrich) in PBS for 10 minutes and then washed with PBS. The cell orientation analysis was determined using a method similar to the fiber orientation analysis described above.
2.8. Measurement of the physiological properties of CMs using atomic force microscopy
The procedure for measuring the CM physiological properties with atomic force microscopy (AFM) was adopted from Liu et al.37 A silicon nitrite tipless cantilever (k = 0.03 N m−1; Nanoworld) was attached to a 0.25 μm radius glass bead at its tip. The thermal noise method was used to calibrate the cantilever. To initiate a measurement, the cantilever was loaded on the CM with 0.1 nN of pre-load force, and the vertical deflection of the cantilever was recorded for more than 20 seconds. The beating rate and amplitude of the CM could then be calculated from the recording. The vertical deflection was then converted into a force according to Hooke's Law: F = ks, where F is the force, k is the spring constant and s is the distance. Based on the spring constant (k) of the cantilever determined during the calibration, the force (F) generated from the cell leading to the vertical deflection (s) of the cantilever was calculated. There were 2 criteria for the measurement: (1) the cell must be contracting and (2) the cells must fit the description of the testing condition. That is, if the cardiac patch was meant to be aligned, only the cells growing in between the aligned patch would be measured; if the patch was meant to be random, then only the cells growing in the midst of a random patch would be measured.
2.9. Rat myocardial infarction model and electrospun patch implantation
All of the animal protocols were approved by the Institutional Animal Care and Use Committee at NCKU. Adult male Sprague–Dawley rats weighing 200 g to 250 g were used and randomly allocated to the different conditions. After the rats were anesthetized, the hearts were exposed through a left thoracotomy. Myocardial infarction (MI) was then induced via the ligation of the left coronary artery (LCA) with a 6-0 polypropylene suture, causing permanent LCA occlusion. Subsequently, cardiac patches with or without seeded cells were sutured along the margin of the MI area to cover the defect created in the left ventricle. After surgery, the chest was closed, and the rats were removed from the respirator. Rats without the LCA ligation (sham-operated group) and those subjected to surgery but receiving no further treatments (MI group) served as controls. For patches with seeded cells (CM–EC/aEP or CM–EC/rEP), ECs and CMs were co-cultured 3 days before the surgery, and the procedure was the same as that used in vitro, except that CMs were pre-labeled with DiI (Invitrogen) according to the manufacturer's protocol.
2.10. Echocardiography
Cardiac functions were assessed by echocardiography, which was performed using a 17.5 MHz probe (Vevo 770; Visualsonic) at 1 day, 1 month and 2 months after the operation. The animals were anesthetized with isoflurane. The left ventricle ejection fraction and left ventricular internal dimensions at end-systole and end-diastole were measured.
2.11. Hemodynamics
At 2 months post-MI, the hemodynamic parameters were assessed by direct ventricular catheterization immediately after echocardiography using 2.0 Fr. pressure–volume sensing catheters (Millar Instruments). The catheter was inserted into the right carotid artery and advanced into the left ventricle (LV). After stabilization, baseline LV pressure–volume loops were recorded. To change the preload, the inferior vena cava was transiently compressed through an incision in the upper abdomen. At the end of each catheterization, 50 μl of 25% saline was injected into the right atrium through the right jugular vein to determine the conductance. The volume calibration and hemodynamic data were analyzed with commercial software (PVAN3.2; Millar Instruments).
2.12. Tissue processing and immunohistochemical staining
After the animals were euthanized, the left ventricles were harvested, washed in PBS and fixed in 4% paraformaldehyde at 4 °C for 24 hours prior to embedding in paraffin wax. Deparaffinized and rehydrated myocardial sections from the 3 different sites of infarction (starting from the suture site as the “higher infarct”, the site between the higher infarct and apex as the “lower infarct”, and the site between the two former section planes as the “middle infarct”) were subjected to Masson's trichrome staining. The infarct length ratio, thickness and collagen deposition at the remote regions were analyzed as previously described,38 and the average of the aforementioned sections was calculated. The evaluations of the vessel connections and leukocyte infiltration were conducted using H&E staining. Leukocytes present in the myocardial infarct foci were counted by a blinded experienced pathologist in 5 fields of view under oil immersion (1000×) in higher density areas from rats of each group. The number of positive cells was expressed as the mean number of cells per field for the statistical analysis.
2.13. Mechanical testing of the myocardium
After the rats were euthanized, the hearts were immediately excised and kept hydrated in ice-cold HBSS. Before testing, the right ventricle was removed, and the remaining left ventricle was dissected along the longitudinal axis and trimmed into dumbbell-shaped specimens (12 mm gauge length, 8 mm width) with the infarct region in the middle. Specimens were mounted on a Tytron 250 Microforce Testing System (MTS System Corporation), which was controlled by TestStar System software with custom fabricated stainless steel tissue grips. The specimens were strained to failure at a rate of approximately 1% per second.
2.14. Fluorescent immunohistochemistry
Deparaffinized and rehydrated sections were first incubated in a blocking buffer (5% goat serum and 5% FBS) for 1 hour at room temperature and then in cTnI (DSHB) and SM22α (Abcam) primary antibodies at 4 °C overnight. Subsequently, the sections were washed 3 times with PBS for 5 minutes and incubated with FITC-conjugated Alexa Fluor secondary antibodies (Invitrogen). Finally, the nuclei were counterstained with 0.1 μg ml−1 DAPI and observed using fluorescence microscopy (Zeiss). The vessel density was quantified by blinded observers using images of the patched region, as previously described.39
2.15.
In vivo electrocardiography and ex vivo optical mapping
The rats were placed under inhalation anesthesia, and a surface 3-lead electrocardiography (EKG) recording was obtained for at least 10 minutes at 1 day after the operation. The QRS durations were measured and normalized by the individual R–R interval. The same animals were subjected to optical mapping measurements by an experienced electrophysiologist at 7 days after the surgery. The conduction velocity from the healthy area to the infarct zone of the rat ventricular myocardium was measured as previously described.40 In brief, the ascending aorta was cannulated, and the heart was perfused with oxygenated Tyrode solution at a flow rate of 5 ml min−1. The perfusion pressure was maintained within a range of 80–100 mmHg. The rat hearts were stained with 15 ml of Tyrode solution plus 30 μl of 2 mM di-4-ANEPPS in DMSO to monitor the cardiac action potential and paced (3 Hz, 3 ms duration, 3-fold threshold voltage) at the basal portion of the right ventricle using a stimulator (S88J; Grass). After the cardiac contraction was blocked by the addition of 10 μM cytochalasin D (Sigma-Aldrich), the action potentials were recorded using optical mapping. Isochronal maps of the activation spread and conduction velocity measurements were obtained using Cardioplex software (RedShirtImaging).
2.16. Statistical analysis
The results are presented as the mean ± SEM. Statistical differences were determined using two-tailed unpaired t-tests, one-way repeated-measure analyses of variance (ANOVA) with Newman–Keuls post-hoc tests to compare mean values between multiple groups, or two-way ANOVA with subsequent Bonferroni's post-hoc tests to compare mean values between multiple groups at different time points. A value of P < 0.05 was considered statistically significant.
3. Results and discussion
3.1. Cell morphology is regulated by anisotropic patterns
Electrospun nanofibrous thin films with an aligned or random orientation were collected respectively with or without magnetic field guidance. The thin films of several micrometers thickness were then trimmed into an elliptical shape with a long axis of 10 mm and a short axis of 8 mm, with the nanofiber alignment along the long axis, for further use as an EP. Scanning electron microscopy (SEM) showed that both the aligned and randomly orientated nanofibers were approximately 320 ± 32 nm in diameter (Fig. 1A). The orientation of the fibers in aEP varied by less than ±10° (Fig. 1B and C). The aEP showed a higher Young's modulus than rEP did before implantation (Fig. S1†). Since the elasticity of cells ranges between 1 and 100 kPa,41 and it is much lower than that of the patches (10–20 MPa), the contribution of cells to the mechanical property of patches, aEP or rEP, should be small.
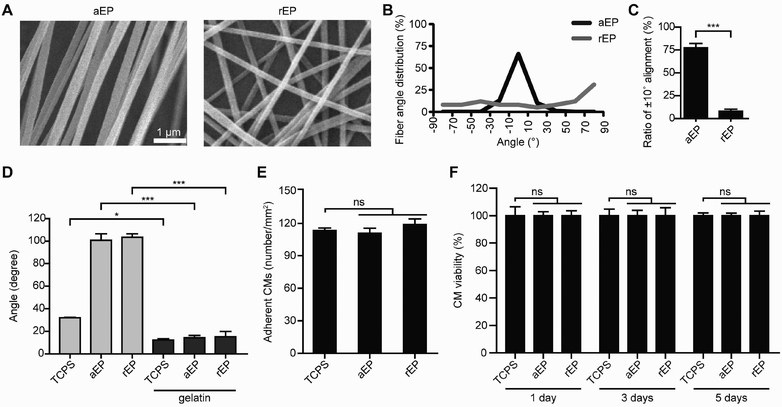 |
| Fig. 1 Anisotropic or unpatterned nanofibrous electrospun patches have a similar hydrophilicity, cell-adhesive tendency and non-toxicity as that of tissue-culture polystyrene dishes after gelatin modification. (A) Representative SEM images of aEP and rEP, (B) the angle distribution of fibers, and (C) the ratio of ±10° alignment. The data are presented as the mean ± SEM (n = 3–6 per group). (D) Quantification of the contact angles on various substrates. The data are presented as the mean ± SEM (n = 3–4 per group). (E) Cell adhesion of CMs cultured under various conditions. (F) The cell viability of CMs cultured on various substrates. The data are presented as the mean ± SEM (n = 6–9 per group). ns, not significant; *P < 0.05, ***P < 0.001. | |
Both aEP and rEP were initially hydrophobic and not favorable for cell attachment (Fig. 1D). For the sake of subsequent cell seeding, the surface properties of aEP and rEP were modified to become hydrophilic by coating with gelatin, which is a derivative of collagen and contains abundant cell binding sequences.26 The cell affinity tests using rat CMs or ECs demonstrated a similar cell-adhesive tendency of aEP and rEP to that of tissue-culture polystyrene (TCPS) dishes (Fig. 1E and Fig. S2A†). Furthermore, there was no significant CM or EC apoptosis when the cells were cultured on aEP or rEP compared with TCPS at various time points, confirming that aEP and rEP are not cytotoxic (Fig. 1F and Fig. S2B†). Importantly, the CMs or ECs aligned in an orderly manner when cultured on aEP compared with the control, in which no alignment was observed (Fig. 2A–C and Fig. S2C–E†). These findings demonstrated that cell morphology and arrangement can be regulated by the topological features of the substrate.
 |
| Fig. 2 Morphology of cardiomyocytes is regulated by anisotropic electrospun patches. (A) Representative immunostaining images of CMs cultured alone on aEP or rEP for 1, 3 and 5 days, (B) the angle distribution of CMs, and (C) the ratio of ±10° alignment of CMs. (D) Representative immunostaining images of CMs cultured with ECs on aEP or rEP for 1, 3 and 5 days, (E) the angle distribution of CMs, and (F) the ratio of ±10° alignment of CMs. The cells were stained with cardiac tropomyosin (red) and isolectin (green) and the nuclei were stained with DAPI (blue). (G) Quantification of the CM length at 3 days under different culturing conditions. The data are presented as the mean ± SEM (n = 3–6 per group). *P < 0.05, **P < 0.01, ***P < 0.001, compared with rEP unless otherwise indicated by brackets. | |
3.2. Anisotropic nanofibrous feature and endothelial co-cultivation optimize the function of a cardiac construct
We have previously reported that there is a close interaction between ECs and CMs within the native myocardium, and co-cultivation with ECs is able to promote CM survival, spatial reorganization and gap junction protein expression.32–34 Therefore, we tested whether the alignment can be further enhanced when CMs were co-cultured with pre-seeded ECs on aEP or rEP. The results showed that CMs aligned not only more uniformly but also more rapidly when co-cultured with ECs on aEP relative to the results for CMs alone (Fig. 2D–F). In addition, the CMs were lengthened when cultured on aEP, and this effect was further enhanced when they were co-cultured with ECs (Fig. 2G).
AFM has been recently used to measure the mechanical properties of cells or tissues.37,42,43 Therefore, we used AFM to investigate whether the physiological properties of CMs were mediated by topological cues (Fig. 3A). The results demonstrated that the beating rate and amplitude of CMs were significantly promoted when the CMs were cultured on aEP rather than rEP (Fig. 3B–D), indicating that both the morphology and cellular behavior were modulated by the aligned nanofibers of the substrate. It is noteworthy that the aforementioned benefits of the aEP alone were further enhanced by EC co-cultivation (Fig. 3B–D). These results suggest that CMs prefer the microenvironment established by aEP with EC co-cultivation.
 |
| Fig. 3 The physiological properties of cardiomyocytes are optimized under endothelial co-culture on aligned electrospun patches. (A) Representative images of AFM measurements and (B) representative deflection-versus-time curves from a single cardiomyocyte under various conditions. (C) Beating frequency and (D) vertical deflection detected with atomic force microscopy. The data are presented as the mean ± SEM (n = 3–7 per group). *P < 0.05, **P < 0.01, ***P < 0.001. | |
The natural ECM ranges from 10 to 300 nm in diameter while the native cardiac ECM is reported to be more than 100 nm in diameter.18,44–46 To investigate whether nanoscale structure is necessary for a biomimetic cardiac patch, we fabricated microfibrous cardiac patches with a diameter of 1081 ± 74 nm (Fig. S3A†) and compared the CM responses after cultivation on microfibrous and nanofibrous substrates. We found that CMs had lower adhesive ability (Fig. S3B†) and reduced viability (Fig. S3C†) when cultured on microfibers than those cultured on nanofibers. Compared to those seeded on TCPS or a nanofibrous substrate, CMs cultured on a microfibrous substrate also showed an atypical round shape (Fig. S3D†) and a weaker beating force (Fig. S3E†). Together these results suggest that a CM- and EC-seeded aligned nanofibrous patch may be the most appropriate cardiac construct for in vivo therapy.
3.3. Post-MI heart functions are preserved by patch implantation alone
To examine the therapeutic efficacy of the cardiac patches, we created a rat MI model and implanted either aEP or rEP with or without CM–EC co-cultivation onto the infarcted epicardium (Fig. 4A and B). There was no obvious mortality (Fig. S4†) induced by the EP implantation, demonstrating the surgical safety of the EP. At 2 months after implantation, the EP remained adhered to the epicardium with connective tissue covering its surface (Fig. 4C). Echocardiographic assessment revealed that the implantation of aEP or rEP alone significantly preserved the left ventricle ejection fraction (LVEF) from deterioration at 1 and 2 months post-MI compared with the baseline at 1 day post-MI (Fig. 4D). Accordingly, post-MI LV dilatation was significantly retarded after aEP or rEP implantation, as demonstrated by reduced LV end-diastolic dimension (LVEDD) and end-systolic dimension (LVESD) compared with control (Fig. S5†). Hemodynamic examination also demonstrated similar beneficial trends of aEP or rEP implantation at 2 months post-MI (Fig. 4E–N), including reduced LV dilation (evaluated based on the LV end diastolic and systolic volumes) and ameliorated systolic and diastolic functions (indexed as the LV end-diastolic pressure, dP/dtmax and dP/dtmin).
 |
| Fig. 4 Transplantation of cells with aligned electrospun patches improves cardiac function after infarction. (A) Experimental procedure flow chart. (B) Schematic diagram of the patch implantation after MI in rats. The upper panel shows a representative picture of a heart with EP implantation. The lower panel shows a sketch of the myocardial fiber orientation after implanting the EP with cells. (C) Representative images of rat hearts with or without cardiac patch implantation at 2 months post-MI. The white dotted region indicates the infarct region, and the white arrows indicate the 6 EP suture sites. (D) The cardiac function was determined based on the LVEF at 1 day, 1 month and 2 months post-MI measured by echocardiography. (E) Hemodynamic parameters including the end systolic volume, (F) end diastolic volume, (G) end diastolic pressure, (H) dP/dtmax, (I) dP/dtmin, (J) time constant of LV pressure decay according to the Weiss method (τ), (K) end-systolic pressure–volume relationship (ESPVR), (L) preload recruitable stroke work (PRSW), (M) dP/dtmax − EDV and (N) elastance max were measured by catheterization at 2 months post-MI. The data are presented as the mean ± SEM (n = 10–13 per group). *P < 0.05, **P < 0.01, ***P < 0.001, compared with MI unless otherwise indicated in brackets. | |
Previous studies have reported the importance of preserving ventricle geometry for functional maintenance post-MI.39,47–49 Similarly, the ex vivo histological examination demonstrated that aEP or rEP implantation prevented cardiac remodeling post-MI, as demonstrated by a smaller infarct size (Fig. 5A–C) and a thicker infarcted myocardium compared with control (with or without deduction of the patch thickness; Fig. 5D and E). The collagen content at the remote area was also significantly reduced after aEP or rEP implantation (Fig. 5F and G), indicating improved cardiac compliance and diastolic function. The scarred myocardium showed a higher Young's modulus than the normal myocardium as quantified by ex vivo mechanical property testing; however, the stiffness (the value of Young's modulus multiplied by the thickness, which is defined as the capability to resist deformation via an outer force) of the scar tissue was too weak to withstand cardiac dilatation because of ventricular wall thinning (Fig. 5H–J). In contrast, the aEP- or rEP-mended myocardium exhibited stiffness similar to that of the sham group, with a similar Young's modulus and thickened myocardial wall. In summary, the aEP or rEP implantation preserved cardiac function and prevented the heart from undergoing cardiomyopathy post-MI.
 |
| Fig. 5 Implantation of electrospun patches alone prevents pathological remodeling after infarction via mechanical support. (A) Sketch of the myocardial sectioning for staining in rats. (B) Representative images of Masson's trichrome staining from each treatment group post-MI. The arrows indicate the location of the EP. Histograms showing the ratio of (C) the infarct length, (D) the infarct size relative to patch size, (E) the thickness of the infarct with patch and (F) the thickness of the infarct alone. (G) Representative images of the collagen content at the remote area for each treatment group post-MI. (H) Statistical analysis of the collagen content of the images in G. The data are presented as the mean ± SEM (n = 6–12 per group). (I) Stress–strain curves of the myocardium after infarction and patch implantation. Quantification of (J) Young's modulus and (K) stiffness of the myocardium after infarction and patch implantation. The data are presented as the mean ± SEM (n = 3–5 per group). *P < 0.05, **P < 0.01, ***P < 0.001, compared with MI unless otherwise indicated in brackets. | |
3.4 Post-MI heart functions are improved by anisotropic cardiac construct implantation but worsen by unpatterned cardiac construct implantation
The LVEF was improved when the aEP with CM–EC co-cultivation was implanted, as shown by echocardiography (Fig. 4D). The cardiac improvement was further supported by restrained ventricular dilation (Fig. 4E and F and Fig. S5†), increased cardiac systolic and diastolic function (indexed by the LVEDP, dP/dtmax, dP/dtmin, stroke work, time constant of LV pressure decay, preload recruitable stroke work, end-systolic pressure–volume relationship, maximum chamber elasticity and dP/dtmax-end-diastolic volume relation; Fig. 4G–N), moderated infarct size (Fig. 5B–F) and reduced collagen deposition at the remote area (Fig. 5G and H). In accordance with the in vitro findings, these results suggest that aEP combined with CM–EC co-cultivation ameliorates cardiac performance for at least 2 months post-MI.
Surprisingly, although in vitro examination revealed a poorer performance of CMs cultured on an unpatterned patch, it was an unexpected finding that the cardiac function deteriorated after CM–EC/rEP implantation and it was worse than that after aEP or rEP implantation alone (Fig. 4D–N and Fig. S5†). We provided further evidence that severe cardiac remodeling was observed after CM–EC/rEP implantation at 2 months post-MI (Fig. 5B–D, G and H). The above-mentioned data suggest that the transplanted cells provide beneficial or harmful effects depending on whether they were patterned to satisfy the host cardiac anisotropy.
Interestingly, both CM–EC/aEP and CM–EC/rEP implantation resulted in better neovascularization at the region between the transplanted patch and the host myocardium (Fig. 6). A functional vascular connection, via the detection of red blood cells from vessels within the transplanted patch, was also revealed in both groups (Fig. 7A). Consistent with the findings reported by Sekine et al., these results highlighted another favorable effect of EC co-cultivation, which is to provide the cardiac construct with an improved blood supply.35 It is noteworthy that we identified that more DiI+ pre-labeled CMs could be observed in the CM–EC/aEP than the CM–EC/rEP group at 2 months post-MI (Fig. 7B). Furthermore, DiI+ CMs in the CM–EC/aEP group were well aligned along the host CMs. Nonetheless, the number of DiI+ CMs in both groups was much lower than expected, suggesting that long-term survival of the transplanted cells remains a major challenge of the present study. These results also imply that cardiac outcomes are affected by factors other than long-term contribution of these cells.
 |
| Fig. 6 Implantation of a cell-seeded cardiac patch increases arteriogenesis at the border regions between the patch and the infarcted myocardium at 2 months after infarction. (A) Immunostaining of smooth muscle cells (SM22α; green) and nuclei (DAPI; blue) from each group. Images of the MI group at the peri-infarct region served as controls. White dashed lines indicate the interface between the host myocardium and the implanted cardiac construct. Quantification of (B) arteriole (external vascular diameter <75 μm) and (C) artery (external vascular diameter >75 μm) densities in A. The data are presented as the mean ± SEM (n = 5–8 per group). *P < 0.05, **P < 0.01. | |
 |
| Fig. 7 Transplantation of cells with aligned electrospun patches promotes functional integration between the graft and the host myocardium at 2 months after infarction. (A) Functional vascular connection between the transplanted cardiac construct and the host circulation is revealed. The left panel shows representative images of whole-heart cross sections subjected to H&E staining from each treatment group post-MI. White dashed lines indicate the interface between the host myocardium and the implanted cardiac construct. Magnified images are shown in the middle panel, and the area inside the boxes is highly magnified, as shown in the right panel. The vessels within the cardiac constructs are functional and are connected to the host circulation, as indicated by the presence of red blood cells within the vessel (arrow). (B) Transplanted cardiomyocytes are found to be well aligned with the host cardiomyocytes. Representative fluorescent immunostaining images of transplanted (DiI; red) and host cardiomyocytes (cTnI; green) and the nuclei (DAPI; blue) from each treatment group post-MI. White dashed lines indicate the interface between the implanted cardiac construct and the host myocardium. The magnified images are shown in the middle (merged) and right (DiI only) panels. Transplanted DiI+ CMs are indicated by arrows. | |
3.5 Cardiac outcomes are determined by electro-coupling and cardiac protection at the early stage post-MI
As demonstrated by previous studies including ours, an early-stage protection of the remaining cardiomyocytes leads to improved cardiac function at a later stage.34,50,51 Consistently, we found that the transplanted cells seeded on aEP protected a greater number of host CMs against apoptosis at 1 day after MI (Fig. S6†). In contrast, significantly more native CMs underwent apoptosis at 1 day after CM–EC/rEP implantation, suggesting the detrimental effect of cell transplantation with a non-anisotropic patch in the early period. The most possible cause of cardiac protection is the paracrine effect from implanted cells, especially when these cells were well primed in a CM–EC coculture manner as previously reported.32–35 The extracellular biophysical cues are also known to regulate the intracellular signaling and thus affect cellular functions such as the secretion of autocrine or paracrine factors.22,23,52–54 Similarly, we found that CMs seeded on aEP showed an elevated gene expression level of beneficial factors, including hematopoietic lineage substrate-1-associated protein X-1 (HAX-1), hepatocyte growth factor (HGF), stromal cell-derived factor-1α (SDF-1α), and vascular endothelial growth factor (VEGF) (Fig. S7†). These factors have been reported to play an important role in promoting cardiomyocyte survival after injury.55–60 Besides, inflammation mediation is considered as another important early-stage factor; however, our results showed that there was no significant inflammatory difference among treatment groups at 1 day post-MI (Fig. S8†). It may be due to the immunomodulatory effects from the implanted adipose derived stromal vascular fraction cells.61 Together, this finding suggests that inflammation may not play a conclusive role in affecting CM apoptosis.
Proarrhythmic risk is one of the major side effects of cardiac cell therapy, especially in the early stage after cell transplantation.14,62 In addition, increasing evidence has shown that irregularity of the heart rhythm can provoke cardiac dysfunction and vice versa.63 Accordingly, we presumed that cardiac aggravation after CM–EC/rEP implantation was attributed to electrical un-coupling in the early period. Thus, we performed another independent study to investigate cardiac electrophysiology at 1 day after MI among different treatment groups using in vivo EKG examination (Fig. 8A). The EKG waveforms of the MI + CM–EC/aEP group were similar to those of the sham group, whereas the waveforms of the MI + CM–EC/rEP group and the MI group were similar, showing ectopic activities in the left ventricle. The quantification of the QRS duration also revealed that CM–EC/aEP implantation improved the post-MI ventricular electro-conductivity to the same level as that of the sham group, whereas the MI + CM–EC/rEP group had slower conductivities similar to those of the MI group (Fig. 8B). Our in vitro data further demonstrated a higher expression of connexin 43, which is the most important junction protein that connects neighbor CMs for electro-coupling, on the CMs seeded on aEP compared to the control (Fig. S9A and B†). In addition, we found that connexin 43 was distributed at the interface between individual CMs when seeded on aEP. In comparison, connexin 43 on CMs seeded on rEP was distributed around the nucleus (Fig. S9C†).
 |
| Fig. 8 Transplantation of cells with unpatterned electrospun patches fails to achieve electro-coupling between the graft and the host myocardium after infarction. (A) Representative images of electrocardiography at 1 day post-MI and (B) histogram showing the normalized QRS duration for each group. (C) Freshly harvested heart on the Langendorff perfusion system; the region of interest, outlined in black, was subjected to optical mapping. (D) Representative optical mapping images from each treatment group at 7 days post-MI. Rhythmic impulses were stimulated for pacing from the host myocardium at the peri-infarct region. (E) Histograms showing the ratio of the conduction velocity from a remote region to the infarct region and (F) the incidence of ventricular tachycardia in the various groups. The data are presented as the mean ± SEM (n = 4–9 per group). **P < 0.01, ***P < 0.001. | |
Following the EKG examination, we performed an ex vivo optical mapping assessment at 7 days post-MI (Fig. 8C). Under rhythmic impulse pacing from outside the region of interest, the action potential propagated smoothly from the upper-left region to the lower-right region in the sham group, whereas the action potential of the MI group from the border to the infarct region moved slowly (Fig. 8D). Importantly, both the anisotropic electro-conduction pattern and conductive velocity were preserved after the implantation of CM–EC/aEP (Fig. 8E). In contrast, there was an appearance of a conduction block between the host myocardium and the cardiac patch in the MI + CM–EC/rEP group, causing decreased conductive velocity and a different pattern in which the action potential traveled around the block before reaching the infarct zone. Furthermore, there was more induced ventricular tachycardia under pacing in the MI + CM–EC/rEP group than in other groups (Fig. 8F). Taken together, these results demonstrate that the CM–EC/rEP implantation causes ventricular arrhythmias, which may be due to myofiber misalignment between the host myocardium and the transplanted cells.14
4. Conclusion
Overall, this study provides a proof-of-concept study showing the beneficial effects and necessity of cardiac anisotropy when developing cardiac patches. We demonstrated cardiac advancement when transplanted cardiomyocytes were grafted on an anisotropic nanofibrous patch. In contrast, cardiac deterioration occurred when exogenous cells were transplanted with an unpatterned patch, which may be due to the inadequate electro-coupling between the graft and the host. To meet clinical demand, the effective therapeutic time frame requires further examination. Other promising cell types, such as autologous cardiac progenitor cell-derived CMs or inducible pluripotent stem cell-derived CMs, should also be tested in the future.2,64 Moreover, whether customized, vascularized and multilayered cardiac patching can be produced using currently available techniques, including non-invasive diffusion tensor magnetic resonance imaging to determine the cardiac muscle fiber orientation and stacking of multilayer cell sheets, remains to be demonstrated.10,65 In conclusion, this study provides a potential solution for cardiac repair and demonstrates the significance of the anisotropic nature of the heart when applying cell transplantation as a treatment.
Acknowledgements
This work was supported by the National Science Council grants 100-2314-B-006-046, 101-2325-B-006-013 and 99-2628-B-006-029; the National Health Research Institutes grant EX102-10123SI and EX102-10026SI; the National Research Program for Biopharmaceuticals grants DOH102-TD-PB-111TM019 and DOH102-TD-PB-111-TM020; and the Academia Sinica Translational Medicine Program. P.C.H.H. received research support from Celgene Cellular Therapeutics. We gratefully acknowledge T. Chu, Y. Shih and W. Lin for their technical assistance of surgery, echocardiography and electrocardiography, respectively (National Cheng Kung University); the optic-image core lab for their support of FACS (fluorescence-activated cell sorting)-like tissue cytometry and a high throughput screening microscope (National Cheng Kung University Hospital); and M. Springer (University of California at San Francisco), J. Lin (National Cheng Kung University) and S. Roffler (Academia Sinica) for their critical comments.
References
- G. Vunjak-Novakovic, K. O. Lui, N. Tandon and K. R. Chien, Annu. Rev. Biomed. Eng., 2011, 13, 245–267 CrossRef CAS PubMed
.
- L. M. Ptaszek, M. Mansour, J. N. Ruskin and K. R. Chien, Lancet, 2012, 379, 933–942 CrossRef
.
- E. T. Pashuck and M. M. Stevens, Sci. Transl. Med., 2012, 4, 160sr4 Search PubMed
.
- M. P. Lutolf and J. A. Hubbell, Nat. Biotechnol., 2005, 23, 47–55 CrossRef CAS PubMed
.
- E. S. Place, N. D. Evans and M. M. Stevens, Nat. Mater., 2009, 8, 457–470 CrossRef CAS PubMed
.
- T. Dvir, B. P. Timko, D. S. Kohane and R. Langer, Nat. Nanotechnol., 2011, 6, 13–22 CrossRef CAS PubMed
.
- S. F. Badylak, D. J. Weiss, A. Caplan and P. Macchiarini, Lancet, 2012, 379, 943–952 CrossRef
.
- A. S. Go, D. Mozaffarian, V. L. Roger, E. J. Benjamin, J. D. Berry, W. B. Borden, D. M. Bravata, S. Dai, E. S. Ford, C. S. Fox, S. Franco, H. J. Fullerton, C. Gillespie, S. M. Hailpern, J. A. Heit, V. J. Howard, M. D. Huffman, B. M. Kissela, S. J. Kittner, D. T. Lackland, J. H. Lichtman, L. D. Lisabeth, D. Magid, G. M. Marcus, A. Marelli, D. B. Matchar, D. K. McGuire, E. R. Mohler, C. S. Moy, M. E. Mussolino, G. Nichol, N. P. Paynter, P. J. Schreiner, P. D. Sorlie, J. Stein, T. N. Turan, S. S. Virani, N. D. Wong, D. Woo and M. B. Turner, Circulation, 2013, 127, e6–e245 CrossRef PubMed
.
- R. K. Iyer, L. L. Y. Chiu, L. A. Reis and M. Radisic, Curr. Opin. Biotechnol., 2011, 22, 706–714 CrossRef CAS PubMed
.
- P. P. Sengupta, J. Korinek, M. Belohlavek, J. Narula, M. A. Vannan, A. Jahangir and B. K. Khandheria, J. Am. Coll. Cardiol., 2006, 48, 1988–2001 CrossRef PubMed
.
- H. C. Ott, T. S. Matthiesen, S. K. Goh, L. D. Black, S. M. Kren, T. I. Netoff and D. A. Taylor, Nat. Med., 2008, 14, 213–221 CrossRef CAS PubMed
.
- K. R. Chien, I. J. Domian and K. K. Parker, Science, 2008, 322, 1494–1497 CrossRef CAS PubMed
.
- S. Fukushima, A. Varela-Carver, S. R. Coppen, K. Yamahara, L. E. Felkin, J. Lee, P. J. R. Barton, C. M. N. Terracciano, M. H. Yacoub and K. Suzuki, Circulation, 2007, 115, 2254–2261 CrossRef PubMed
.
- H. S. V. Chen, C. Kim and M. Mercola, Circulation, 2009, 120, 2496–2508 CrossRef PubMed
.
- A. W. Feinberg, A. Feigel, S. S. Shevkoplyas, S. Sheehy, G. M. Whitesides and K. K. Parker, Science, 2007, 317, 1366–1370 CrossRef CAS PubMed
.
- G. C. Engelmayr, M. Cheng, C. J. Bettinger, J. T. Borenstein, R. Langer and L. E. Freed, Nat. Mater., 2008, 7, 1003–1010 CrossRef CAS PubMed
.
- W. Bian, B. Liau, N. Badie and N. Bursac, Nat. Protoc., 2009, 4, 1522–1534 CrossRef CAS PubMed
.
- D. H. Kim, E. A. Lipke, P. Kim, R. Cheong, S. Thompson, M. Delannoy, K. Y. Suh, L. Tung and A. Levchenko, Proc. Natl. Acad. Sci. U. S. A., 2010, 107, 565–570 CrossRef CAS PubMed
.
- J. C. Nawroth, H. Lee, A. W. Feinberg, C. M. Ripplinger, M. L. McCain, A. Grosberg, J. O. Dabiri and K. K. Parker, Nat. Biotechnol., 2012, 30, 792–797 CrossRef CAS PubMed
.
- S. R. Shin, S. M. Jung, M. Zalabany, K. Kim, P. Zorlutuna, S. b. Kim, M. Nikkhah, M. Khabiry, M. Azize, J. Kong, K. T. Wan, T. Palacios, M. R. Dokmeci, H. Bae, X. Tang and A. Khademhosseini, ACS Nano, 2013, 7, 2369–2380 CrossRef CAS PubMed
.
- E. Chavakis, M. Koyanagi and S. Dimmeler, Circulation, 2010, 121, 325–335 CrossRef PubMed
.
- A. F. G. Godier-Furnémont, T. P. Martens, M. S. Koeckert, L. Wan, J. Parks, K. Arai, G. Zhang, B. Hudson, S. Homma and G. Vunjak-Novakovic, Proc. Natl. Acad. Sci. U. S. A., 2011, 108, 7974–7979 CrossRef PubMed
.
- D. H. Kim, Kshitiz, R. R. Smith, P. Kim, E. H. Ahn, H. N. Kim, E. Marban, K. Y. Suh and A. Levchenko, Integr. Biol., 2012, 4, 1019–1033 RSC
.
- W. Liu, S. Thomopoulos and Y. Xia, Adv. Healthcare Mater., 2012, 1, 10–25 CrossRef CAS PubMed
.
- S. H. Lim and H. Q. Mao, Adv. Drug Delivery Rev., 2009, 61, 1084–1096 CrossRef CAS PubMed
.
- S. A. Sell, M. J. McClure, K. Garg, P. S. Wolfe and G. L. Bowlin, Adv. Drug Delivery Rev., 2009, 61, 1007–1019 CrossRef CAS PubMed
.
- H. S. Yoo, T. G. Kim and T. G. Park, Adv. Drug Delivery Rev., 2009, 61, 1033–1042 CrossRef CAS PubMed
.
- M. A. Alamein, Q. Liu, S. Stephens, S. Skabo, F. Warnke, R. Bourke, P. Heiner and P. H. Warnke, Adv. Healthcare Mater., 2013, 2, 702–717 CrossRef CAS PubMed
.
- C. Wang, K. W. Yan, Y. D. Lin and P. C. H. Hsieh, Macromolecules, 2010, 43, 6389–6397 CrossRef CAS
.
- D. Grafahrend, K. H. Heffels, M. V. Beer, P. Gasteier, M. Möller, G. Boehm, P. D. Dalton and J. Groll, Nat. Mater., 2011, 10, 67–73 CrossRef CAS PubMed
.
- B. Tian, J. Liu, T. Dvir, L. Jin, J. H. Tsui, Q. Qing, Z. Suo, R. Langer, D. S. Kohane and C. M. Lieber, Nat. Mater., 2012, 11, 986–994 CrossRef CAS PubMed
.
- P. C. H. Hsieh, M. E. Davis, L. K. Lisowski and R. T. Lee, Annu. Rev. Physiol., 2006, 68, 51–66 CrossRef CAS PubMed
.
- D. A. Narmoneva, R. Vukmirovic, M. E. Davis, R. D. Kamm and R. T. Lee, Circulation, 2004, 110, 962–968 CrossRef PubMed
.
- P. C. H. Hsieh, M. E. Davis, J. Gannon, C. MacGillivray and R. T. Lee, J. Clin. Invest., 2006, 116, 237–248 CrossRef CAS PubMed
.
- H. Sekine, T. Shimizu, K. Hobo, S. Sekiya, J. Yang, M. Yamato, H. Kurosawa, E. Kobayashi and T. Okano, Circulation, 2008, 118, S145–S152 CrossRef CAS PubMed
.
- S. F. Li and W. T. Wu, Biochem. Eng. J., 2009, 45, 48–53 CrossRef CAS PubMed
.
- J. Liu, N. Sun, M. A. Bruce, J. C. Wu and M. J. Butte, PLoS One, 2012, 7, e37559 CAS
.
- Y. D. Lin, C. Y. Luo, Y. N. Hu, M. L. Yeh, Y. C. Hsueh, M. Y. Chang, D. C. Tsai, J. N. Wang, M. J. Tang, E. I. H. Wei, M. L. Springer and P. C. H. Hsieh, Sci. Transl. Med., 2012, 4, 146ra109 Search PubMed
.
- Y. D. Lin, M. L. Yeh, Y. J. Yang, D. C. Tsai, T. Y. Chu, Y. Y. Shih, M. Y. Chang, Y. W. Liu, A. C. L. Tang, T. Y. Chen, C. Y. Luo, K. C. Chang, J. H. Chen, H. L. Wu, T. K. Hung and P. C. H. Hsieh, Circulation, 2010, 122, S132–S141 CrossRef CAS PubMed
.
- Y. J. Lai, C. L. Hung, R. C. Hong, Y. M. Tseng, C. I. Lin, Y. S. Ko, C. H. Tsai and H. I. Yeh, J. Biomed. Sci., 2011, 18, 72 CrossRef CAS PubMed
.
- A. J. Engler, S. Sen, H. L. Sweeney and D. E. Discher, Cell, 2006, 126, 677–689 CrossRef CAS PubMed
.
- M. Stolz, R. Gottardi, R. Raiteri, S. Miot, I. Martin, R. Imer, U. Staufer, A. Raducanu, M. Duggelin, W. Baschong, A. U. Daniels, N. F. Friederich, A. Aszodi and U. Aebi, Nat. Nanotechnol., 2009, 4, 186–192 CrossRef CAS PubMed
.
- M. Plodinec, M. Loparic, C. A. Monnier, E. C. Obermann, R. Zanetti-Dallenbach, P. Oertle, J. T. Hyotyla, U. Aebi, M. Bentires-Alj, Y. H. LimRoderick and C. A. Schoenenberger, Nat. Nanotechnol., 2012, 7, 757–765 CrossRef CAS PubMed
.
- M. M. Stevens and J. H. George, Science, 2005, 310, 1135–1138 CrossRef CAS PubMed
.
- C. P. Barnes, S. A. Sell, E. D. Boland, D. G. Simpson and G. L. Bowlin, Adv. Drug Delivery Rev., 2007, 59, 1413–1433 CrossRef CAS PubMed
.
- D. H. Kim, P. P. Provenzano, C. L. Smith and A. Levchenko, J. Cell Biol., 2012, 197, 351–360 CrossRef CAS PubMed
.
- B. I. Jugdutt, Circulation, 2003, 108, 1395–1403 CrossRef PubMed
.
- S. T. Wall, J. C. Walker, K. E. Healy, M. B. Ratcliffe and J. M. Guccione, Circulation, 2006, 114, 2627–2635 CrossRef PubMed
.
- J. L. Ifkovits, E. Tous, M. Minakawa, M. Morita, J. D. Robb, K. J. Koomalsingh, J. H. Gorman, R. C. Gorman and J. A. Burdick, Proc. Natl. Acad. Sci. U. S. A., 2010, 107, 11507–11512 CrossRef CAS PubMed
.
- M. Y. Chang, Y. J. Yang, C. H. Chang, A. C. L. Tang, W. Y. Liao, F. Y. Cheng, C. S. Yeh, J. J. Lai, P. S. Stayton and P. C. H. Hsieh, J. Controlled Release, 2013, 170, 287–294 CrossRef CAS PubMed
.
- Y. Zhang, R. E. Sievers, M. Prasad, R. Mirsky, H. Shih, M. L. Wong, F. S. Angeli, J. Ye, J. Takagawa, J. W. Koskenvuo, M. L. Springer, W. Grossman, A. J. Boyle and Y. Yeghiazarians, Cardiovasc. Pathol., 2011, 20, 204–212 CrossRef PubMed
.
- Y. C. Toh, S. Ng, Y. M. Khong, X. Zhang, Y. Zhu, P. C. Lin, C. M. Te, W. Sun and H. Yu, Nano Today, 2006, 1, 34–43 CrossRef
.
- H. Takahashi, M. Nakayama, T. Shimizu, M. Yamato and T. Okano, Biomaterials, 2011, 32, 8830–8838 CrossRef CAS PubMed
.
- H. N. Kim, A. Jiao, N. S. Hwang, M. S. Kim, D. H. Kang, D. H. Kim and K. Y. Suh, Adv. Drug Delivery Rev., 2013, 65, 536–558 CrossRef CAS PubMed
.
- T. Nakamura, S. Mizuno, K. Matsumoto, Y. Sawa, H. Matsuda and T. Nakamura, J. Clin. Invest., 2000, 106, 1511–1519 CrossRef CAS PubMed
.
- Y. Li, G. Takemura, K. I. Kosai, K. Yuge, S. Nagano, M. Esaki, K. Goto, T. Takahashi, K. Hayakawa, M. Koda, Y. Kawase, R. Maruyama, H. Okada, S. Minatoguchi, H. Mizuguchi, T. Fujiwara and H. Fujiwara, Circulation, 2003, 107, 2499–2506 CrossRef CAS PubMed
.
- X. Hu, S. Dai, W. J. Wu, W. Tan, X. Zhu, J. Mu, Y. Guo, R. Bolli and G. Rokosh, Circulation, 2007, 116, 654–663 CrossRef CAS PubMed
.
- W. Zhao, J. R. Waggoner, Z. G. Zhang, C. K. Lam, P. Han, J. Qian, P. M. Schroder, B. Mitton, A. Kontrogianni-Konstantopoulos, S. L. Robia and E. G. Kranias, Proc. Natl. Acad. Sci. U. S. A., 2009, 106, 20776–20781 CrossRef CAS PubMed
.
- J. Zhang, L. Ding, Y. Zhao, W. Sun, B. Chen, H. Lin, X. Wang, L. Zhang, B. Xu and J. Dai, Circulation, 2009, 119, 1776–1784 CrossRef CAS PubMed
.
- C. K. Lam, W. Zhao, W. Cai, E. Vafiadaki, S. M. Florea, X. Ren, Y. Liu, N. Robbins, Z. Zhang, X. Zhou, M. Jiang, J. Rubinstein, W. K. Jones and E. G. Kranias, Circ. Res., 2013, 112, 79–89 CrossRef CAS PubMed
.
- J. M. Gimble, B. A. Bunnell, E. S. Chiu and F. Guilak, Stem Cells, 2011, 29, 749–754 CrossRef PubMed
.
- E. Macia and P. A. Boyden, Circulation, 2009, 119, 1814–1823 CrossRef PubMed
.
- E. N. Simantirakis, E. P. Koutalas and P. E. Vardas, Europace, 2012, 14, 466–473 CrossRef PubMed
.
- S. M. Wu and K. Hochedlinger, Nat. Cell Biol., 2011, 13, 497–505 CrossRef CAS PubMed
.
- Y. Haraguchi, T. Shimizu, T. Sasagawa, H. Sekine, K. Sakaguchi, T. Kikuchi, W. Sekine, S. Sekiya, M. Yamato, M. Umezu and T. Okano, Nat. Protoc., 2012, 7, 850–858 CrossRef CAS PubMed
.
Footnote |
† Electronic supplementary information (ESI) available. See DOI: 10.1039/c3bm60289c |
|
This journal is © The Royal Society of Chemistry 2014 |