DOI:
10.1039/C3BM60277J
(Paper)
Biomater. Sci., 2014,
2, 784-797
Molecular farming of fluorescent virus-based nanoparticles for optical imaging in plants, human cells and mouse models†
Received
8th November 2013
, Accepted 26th February 2014
First published on 12th March 2014
Abstract
The application of plant virus-derived nanostructures in materials science, biomedical research and engineering has recently been promoted by the development of fluorescence-labeled viruses for optical imaging in tissue culture and preclinical animal models. Most studies reported thus far have focused on the application of viruses that have been chemically modified with organic dyes. In this investigation, we sought to develop and study genetically-engineered virus-based biomaterials that incorporate green or red fluorescent proteins. The genetic introduction of such imaging moieties is advantageous because post-harvest modifications are not required, thus minimizing the number of manufacturing steps and maximizing the yields of each fluorescent probe. Specifically, we engineered the filamentous plant virus Potato virus X (PVX) to display green fluorescent protein (GFP) or mCherry as N-terminal coat protein (CP) fusions, producing a 1
:
3 fusion protein to CP ratio. The infection of Nicotiana benthamiana plants with the recombinant GFP-PVX and mCherry-PVX particles was documented by fluorescence imaging, structural analysis and genetic characterization to determine the stability of the chimeras and optimize the molecular farming protocols. We also demonstrated the application of fluorescent mCherry-PVX filaments as probes for optical imaging in human cancer cells and a preclinical mouse model. Cell viability assays and histological analysis following the administration of mCherry-PVX indicated the biocompatibility and rapid tissue clearance of the particles. Such particles could therefore be functionalized with additional cancer-specific detection ligands to provide tools for molecular imaging, allowing the investigation of molecular signatures, disease progression/recurrence and the efficacy of novel therapies.
Introduction
Viruses were discovered in the early 1900s and have been studied since then by biologists and pathologists for insight into their infectious and pathogenic properties, and by chemists and engineers interested in the development of self-assembling nanostructured materials that provide a three-dimensional scaffold for the controlled arrangement of functional molecules. For example, the versatility of functionalized hybrid virus-based materials in the energy sciences has been recognized and several hybrid materials have been developed and tested for applications such as light harvesting,1–3 plasmonic metamaterial design,4 and energy and data storage.5–8 In the medical sector, virus-based materials are used to develop gene therapies, vaccines, and oncolytic virotherapies.9–11 More recently, virus-based scaffolds have been engineered for applications in molecular imaging and drug delivery.12,13
All types of viruses have been investigated, including mammalian viruses, bacteriophages, and plant viruses. We have focused on plant viruses for applications in materials science and medicine. Viruses are attractive as scaffolds because structure-based engineering using genetic and chemical modification procedures allows the introduction of deterministic, precise, and reproducible modifications. This level of quality control cannot yet be achieved with synthetic nanoparticles. The production of plant viruses by molecular farming in plants is highly scalable and economical, and does not involve toxic solvents or high-temperature processes.14–16 Plant virus-based technologies therefore have the potential to make a broad and global impact on medicine because they can be deployed ‘in the region for the region’ in developing countries lacking a conventional refrigeration system and healthcare infrastructure. Plant virus-based materials are biocompatible and biodegradable, and can be delivered intravenously or orally as purified nanoparticles or in edible plant tissues.15,17 Plant viruses do not replicate or infect mammalian cells and are therefore considered safe from a human health perspective. Billions of plant viruses are consumed by humans every day as part of the food chain.
In recent years, we and others have developed plant viruses labeled with fluorescent tags as molecular probes for optical imaging.18,19 For example, we demonstrated tumor-specific imaging using virus-based nanoparticles chemically tailored with peptide ligands.20,21 We and others have engineered viruses to target a variety of cancer-related cell-surface molecules, including transferrin, integrins, vascular endothelial growth factor (VEGF) receptors, and gastrin-releasing peptide receptors.21–24
Although most studies reported thus far have focused on the application of viruses that have been chemically modified with organic dyes, we sought to develop and study genetically-engineered virus-based biomaterials that incorporate green or red fluorescent proteins. This is advantageous because post-harvest modifications are not required, thus minimizing the number of manufacturing steps and maximizing yields.
Specifically, we set out to engineer Potato virus X (PVX) as a tool for optical imaging. Recent data from our laboratory indicate that the flexible filaments formed by PVX accumulate in tumors in preclinical animal models25,26 and that such passive targeting is based on the enhanced permeability and retention effect.27 The shape-mediated tumor homing agrees with earlier studies showing that elongated high-aspect-ratio (length × width) materials have favorable margination, flow and penetration properties, thus enhancing their accumulation in tumors.28–34 Furthermore, the reduced uptake of high-aspect-ratio materials into mononuclear phagocytotic cells synergistically contributes to the shape-mediated in vivo properties.35,36 Therefore, we reasoned that PVX would be a suitable platform for the development of optical imaging probes.
PVX is the type member of the Potexvirus group and forms filamentous particles (515 × 13 nm) comprising 1270 identical 25 kDa coat protein (CP) subunits and a monopartite positive-sense single-stranded RNA genome.37 Each CP contains at least one solvent-exposed lysine residue available for chemical modification.38 The X-ray structure of PVX has not been reported, so the location of the chemically-addressable lysine side chain within the CP remains elusive. It is thought that the N-terminal part of the CP is exposed on the surface, so in most cases the fusion partner is joined to the CP N-terminus.39,40
Here we report the genetic engineering of PVX to display green fluorescent protein (GFP) or red fluorescent mCherry protein as N-terminal CP fusions. We also describe the application of these structures as optical probes for imaging in cells, as well as animal and plant tissues. Although the preparation of fluorescent PVX ‘overcoat’ particles in plants has been achieved using similar strategies,41–45 the use of such probes in optical imaging is a novel application. Fluorescence-labeled PVX was produced by molecular farming in Nicotiana benthamiana plants. Time-course studies were carried out to monitor the genetic stability of the chimeras, and to optimize the molecular farming protocols. We demonstrate the application of genetically-engineered, fluorescent PVX particles as probes for optical imaging in human cells and mouse models.
Experimental
Genetic engineering of GFP-PVX and mCherry-PVX
Vector pTCXI was constructed by cloning the full-length PVX genome including the GFP-2A-CP fusion sequence from pCX145 into the pTRAc.46 The mCherry construct was created by replacing the GFP sequence in vector pTCXI with the mCherry sequence from plasmid pmCherry-C1. The original pTCXI vector contained the full-length PVX genome including the GFP-2A-CP fusion sequence, and the replacement was made by excising the GFP sequence with restriction enzymes NheI and BspEI and inserting the mCherry sequence (Clontech, Mountain View, CA, USA).47 The resulting construct, containing the mCherry-2A-CP fusion sequence, was named pTCXIIc (Fig. 1).
 |
| Fig. 1 (a) Schematic representation of the pCX1 and pTCXIIc expression vectors, containing the full-length genome of PVX under the control of the Cauliflower mosaic virus (CaMV) 35S promoter: RdRp = RNA-dependent RNA polymerase; p25, p12 and p8 form the PVX triple gene block, encoding proteins involved in movement; 2A encodes the 2A sequence NFDLLKLAGDVESNPG from Foot and mouth disease virus (FMDV), which induces a ribosomal skip leading to the production of fusion GFP/mCherry-CP and free proteins; CP = coat protein. (b) Schematic representation of GFP-PVX and mCherry-PVX. The filamentous nanoparticle is assembled from CP and fusion proteins and encapsidates a positive-sense single-stranded RNA (+ssRNA) genome. | |
Propagation of PVX in plants and purification
The recombinant vectors were amplified in Escherichia coli strain DH5α and purified using the Qiagen Midi Kit (Qiagen, Hilden, Germany). N. benthamiana plants were mechanically inoculated using purified pCXI and pTCXIIc DNA (5–10 μg per leaf) mixed with carborundum. Systemically infected leaves were harvested 14–21 days post-inoculation (dpi) and stored at −80 °C. Recombinant PVX was purified from the infected leaves as previously described.48
Expression and purification of mCherry
The mCherry protein was transiently expressed in N. benthamiana following agroinfiltration with Agrobacterium tumefaciens strain GV3101 carrying vector pTRAkc-ERH-mCherry-his6, in which mCherry is under control of the 35S promoter46 and is expressed with C-terminal His6 and KDEL tags, the latter directing the protein to accumulate in the endoplasmic reticulum. Plant material was harvested at 3 dpi and the mCherry was purified by immobilized metal ion affinity chromatography (IMAC). The plant material was ground in two volumes of phosphate-buffered saline (PBS) and centrifuged for 15 min at 4 °C and 13
000 rpm. The supernatant was diluted 1
:
10 with PBS and applied to Ni-NTA agarose columns prepared according to the manufacturer's instructions (Qiagen). The columns were washed with 10 mM imidazole in PBS and the mCherry was eluted with 150 mM imidazole in PBS. The eluate was dialyzed against 0.01 M phosphate buffer (pH 7.2) and concentrated with Vivaspin 6 columns (Sartorius). Purified mCherry was characterized by polyacrylamide gel electrophoresis (ESI Fig. S2†).
UV/visible spectroscopy
The concentration of PVX chimeras was determined by UV/visible spectroscopy. The extinction coefficient of PVX is 2.97 cm−1 mg−1 mL (at 260 nm). The samples were considered to be pure if the A260
:
280 ratio was 1.2 ± 0.1.
Transmission electron microscopy
The particles were either bound directly to adsorption grids for standard transmission electron microscopy (TEM), or processed for immunosorbent transmission electron microscopy (ISEM). For standard TEM, 10 μg of purified PVX particles was incubated on Pioloform-coated nickel grids (400 mesh grids, Plano GmbH, Wetzlar, Germany) for 20 min. For ISEM, nickel grids were coated for 20 min with either α-PVX (DSMZ, Braunschweig, Germany), α-mCherry (DsRed antibody, GeneTex, Irvine, CA, USA) or α-GFP antibodies (GeneTex, Irvine, CA, USA) diluted 1
:
10 in PBS. Excess antibody was washed off with PBS plus 0.2% (w/v) Tween 20 (PBST) and the grids were blocked with 0.5% (v/v) bovine serum albumin (BSA) in PBS for 15 min. The grids were then incubated with 10 μg of purified PVX particles for 20 min, followed by washing with PBS. For immunogold staining of the absorbed or captured PVX particles, the grids were incubated for 2 h with the α-PVX, α-mCherry or α-GFP antibodies diluted 1
:
100 in PBS. Bound antibodies were detected by incubating the grids overnight with goat-anti-rabbit secondary antibodies labeled with 15 nm gold particles diluted 1
:
50 in PBS. The grids were then washed thoroughly with distilled water and counterstained with 1% (w/v) uranyl acetate (pH 4.3) before analysis with a Zeiss EM 10 TEM (Oberkochen, Germany).
Gel electrophoresis and western blotting
Sodium dodecylsulfate polyacrylamide gel electrophoresis (SDS-PAGE) was carried out as described49 using a stacking gel (T = 4%, C = 2.7%, pH 6.8) and a resolving gel (T = 12%, C = 2.7%, pH 8.8). Samples were mixed with loading dye (35 mM Tris-HCl, pH 6.8; 60 mM dithiothreitol (DTT); 10.3% (w/v) SDS; 0.05% (w/v) bromophenol blue; 31% (w/v) glycerol or 62.5 mM Tris-HCl pH 6.8, 30% (w/v) glycerol, 4% (w/v) SDS, 10% (v/v) 2-mercaptoethanol, 0.05% (w/v) bromophenol blue) and denatured at 100 °C. Samples containing GFP or mCherry were not heated to 100 °C if required for in-gel fluorescence visualization, to prevent chromophore damage.50 After electrophoresis, proteins were imaged under UV light and also under white light following staining with Coomassie Brilliant Blue.51 Alternatively, proteins were transferred onto nitrocellulose membranes (HybondC, Amersham) using a Mini Protean II transblot cell (BioRad) and incubated with the α-PVX, α-mCherry or α-GFP antibodies. These were detected with alkaline phosphatase-labeled anti-rabbit polyclonal secondary antibodies and NBT/BCIP (Sigma Aldrich).
Reverse transcriptase PCR and sequencing
Infected leaves were harvested and PVX particles were immunocaptured by coating 0.5 mL tubes with anti-PVX antibodies and filling with crude extracts from infected leaves.52 Virus ssRNA was extracted with phenol–chloroform–isoamylalcohol and used for cDNA synthesis with the ProtoScript™ first strand cDNA synthesis kit (New England Biolabs). Alternatively, reverse transcription was carried out using M-MLV Reverse Transcriptase RNaseH Minus Point Mutant (Promega, Madison, USA). In each case, 1 μl of 10 μM primer CX1 (5′-TTG AAG AAG TCG AAT GCA GC-3′) was used with Taq polymerase (Promega, Madison, USA) and primers CX2 (5′-CTA GAT GCA GAA ACC ATA AG-3′) and CX4 (5′-CGG GCT GTA CTA AAG AAA TC-3′), which bind upstream and downstream of the GFP/mCherry open reading frames. DNA sequencing was carried out using a Prism 3700 device (Perkin-Elmer).
Agarose gel electrophoresis of PCR products
RT-PCR products were analyzed by 1.2% agarose gel electrophoresis in 1× TBE buffer (45 mM Tris, 45 mM boric acid, 1.25 mM ethylenediaminetetraacetic acid (EDTA) in MilliQ water). Ethidium bromide was used for gel staining and the gels were photographed under UV light.
PVX biodistribution in mice
All experiments were carried out according to the Case Western Reserve University's Institutional Animal Care and Use Committee approved protocols. For biodistribution studies, 6-week-old C57BL/6 mice were maintained on an alfalfa-free rodent diet (Teklad-2018S) for 2 weeks prior to the administration of mCherry-PVX particles, to reduce tissue autofluorescence. We administered 10 mg kg−1 mCherry-PVX in 100 μL sterile PBS or PBS alone as a control by intravenous tail vein injection using a 1 mL 28-gauge insulin syringe. For tumor-homing studies, 6-week-old male NCr nu/nu nude mice maintained on an alfalfa-free diet as above were subcutaneously injected in the flank with 2.5 × 106 HT-29 cells in 50 μL medium mixed with an equal volume of matrigel (BD Matrigel, BD Biosciences, USA) using a microliter Hamilton 22-gauge syringe. The animals were monitored daily and tumor homing studies commenced when tumors reached an average volume of 20 mm3 (typically within 12 days after the injection of HT-29 cells). We administered 10 mg kg−1 mCherry-PVX and 0.25 mg kg−1 free mCherry, therefore keeping the mCherry dose constant between the two groups. The mCherry-PVX and free mCherry protein were administered in 100 μL sterile PBS by intravenous tail vein injection using a 1 mL 28-gauge insulin syringe. For Maestro imaging, mice were sacrificed 12 h, 24 h and 7 days after injection. Major tissues were removed and analyzed using the Maestro fluorescence imager fitted with green excitation and emission filters (800 ms exposure). The relative fluorescence intensity was determined using ImageJ v1.44o software (http://imagej.nih.gov/ij). Tissues were then frozen in OCT medium (Tissue-Tek, Sakura Finetek, USA) and stored at −80 °C for immunofluorescence analysis (see below).
Immunofluorescence microscopy of frozen tissues
Embedded organs were used to prepare 10 μm sections on a Leica CM 1850 cryostat. Frozen liver tissue sections were fixed in ice-cold 95% (v/v) ethanol for 20 min and washed three times with cold PBS. Fixed tissues were then permeabilized using 0.2% (v/v) Triton-X in PBS for 2 min and washed again with PBS followed by blocking with 10% (v/v) goat serum for 1 h. Macrophages in the liver were stained using rat anti-mouse F4/80 (Biolegend) diluted 1
:
250 with 1% (v/v) goat serum, then Alexa488-labeled goat anti-rat IgG (Life Technologies) diluted 1
:
500 with 1% (v/v) goat serum. Frozen tumor sections were stained with an FITC-labeled anti-mouse CD31 antibody (BioLegend) to visualize the endothelium. Each step was followed by multiple washes with cold PBS. Slides were mounted using Fluorshield with DAPI mounting medium (Sigma) for nuclear staining, sealed using nail polish and stored at −20 °C. Fluorescence imaging was carried out using a Zeiss Axio Observer Z1 motorized FL inverted microscope, and confocal images were captured using a Fluoview FV1000 LSCM (Olympus).
Hematoxylin and eosin histology
The frozen sections were fixed with 95% (v/v) ethanol for 5 min at −20 °C then air-dried. After washing with deionized water, the slides were stained with hematoxylin (Richard-Allen Scientific) for 45 s. The tissues were washed sequentially with 70%, 80% and 100% (v/v) ethanol each for 3 min, then stained with eosin for 1 min. The tissues were mounted and analyzed using a Zeiss Axio Observer Z1 motorized FL inverted microscope.
Cell imaging
HT-29 cells (ATCC) were maintained on McCoy's5A medium supplemented with 10% (v/v) fetal bovine serum (FBS) and 1% (v/v) penicillin/streptomycin (Life Technologies) at 37 °C, 5% CO2. For uptake studies, 25
000 HT-29 cells per well were cultivated for 24 h on glass coverslips placed in an untreated 24-well plate in 250 μL culture medium at 37 °C, 5% CO2. Cells were washed and mCherry-PVX (5 μg per well) was introduced in 100 μL of the corresponding medium, incubated for 3 h, and then washed with saline to remove any unbound particles. After 3 h further incubation, cells were fixed for 5 min at room temperature using PBS containing 4% (v/v) paraformaldehyde and 0.3% (v/v) glutaraldehyde. Cell membranes were stained using 1 μg mL−1 wheat germ agglutinin conjugated with AlexaFluor 647 (Life Technologies) in 5% (v/v) goat serum (Life Technologies) for 45 min at room temperature in the dark followed by washing with DPBS. Coverslips were then mounted onto glass slides using Fluorshield with DAPI mounting medium (Sigma) for nuclear staining and sealed using nail polish. Confocal images were captured on an Olympus FluoView FV1000 LSCM and the data were processed using Image J v1.44o software.
Cell viability assay
Cell viability was determined using the XTT Cell Proliferation Assay Kit (ATCC). HT-29 cells were seeded onto 96-well plates (25
000 cells per well; 100 μL McCoy's medium per well), incubated for 24 h at 37 °C, 5% CO2, washed twice, and then incubated in triplicates with 100 μL McCoy's medium containing 1 μg, 10 μg or 30 μg mCherry-PVX and similar amounts of PVX for 3 h. The cells were then washed three times and incubated for an additional 24 h with 100 μL of fresh medium at 37 °C, 5% CO2. Each well was then supplemented with 50 μL of XTT reagent and the plates were incubated for another 2–3 h for color development. Absorbance was recorded at 450 and 650 nm on TECAN Infinite® 200 PRO multimode plate reader, followed by data analysis as recommended by the supplier. All assays were carried out at least twice in triplicate, and the data were analyzed using Microsoft Excel software.
Results and discussion
Systemic infection of N. benthamiana plants with PVX chimeras
PVX infects more than 240 plant species in 16 families, predominantly the Solanaceae (nightshades) (dpvweb.net). N. benthamiana plants are susceptible to PVX infection by mechanical inoculation or agroinfiltration using infectious plasmids or purified PVX particles. N. benthamiana was therefore chosen as the production host for fluorescence-tagged PVX particles: GFP-PVX and mCherry-PVX.
To optimize the yields of fluorescent PVX particles, we conducted time-course studies and documented the systemic infection process under white, UV, and green light (Fig. 2 and ESI Fig. S1†). Plants were infected with pCX1 (encoding GFP-PVX) or pTCXIIc (encoding mCherry-PVX) by mechanical inoculation 3–4 weeks after seeding. Photographs were taken every second or third day post inoculation (dpi) over a 3-month time frame. In parallel, we assessed the stability of the PVX chimeras using a combination of gel electrophoresis and western blotting (protein stability) and RT-PCR/cDNA sequencing (genomic stability).
 |
| Fig. 2 Time course of the infection of N. benthamiana with GFP-PVX (dpi = days post inoculation). Pictures were taken under daylight (left) and under UV light (right). Pink arrows indicate the inoculated leaves. The green arrow at 8 dpi shows the first fluorescent signals in the sink leaves, indicating a systemic infection. All studies were conducted at least twice in triplicate. Representative photographs are shown. | |
At early time points (6–10 dpi), we observed cell-to-cell movement. An infected zone was indicated by the appearance of green fluorescence, slowly and radially spreading on the inoculated leaves (Fig. 3). A transition to long-distance movement leading to systemic infection occurred as soon as the infected zone reached the veins (12–16 dpi), enabling the transport of PVX via the vascular bundles. Systemic infection was established between 14 and 21 dpi (Fig. 2 and 3, and ESI Fig. S1†). At later time points (28 dpi onwards), the infection continued to spread from source to sink leaves (‘source’ being defined as photosynthetically-active leaves and ‘sink’ being defined as developing leaves on the shoot of the growing plant), as shown by chlorotic and mottled sink leaves (Fig. 2, images under white light). However, the loss of green fluorescence indicated that the corresponding gene sequence was lost after 28 dpi (Fig. 2). Loss of function was also confirmed at the protein and genetic levels (see below). Overall, the time course GFP-PVX and mCherry-PVX infections were similar, with the greatest yields of chimeric particles between 14 and 21 dpi (Fig. 2 and 3, and ESI Fig. S1†).
 |
| Fig. 3 Infection process over time. In the inoculated leaves (left panel), GFP-PVX spreads via cell-to-cell movement (6 dpi). Transition to long-distance movement occurs when the particles reach a vein (10 dpi), leading to systemic infection (16 dpi). The GFP-PVX particles travel to the roots (12 dpi) prior to establishing a systemic infection (16 dpi). The green star in the middle panel indicates the stem, showing fluorescence at 16 dpi but not at 12 dpi. Middle and right panels show an infected plant at 12 and 16 dpi. Arrows (right panel) indicate the position of the inoculated leaf. A magnified image of the same plant is shown in the middle panel, rotated 90 degrees clockwise. Pictures were taken under UV light. | |
The systemic infection with fluorescent PVX chimeras was consistent with previous reports.41,43–45 Following DNA transfer through the lesions created by mechanical inoculation, virus replication, protein synthesis, assembly and movement takes place. First, we observed cell-to-cell movement, then long-distance movement. Using fluorescence-labeled PVX, others have recently proposed a coreplicational insertion model, in which the movement proteins function to compartmentalize replication complexes at the plasmodesmata for localized RNA synthesis and directional trafficking of the virus between cells.42 PVX mobilizes as filamentous virus-like particles assembled from RNA and CPs, defined as RNP particles, that travel from cell to cell through the plasmodesmata, whereas long-distance transport involves passive source-to-sink transport through the phloem.41,53
Close-up images of infected leaves, petioles and stems indicated that GFP-PVX travels to the roots before establishing a systemic infection (Fig. 3 and 4). At 12 dpi, green fluorescence was apparent in the vein, petiole, and along the stem, moving down toward the roots. Fluorescence was not apparent in the stem region above the petiole of the source leaf (Fig. 3). In contrast, at 16 dpi, fluorescence signals were observed traveling both up and down the stem from the petiole of the source leaf, and this was consistent with the establishment of a systemic infection, as indicated by green fluorescence in the sink leaves (Fig. 3). The analysis of hydroponic N. benthamiana plants confirmed that PVX spreads into the roots (Fig. 4).
 |
| Fig. 4 Hydroponic cultures of N. benthamiana under UV light 21 days after mechanical inoculation with pCXI. Left panel: Root and stem of a plant infected with GFP-PVX, showing fluorescence in the roots. Middle panel: GFP-PVX-infected plant (left) and non-infected plant (right). Right panel: Roots from a non-infected plant. | |
Transport in the phloem occurs via the sieve elements from source to sink. In N. benthamiana, PVX enters the sieve elements via apoplastic phloem loading.54 Based on the Münch mass flow hypothesis,55 transport within the phloem reflects osmotic pressure due to the increased solute concentrations in source tissues (high osmotic potential Ψπ) versus sink tissues (low Ψπ). Our observation that PVX travels to the roots prior to systemic trafficking to the sink leaves is consistent with the osmotic potential following the trend Ψπ source leaves ≫ sink leaves (shoot) > roots.
Stability of PVX chimeras in plants over time
Photographic documentation of infected plants over time indicated that although sink leaves continue to be infected with PVX after 28 dpi, the inserted gene may be lost or mutated because the leaves show the characteristic symptoms of PVX infection but lack green fluorescence (Fig. 2). Furthermore, we noted that the fluorescence of the infected leaves decreased over time following a peak of intensity at 14–21 dpi and a peak infection area at 18–25 dpi (ESI Fig. S3†). Image analysis using ImageJ software indicated that fluorescence intensity decreased to 70% of the maximum during later stages (ESI Fig. S4†). The steady expansion of the infected zone can be explained by continued cell-to-cell movement after phloem unloading. The reduced fluorescence intensity observed over time may reflect the genetic instability of the constructs (see Discussion below) or the structural instability of the chimeras in leaves over time. Changing leaf physiology and senescence may also contribute the observed effect, because chloroplasts are converted to gerontoplasts leading to leaf yellowing which reduces the contrast with GFP.56
Particle stability at the protein level was determined by TEM and biochemical analysis (see below). TEM imaging confirmed the structural integrity of the PVX particles and the presence of GFP or mCherry fusion proteins. Immunosorbent transmission electron microscopy (ISEM) was carried out either by capturing the particles using α-GFP or α-mCherry antibodies followed by staining with an α-PVX antibody or vice versa (Fig. 5). In all cases, elongated filaments were observed, indicating structural integrity. Wild-type PVX (PVX 0018) was generally not captured by the α-GFP or α-mCherry antibodies (although we observed low to negligible non-specific absorption probably reflecting imperfect blocking with BSA, resulting in direct binding to the TEM grids as shown in Fig. 5D and G). We confirmed the specific capture of GFP-PVX and mCherry-PVX by α-GFP and α-mCherry, respectively (Fig. 5E and F, cross-reactivity of the antibodies was not apparent, see ESI Fig. S5†). Furthermore, the capture of GFP-PVX and mCherry-PVX particles by α-PVX followed by staining with the α-GFP or α-mCherry antibodies confirmed the high-density clustering of recombinant proteins along the GFP-PVX and mCherry-PVX particle surfaces (Fig. 5H and I).
 |
| Fig. 5 TEM imaging of wild-type PVX (PVX 0018), GFP-PVX, and mCherry-PVX. (A–C) Adsorption grids with A = PVX 0018, B = GFP-PVX (pCXI), C = mCherry-PVX (pTCXIIc). (D–I) ISEM grids with D = PVX 0018 captured with α-GFP, detected with α-PVX, E = GFP-PVX captured with α-GFP, detected with α-PVX, F = mCherry-PVX captured with α-mCherry, detected with α-PVX, G = PVX0018 captured with α-mCherry, detected with α-PVX, H = GFP-PVX captured with α-PVX, detected with α-GFP, I = mCherry-PVX captured with α-PVX, detected with α-mCherry. Scale bars = 500 nm. Further controls are shown in the ESI, see Fig. S5.† | |
Denaturing gel electrophoresis and western blot analysis confirmed the presence of the PVX CP as well as the fusion proteins GFP-2A-CP and mCherry-2A-CP (Fig. 6A–C). Time-course studies involving the analysis of non-fluorescent but symptomatic leaves as well as fluorescent, symptomatic leaves harvested at 20, 42 and 65 dpi confirmed the presence of the PVX CP (25 kDa) in all leaves showing symptoms, whereas the GFP-2A-CP fusion protein (54 kDa) was detected only in fluorescent, symptomatic leaves. Lane analysis using ImageJ software indicated that the GFP-2A-CP
:
free CP ratio was 1
:
3 at 20 dpi, 1
:
7 at 42 dpi, and 1
:
10 at 65 dpi (Fig. 6A) thus confirming the instability over time discussed above. The expected ratio for GFP-2A-CP
:
free CP is 1
:
3 based on the intervening 2A sequence.58–62
 |
| Fig. 6 Biochemical analysis of chimeric, fluorescent PVX constructs. (A) Western blots of plant sap probed with α-PVX and α-GFP antibodies. 1 = N. benthamiana, non-infected plant; 2 = non-symptomatic, non-fluorescent leaves from an infected plant at 65 dpi; 3 = symptomatic, non-fluorescent leaves from the shoot of an infected plant at 65 dpi, 4 = purified wild-type PVX0018 (positive control, 25 kDa), 5 = GFP (positive control, 27 kDa); 6 marker (NEB), molecular weight indicated; 7–9 = symptomatic, fluorescent leaves from an infected plant at 20, 42, and 65 dpi. Note: since plant sap is studied here, both GFP-CP fusion (54 kDa) as well as free GFP are detected in the western blots. (B) Western blot of plant sap with different fluorescent protein-2A-CP fusions at 21 dpi, probed with α-PVX (top panel) and visualization of fluorescence under UV light (bottom panel). 1 = ColorPlus Prestained Protein Ladder (NEB), 2 = pTCXI (GFP-2A-CP), 3 = pTCXII (DsRed-2A-CP), 4 = pTCXIIb (mBanana-2A-CP), 5 = pTCXIIc (mCherry-2A-CP), 6 = N. benthamiana sap (non-infected), 7 = pTCXV-mCherry (mCherry control, 26.7 kDa), 8 = PVX0018. (C) SDS-PAGE (left panel) and Western blots of 1 = ColorPlus Prestained Protein Ladder (NEB), 2 = PVX (control), 3 = purified mCherry-PVX, and stained with Coomassie or α-PVX (middle panel) and α-mCherry antibodies (right panel). (D) SDS-PAGE of 1 = ColorPlus Prestained Protein Ladder (NEB), 2 = purified mCherry-PVX, and 3 = PVX (control) visualized by staining with Coomassie Brilliant blue and direct green light illumination with a red filter. (E) Agarose gel electrophoresis of amplified cDNA after RT-PCR. 1 = N. benthamiana, non-infected plant; 2 = marker, lambda DNA digested with PstI, 3–5 = symptomatic, fluorescent leaves from a GFP-PVX infected plant at 20, 42, and 65 dpi. (F) Agarose gel electrophoresis of amplified cDNA after RT-PCR, at 21 dpi, 1 and 11 = GeneRuler™ 100 Plus DNA Ladder (Fermentas), 2 = N. benthamiana non infected, 3 = plant infected with PVX0018 (expected band 216 bp), 4 = plant infected with pTCXI, 5 = empty, 6 = plant infected with pTCXIIc, 7 = PCR negative control (no template), 8 = empty PVX cloning vector (305 bp), 9 = plasmid pTCXI (1011 bp), 10 = plasmid pTCXIIc (1009 bp). | |
Long-term instability of the GFP-PVX constructs was confirmed at the genetic level by RT-PCR analysis, gel electrophoresis and sequencing. The full-length GFP-2A-CP construct (1011 bp) was detectable at 20 dpi, but at later time points (42 and 65 dpi) the insert was truncated and then lost (Fig. 6E). These data were consistent with sequencing analysis, indicating a loss of function over time. The genetic instability of viral vectors is common because they are prone to heterologous or homologous recombination events that partially or completely delete the nonessential transgene insert.57
The mCherry-PVX particles were extracted from leaves harvested at 14–21 dpi, and gel electrophoresis followed by western blot analysis revealed an mCherry-2A-CP
:
free CP ratio of 1
:
3 (Fig. 6C and D) as anticipated based on the intervening 2A sequence.58–62 The resulting ribosomal skip produces the fusion protein as well as free CP, the latter being essential for virus assembly and systemic infection.45,63,64
The systemic infection in plants was monitored over time, revealing that systemic infection occurred more rapidly in plants infected with mCherry-PVX than in those infected with GFP-PVX. The further analysis of genomic and protein stability also indicated that mCherry-PVX particles were less prone to lose the transgene over time and that mCherry fluorescence was more robust, i.e. less susceptible to bleaching (Fig. 6B–D and F and ESI Fig. S1†). We also constructed plasmids encoding DsRed and mBanana CP fusions (Fig. 6B). However, we decided to proceed with mCherry-PVX alone because this construct produced the most stable particles at the genomic and protein levels, and the highest particle yields at 21 dpi. The DsRed fusion is only fluorescent as tetramer and is therefore not suitable for display on the surface of PVX. Another option for future consideration is the mBanana-fusion, which achieved similar yields to mCherry-PVX.
In summary, our imaging and biochemical analysis of plants and purified PVX particles indicated that infected leaves should be harvested at 14–21 dpi to achieve the maximum yields of chimeric, fluorescent PVX particles. In this window, the greatest number of leaves was infected, reaching a maximum area per leaf and maximum fluorescence intensity. To limit the biomass that needs to be processed, only the fluorescent leaves should be harvested.
Biodistribution and clearance of mCherry-PVX in healthy mice
We produced and purified mCherry-PVX particles using the optimized high-yield protocols referenced above. Intact mCherry-PVX particles were extracted from infected leaves at yields of 4 mg pure mCherry-PVX particle per 100 grams of infected leaf tissue. Particle integrity was confirmed by TEM imaging (Fig. 5), gel electrophoresis and western blotting (Fig. 6).
The organ biodistribution for mCherry-PVX was determined following the injection of 10 mg kg−1 mCherry-PVX into the tail veins of healthy C57BL/6 mice, which were sacrificed 24 h and 7 days post-administration. Following perfusion, we dissected the heart, liver, kidneys, spleen, lungs, and brain for fluorescence analysis using the Maestro imaging system (Fig. 7A). This revealed that mCherry-PVX is cleared via the reticuloendothelial system and deposited in the liver, resulting in tissue clearance by 7 days post-administration. Fluorescence imaging of liver sections indicated co-localization with macrophages (F4/80 marker, Fig. 7B). This is consistent with the clearance of proteinaceous nanoparticle formulations via the mononuclear phagocytotic system (MPS), specifically Kupffer cells in the liver.65
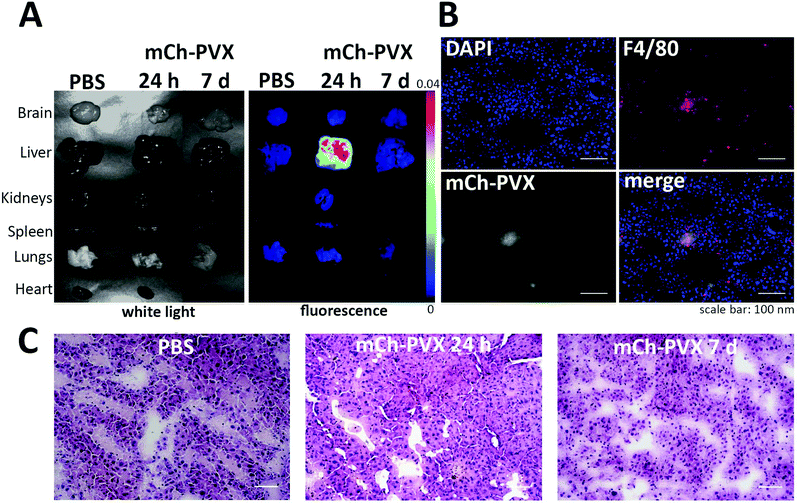 |
| Fig. 7 (A) Organ distribution of mCherry-PVX at 24 h and 7 days post administration in healthy C57BL/6 mice. (B) Fluorescence microscopy of cryosectioned liver tissue stained with DAPI (blue) and F4/80 antibody (red, macrophages). The mCherry-PVX particles are pseudocolored in green. (C) H&E staining of liver tissues 24 h and 7 days post administration of PVX. Scale bars = 100 nm. | |
Histological comparison of tissues from animals inoculated with mCherry-PVX (or PBS as a control) at 24 h and 7 days post-administration showed no evidence of pathological changes, and the tissue morphology was similar in both groups with no signs of overt toxicity or inflammation, such as tissue degeneration, apoptosis or necrosis (Fig. 7C).
Overall, the data indicate that mCherry-PVX nanoparticles follow typical biodistribution and clearance profiles in mice. Optical imaging and histology confirmed clearance from the circulation via the reticuloendothelial system, as indicated by deposition in the liver, followed by rapid tissue clearance. At 7 days post-administration, mCherry-PVX particles were no longer detectable in tissues, as confirmed by imaging based on the mCherry-label as well as immunostaining using PVX-specific antibodies (data not shown). The rapid hepatobiliary clearance of nanoparticles is important for clinical applications. In stark contrast, hard inorganic nanoparticles, such as single-walled carbon nanotubes (SWNTs), gold and silica, are secreted slowly following uptake by the liver and spleen, and it may take several months for such materials to be cleared from tissues completely.66 Persistence in tissues can induce toxic effects such as inflammation and apoptosis.67,68 In agreement with the rapid tissue clearance, H&E-stained liver tissue revealed no signs of overt toxicity or inflammation, such as tissue degeneration, apoptosis or necrosis, providing further evidence for the biocompatibility of the mCherry-PVX platform. However, more detailed organ-function assays will be needed to confirm the biocompatibility of PVX-based probes.
Imaging in human cells
To determine whether mCherry-PVX particles are taken up by human HT-29 (colon) tumor cells, in vitro cell cultures were analyzed by confocal microscopy after exposure to mCherry-PVX particles. This showed that mCherry-PVX nanoparticles were indeed taken up by the cells and localized in the perinuclear region (Fig. 8A). This is consistent with previous reports that PVX nanoparticles labeled with organic dyes are taken up by mammalian cells, including human cancer cells and mouse fibroblasts.38 There was no evidence of acute cell toxicity (Fig. 8B).
 |
| Fig. 8 (A) The uptake of mCherry-PVX (pseudocolored in green) into HT-29 cells stained with wheat germ agglutinin (cell membrane, red) and DAPI (nucleus, blue). Scale bar = 20 μm. (B) XTT cell viability assay, comparing HT-29 cells exposed to mCherry and wild-type PVX for 24 h at various concentrations. | |
Imaging of solid tumors using a preclinical mouse model
We have previously shown that PVX filaments carrying organic dyes accumulate within solid tumors in preclinical animal models.25,26 Here, we set out to determine whether mCherry-PVX also homes to solid tumors by comparing the biodistribution of mCherry-PVX versus free mCherry protein in nude mice with human HT-29 (colon) tumor xenografts (Fig. 9). Equivalent amounts of mCherry-PVX and purified mCherry proteins were administered by tail vein injection, the mice were sacrificed and imaging was carried out 12 h post injection. Equivalent amounts of both proteins accumulated in solid tumors, although the intra-tumoral distribution profiles were distinct (Fig. 9A). Immunofluorescence imaging and co-localization studies using frozen tumor tissue sections and a CD31 antibody indicated that mCherry co-localized with the tumor endothelium (M2 = 0.83) whereas mCherry-PVX extravasates into the tumor tissue (M2 = 0.07) (Fig. 9B). Such enhanced tissue penetration combined with functional versatility and the capacity for large payload delivery makes PVX an attractive platform for tumor imaging and drug delivery. We also observed differences in clearance behavior. Whole tissue imaging showed that free mCherry protein was present in the kidneys 12 h post-administration, whereas we have previously shown that PVX particles are cleared from healthy mice primarily via renal filtration and hepatobiliary clearance within 4 h post administration (Lee & Steinmetz, manuscript in preparation). The lack of a fluorescence signal in the kidneys of animals treated with mCherry-PVX particles may indicate that mCherry-PVX is cleared more rapidly from the kidneys than free mCherry protein. However, more detailed time course studies are required to characterize the clearance of mCherry-PVX versus free mCherry protein, and this is beyond the scope of the current report.
 |
| Fig. 9 (A) Biodistribution of mCherry-PVX versus purified mCherry protein in NCR nu/nu mice with HT-29 tumor xenografts 12 h post intravenous administration. Imaging was carried out using the Maestro imager. (B) Confocal images of tumor sections from mice injected with mCherry (a) and mCherry-PVX (c) stained with DAPI (blue) and FITC-labeled anti mouse CD31 antibody (pseudocolored red). Panels (b) and (d) are the corresponding mCherry and mCherry-PVX : CD31 colocalization signals. Scale bars in B = 50 μm. | |
In summary, based on passive tumor accumulation, biodistribution and the ability to enter mammalian cells, mCherry-PVX nanoparticles provide a novel platform for optical imaging in tissue culture and preclinical animal models.
Conclusions
We have engineered PVX to display fluorescent proteins (GFP or mCherry) as N-terminal CP fusions and have investigated their application as imaging probes. We systematically studied the infection process, documenting cell-to-cell and long-distance movement from source to sink tissues. The potential applications of fluorescence-tagged plant viruses and their proteins have been recognized, and GFP-tagged PVX has been widely used to study virus behavior in plants, e.g. in vivo imaging of GFP-tagged PVX to follow cell-to-cell movement, phloem loading and unloading showed that the CP is required for cell-to-cell movement.41–45,54
In our studies, we found that PVX spreads to the roots prior to the establishment of a systemic infection of the aerial parts of the plant, reflecting the Münch mass flow hypothesis in which there is a trend in osmotic potential: Ψπ source leaves ≫ sink leaves (shoot) > roots. To the best of our knowledge, this is the first report demonstrating that PVX spreads to the roots prior to distribution to the shoot.
Documentation of the infection process allowed us to optimize protocols to ensure the highest yields of recombinant GFP-PVX and mCherry-PVX. Genetic instability was apparent after 25 dpi. Therefore, harvest and purification should be carried out at 14–21 dpi, when systemic infection is established and the maximum area of infected leaf tissue is achieved but genetic and structural stability is maintained. However, it is beneficial to check each batch for the presence of the transgene and to verify fusion protein expression at the anticipated ratio (1
:
3 fusion protein to free CP).
We evaluated the suitability of fluorescence-labeled PVX particles as probes for optical imaging applications in tissue culture and preclinical animal models. The in vivo fate followed the trends observed previously for PVX particles labeled with organic dyes25,26 and was also in agreement with biodistribution profiles reported for other plant virus-based nanoparticles, including Cowpea mosaic virus,69,70Cowpea chlorotic mottle virus71 and Tobacco mosaic virus.72 Furthermore, we confirmed that mCherry-PVX homes to solid tumors in vivo (Fig. 9), which is consistent with previous studies showing that PVX filaments carrying organic dyes accumulate in tumors in preclinical animal models.25,26
The application of plant virus-based systems in the medical sector is attracting increasing interest. We propose the use of GFP-PVX and mCherry-PVX nanoparticles (as well as other color combinations) as optical imaging probes. Optical imaging has a high sensitivity and spatial resolution, avoids exposure to radiation, and allows multi-color imaging using spectrally-resolved probes (i.e. fluorophores with distinct emission wavelengths such as GFP and mCherry). Optical imaging has the potential not only to detect tumors, but also to achieve tumor subtyping (e.g., through the application of different target-color combinations).73 One limitation of in vivo optical imaging is the reduced tissue penetration, which makes it more suitable for ex vivo specimens, superficial tumors and preclinical studies. Fluorescence-labeled PVX could be functionalized with additional cancer-specific detection ligands that may improve the versatility of molecular imaging to study disease progression or recurrence during and after treatment, e.g. to evaluate novel therapies.
Acknowledgements
This work was supported by a grant from the National Science Foundation: NSF CMMI NM 333651 to NFS, and a Scholarship RFwN from RWTH Aachen University to CD.
Notes and references
- R. A. Miller, N. Stephanopoulos, J. M. McFarland, A. S. Rosko, P. L. Geissler and M. B. Francis, Impact of assembly state on the defect tolerance of TMV-based light harvesting arrays, J. Am. Chem. Soc., 2010, 132, 6068–6074 CrossRef CAS PubMed.
- Y. Z. Ma, R. A. Miller, G. R. Fleming and M. B. Francis, Energy transfer dynamics in light-harvesting assemblies templated by the tobacco mosaic virus coat protein, J. Phys. Chem. B, 2008, 112, 6887–6892 CrossRef CAS PubMed.
- R. A. Miller, A. D. Presley and M. B. Francis, Self-assembling light-harvesting systems from synthetically modified tobacco mosaic virus coat proteins, J. Am. Chem. Soc., 2007, 129, 3104–3109 CrossRef CAS PubMed.
- J. Sun, C. DuFort, M. C. Daniel, A. Murali, C. Chen, K. Gopinath, B. Stein, M. De, V. M. Rotello, A. Holzenburg, C. C. Kao and B. Dragnea, Core-controlled polymorphism in virus-like particles, Proc. Natl. Acad. Sci. U. S. A., 2007, 104, 1354–1359 CrossRef CAS PubMed.
- Y. J. Lee, Y. Lee, D. Oh, T. Chen, G. Ceder and A. M. Belcher, Biologically activated noble metal alloys at the nanoscale: for lithium ion battery anodes, Nano Lett., 2010, 10, 2433–2440 CrossRef CAS PubMed.
- K. T. Nam, D. W. Kim, P. J. Yoo, C. Y. Chiang, N. Meethong, P. T. Hammond, Y. M. Chiang and A. M. Belcher, Virus-enabled synthesis and assembly of nanowires for lithium ion battery electrodes, Science, 2006, 312, 885–888 CrossRef CAS PubMed.
- R. J. Tseng, C. Tsai, L. Ma, J. Ouyang, C. S. Ozkan and Y. Yang, Digital memory device based on tobacco mosaic virus conjugated with nanoparticles, Nat. Nanotechnol., 2006, 1, 72–77 CrossRef CAS PubMed.
- E. Royston, A. Ghosh, P. Kofinas, M. T. Harris and J. N. Culver, Self-assembly of virus-structured high surface area nanomaterials and their application as battery electrodes, Langmuir, 2008, 24, 906–912 CrossRef CAS PubMed.
- R. Chitale, Merck hopes to extend gardasil vaccine to men, J. Natl. Cancer Inst., 2009, 101, 222–223 CrossRef PubMed.
- T. C. Liu, E. Galanis and D. Kirn, Clinical trial results with oncolytic virotherapy: a century of promise, a decade of progress, Nat. Clin. Pract. Oncol., 2007, 4, 101–117 CrossRef CAS PubMed.
- T. Shirakawa, Clinical trial design for adenoviral gene therapy products, Drug News Perspect., 2009, 22, 140–145 CrossRef CAS PubMed.
- S. Franzen and S. A. Lommel, Targeting cancer with ‘smart bombs’: equipping plant virus nanoparticles for a ‘seek and destroy’ mission, Nanomedicine, 2009, 4, 575–588 CrossRef CAS PubMed.
- I. Yildiz, S. Shukla and N. F. Steinmetz, Applications of viral nanoparticles in medicine, Curr. Opin. Biotechnol., 2011, 22, 901–908 CrossRef CAS PubMed.
- R. Fischer, J. Drossard, U. Commandeur, S. Schillberg and N. Emans, Towards molecular farming in the future: moving from diagnostic protein and antibody production in microbes to plants, Biotechnol. Appl. Biochem., 1999, 30(Pt 2), 101–108 CAS.
- V. Yusibov, S. Rabindran, U. Commandeur, R. M. Twyman and R. Fischer, The potential of plant virus vectors for vaccine production, Drugs R D, 2006, 7, 203–217 CAS.
- R. Fischer, Y. C. Liao, K. Hoffmann, S. Schillberg and N. Emans, Molecular farming of recombinant antibodies in plants, Biol. Chem., 1999, 380, 825–839 CrossRef CAS PubMed.
- C. S. Rae, I. W. Khor, Q. Wang, G. Destito, M. J. Gonzalez, P. Singh, D. M. Thomas, M. N. Estrada, E. Powell, M. G. Finn and M. Manchester, Systemic trafficking of plant virus nanoparticles in mice via the oral route, Virology, 2005, 343, 224–235 CrossRef CAS PubMed.
- H. S. Leong, N. F. Steinmetz, A. Ablack, G. Destito, A. Zijlstra, H. Stuhlmann, M. Manchester and J. D. Lewis, Intravital imaging of embryonic and tumor neovasculature using viral nanoparticles, Nat. Protocols, 2010, 5, 1406–1417 CAS.
- J. D. Lewis, G. Destito, A. Zijlstra, M. J. Gonzalez, J. P. Quigley, M. Manchester and H. Stuhlmann, Viral nanoparticles as tools for intravital vascular imaging, Nat. Med., 2006, 12, 354–360 CrossRef CAS PubMed.
- F. M. Brunel, J. D. Lewis, G. Destito, N. F. Steinmetz, M. Manchester, H. Stuhlmann and P. E. Dawson, Hydrazone ligation strategy to assemble multifunctional viral nanoparticles for cell imaging and tumor targeting, Nano Lett., 2010, 10, 1093–1097 CrossRef CAS PubMed.
- N. F. Steinmetz, A. Ablack, J. L. Hickey, J. Ablack, B. Manocha, J. S. Mymryk, L. G. Luyt and J. D. Lewis, Intravital Imaging of Human Prostate Cancer using Viral Nanoparticles Targeted to Gastrin-Releasing Peptide Receptors, Small, 2011, 7, 1664–1672 CrossRef CAS PubMed.
- N. F. Steinmetz, C. F. Cho, A. Ablack, J. D. Lewis and M. Manchester, Cowpea mosaic virus nanoparticles target surface vimentin on cancer cells, Nanomedicine, 2011, 6, 351–364 CrossRef CAS PubMed.
- R. K. Huang, N. F. Steinmetz, C. Y. Fu, M. Manchester and J. E. Johnson, Transferrin-mediated targeting of bacteriophage HK97 nanoparticles into tumor cells, Nanomedicine, 2011, 6, 55–68 CrossRef CAS PubMed.
- M. L. Hovlid, N. F. Steinmetz, B. Laufer, J. L. Lau, J. Kuzelka, Q. Wang, T. Hyypia, G. R. Nemerow, H. Kessler, M. Manchester and M. G. Finn, Guiding plant virus particles to integrin-displaying cells, Nanoscale, 2012, 4, 3698–3705 RSC.
- S. Shukla, A. L. Ablack, A. M. Wen, K. L. Lee, J. D. Lewis and N. F. Steinmetz, Increased Tumor Homing and Tissue Penetration of the Filamentous Plant Viral Nanoparticle Potato virus X, Mol. Pharm., 2013, 10, 33–42 CrossRef CAS PubMed.
- S. Shukla, A. M. Wen, N. R. Ayat, U. Commandeur, R. Gopalkrishnan, A. M. Broome, K. W. Lozada, R. A. Keri and N. F. Steinmetz, Biodistribution and clearance of a filamentous plant virus in healthy and tumor-bearing mice, Nanomedicine, 2014, 9, 221–235 CrossRef CAS PubMed.
- A. K. Iyer, G. Khaled, J. Fang and H. Maeda, Exploiting the enhanced permeability and retention effect for tumor targeting, Drug Discov. Today, 2006, 11, 812–818 CrossRef CAS PubMed.
- S. Cai, K. Vijayan, D. Cheng, E. M. Lima and D. E. Discher, Micelles of Different Morphologies—Advantages of Worm-like Filomicelles of PEO-PCL in Paclitaxel Delivery, Pharm. Res., 2007, 24, 2099–2109 CrossRef CAS PubMed.
- V. P. Chauhan, Z. Popovic, O. Chen, J. Cui, D. Fukumura, M. G. Bawendi and R. K. Jain, Fluorescent nanorods and nanospheres for real-time in vivo probing of nanoparticle shape-dependent tumor penetration, Angew. Chem. Int. Ed., 2011, 50, 11417–11420 CrossRef CAS PubMed.
- D. A. Christian, S. Cai, O. B. Garbuzenko, T. Harada, A. L. Zajac, T. Minko and D. E. Discher, Flexible filaments for in vivo imaging and delivery: persistent circulation of filomicelles opens the dosage window for sustained tumor shrinkage, Mol. Pharm., 2009, 6, 1343–1352 CrossRef CAS PubMed.
- P. Decuzzi, B. Godin, T. Tanaka, S.-Y. Lee, C. Chiappini, X. Liu and M. Ferrari, Size and shape effects in the biodistribution of intravascularly injected particles, J. Controlled Release, 2010, 141, 320–327 CrossRef CAS PubMed.
- Y. Geng, P. Dalhaimer, S. Cai, R. Tsai, M. Tewari, T. Minko and D. E. Discher, Shape effects of filaments versus spherical particles in flow and drug delivery, Nat. Nanotechnol., 2007, 2, 249–255 CrossRef CAS PubMed.
- F. Gentile, C. Chiappini, D. Fine, R. C. Bhavane, M. S. Peluccio, M. M.-C. Cheng, X. Liu, M. Ferrari and P. Decuzzi, The effect of shape on the margination dynamics of non-neutrally buoyant particles in two-dimensional shear flows, J. Biomech., 2008, 41, 2312–2318 CrossRef CAS PubMed.
- S.-Y. Lee, M. Ferrari and P. Decuzzi, Shaping nano-/micro-particles for enhanced vascular interaction in laminar flows, Nanotechnology, 2009, 20, 495101 CrossRef PubMed.
- S. Barua, J. W. Yoo, P. Kolhar, A. Wakankar, Y. R. Gokarn and S. Mitragotri, Particle shape enhances specificity of antibody-displaying nanoparticles, Proc. Natl. Acad. Sci. U. S. A., 2013, 110, 3270–3275 CrossRef CAS PubMed.
- J. A. Champion and S. Mitragotri, Role of target geometry in phagocytosis, Proc. Natl. Acad. Sci. U. S. A., 2006, 103, 4930–4934 CrossRef CAS PubMed.
-
R. Koenig and D. E. Lesemann, Potato virus X, potexvirus group, Association of Applied Biologists, Warwick, 1989 Search PubMed.
- N. F. Steinmetz, M. E. Mertens, R. E. Taurog, J. E. Johnson, U. Commandeur, R. Fischer and M. Manchester, Potato virus X as a novel platform for potential biomedical applications, Nano Lett., 2010, 10, 305–312 CrossRef CAS PubMed.
- K. Uhde, R. Fischer and U. Commandeur, Expression of multiple foreign epitopes presented as synthetic antigens on the surface of Potato virus X particles, Arch. Virol., 2005, 150, 327–340 CrossRef CAS PubMed.
- K. Uhde-Holzem, R. Fischer and U. Commandeur, Genetic stability of recombinant potato virus X virus vectors presenting foreign epitopes, Arch. Virol., 2007, 152, 805–811 CrossRef CAS PubMed.
- S. S. Cruz, A. G. Roberts, D. A. Prior, S. Chapman and K. J. Oparka, Cell-to-cell and phloem-mediated transport of potato virus X. The role of virions, Plant Cell, 1998, 10, 495–510 CAS.
- J. Tilsner, O. Linnik, M. Louveaux, I. M. Roberts, S. N. Chapman and K. J. Oparka, Replication and trafficking of a plant virus are coupled at the entrances of plasmodesmata, J. Cell Biol., 2013, 201, 981–995 CAS.
- D. Baulcombe, S. Chapman and S. Santa Cruz, Jellyfish green fluorescent protein as a reporter for virus infections, Plant J., 1995, 7, 1045–1053 CAS.
- K. J. Oparka, A. G. Roberts, D. A. M. Prior, S. Chapman, D. Baulcombe and S. Santa Cruz, Imaging the greenfluorescent protein in plants – viruses carrying the torch, Protoplasma, 1996, 189, 133–141 CrossRef.
- S. S. Cruz, S. Chapman, A. G. Roberts, I. M. Roberts, D. A. Prior and K. J. Oparka, Assembly and movement of a plant virus carrying a green fluorescent protein overcoat, Proc. Natl. Acad. Sci. U. S. A., 1996, 93, 6286–6290 CrossRef CAS.
- J. Maclean, M. Koekemoer, A. J. Olivier, D. Stewart, I. I. Hitzeroth, T. Rademacher, R. Fischer, A. L. Williamson and E. P. Rybicki, Optimization of human papillomavirus type 16 (HPV-16) L1 expression in plants: comparison of the suitability of different HPV-16 L1 gene variants and different cell-compartment localization, J. Gen. Virol., 2007, 88, 1460–1469 CrossRef CAS PubMed.
- N. C. Shaner, R. E. Campbell, P. A. Steinbach, B. N. Giepmans, A. E. Palmer and R. Y. Tsien, Improved monomeric red: orange and yellow fluorescent
proteins derived from Discosoma sp. red fluorescent protein, Nat. Biotechnol., 2004, 22, 1567–1572 CrossRef CAS PubMed.
- K. L. Lee, K. Uhde-Holzem, R. Fischer, U. Commandeur and N. F. Steinmetz, Genetic engineering and chemical conjugation of potato virus X, Methods Mol. Biol., 2014, 1108, 3–21 Search PubMed.
- U. K. Laemmli, Cleavage of structural proteins during the assembly of the head of bacteriophage T4, Nature, 1970, 227, 680–685 CrossRef CAS.
- S. Inouye and F. I. Tsuji, Aequorea green fluorescent protein expression of the gene and fluorescence characteristics of the recombinant protein, FEBS Lett., 1994, 341, 277–280 CrossRef CAS.
- C. M. Wilson, Staining of proteins on gels: comparison of dyes and procedures, Methods Enzymol., 1983, 91, 236–247 CAS.
- R. Koenig, P. Lüddecke and A. M. Haeberle, Detection of beet necrotic yellow vein virus strains, variants and mixed infections by examining single-strand conformation polymorphisms of immunocapture RT-PCR products, J. Gen. Virol., 1995, 76, 2051–2055 CrossRef CAS.
- J. Verchot-Lubicz, L. Torrance, A. G. Solovyev, S. Y. Morozov, A. O. Jackson and D. Gilmer, Varied movement strategies employed by triple gene block-encoding viruses, Mol. Plant Microbe Interact., 2010, 23, 1231–1247 CrossRef CAS PubMed.
- S. S. Cruz, Perspective: Phloem transport of viruses and macromolecules-what goes in must come out, Trends Microbiol., 1999, 7, 237–241 CrossRef.
-
R. Turgeon, Symplastic phloem loading and the sink-source transition in leaves: a model, in Recent Advances Phloem Transport and Assimilate Compartmentation, ed. V. L. Bonnemain, S. Delrot, J. Dainty and W. J. Lucas, 1991 Search PubMed.
- P. Matile, Biochemistry of Indian summer: physiology of autumnal leaf coloration, Exp. Gerontol., 2000, 35, 145–158 CrossRef CAS.
- H. B. Scholthof, B. G. Scholthof and A. O. Jackson, Plant virus vectors for transient expression of foreign proteins in plants, Annu. Rev. Phytopathol., 1996, 34, 229–323 Search PubMed.
- M. D. Ryan, A. M. King and G. P. Thomas, Cleavage of foot-and-mouth disease virus polyprotein is mediated by residues located within a 19 amino acid sequence, J. Gen. Virol., 1991, 72, 2727–2732 CrossRef CAS.
- M. D. Ryan and J. Drew, Foot-and-mouth disease virus 2A oligopeptide mediated cleavage of an artificial polyprotein, EMBO J., 1994, 13, 928–933 CAS.
- C. Ma, F. M. Marassi, D. H. Jones, S. K. Straus, S. Bour, K. Strebel, U. Schubert, M. Oblatt-Montal, M. Montal and S. J. Opella, Expression, purification, and activities of full-length and truncated versions of the integral membrane protein Vpu from HIV-1, Protein Sci., 2002, 11, 546–557 CrossRef CAS PubMed.
- M. L. Donnelly, G. Luke, A. Mehrotra, X. Li, L. E. Hughes, D. Gani and M. D. Ryan, Analysis of the aphthovirus 2A/2B polyprotein ‘cleavage’ mechanism indicates not a proteolytic reaction, but a novel translational effect: a putative ribosomal ‘skip’, J. Gen. Virol., 2001, 82, 1013–1025 CAS.
- M. L. Donnelly, L. E. Hughes, G. Luke, H. Mendoza, E. ten Dam, D. Gani and M. D. Ryan, The ‘cleavage’ activities of foot-and-mouth disease virus 2A site-directed mutants and naturally occurring ‘2A-like’ sequences, J. Gen. Virol., 2001, 82, 1027–1041 CAS.
- L. Smolenska, I. M. Roberts, D. Learmonth, A. J. Porter, W. J. Harris, T. M. Wilson and S. Santa Cruz, Production of a functional single chain antibody attached to the surface of a plant virus, FEBS Lett., 1998, 441, 379–382 CrossRef CAS.
- G. J. O'Brien, C. J. Bryant, C. Voogd, H. B. Greenberg, R. C. Gardner and A. R. Bellamy, Rotavirus VP6 expressed by PVX vectors in Nicotiana benthamiana coats PVX rods and also assembles into viruslike particles, Virology, 2000, 270, 444–453 CrossRef CAS PubMed.
- E. Sadauskas, H. Wallin, M. Stoltenberg, U. Vogel, P. Doering, A. Larsen and G. Danscher, Kupffer cells are central in the removal of nanoparticles from the organism, Part Fibre Toxicol., 2007, 4, 10 CrossRef PubMed.
- Z. Liu, C. Davis, W. Cai, L. He, X. Chen and H. Dai, Circulation and long-term fate of functionalized, biocompatible single-walled carbon nanotubes in mice by Raman spectroscopy, Proc. Natl. Acad. Sci. U. S. A., 2008, 105, 1410–1415 CrossRef CAS PubMed.
- S. C. Gad, K. L. Sharp, C. Montgomery, J. D. Payne and G. P. Goodrich, Evaluation of the Toxicity of Intravenous Delivery of Auroshell Particles (Gold-Silica Nanoshells), Int. J. Toxicol., 2013, 31, 584–594 CrossRef PubMed.
- N. Khlebtsov and L. Dykman, Biodistribution and toxicity of engineered gold nanoparticles: a review of in vitro and in vivo studies, Chem. Soc. Rev., 2011, 40, 1647 RSC.
- C. S. Rae, I. Wei Khor, Q. Wang, G. Destito, M. J. Gonzalez, P. Singh, D. M. Thomas, M. N. Estrada, E. Powell, M. G. Finn and M. Manchester, Systemic trafficking of plant virus nanoparticles in mice via the oral route, Virology, 2005, 343, 224–235 CrossRef CAS PubMed.
- P. Singh, D. Prasuhn, R. M. Yeh, G. Destito, C. S. Rae, K. Osborn, M. G. Finn and M. Manchester, Bio-distribution, toxicity and pathology of cowpea mosaic virus nanoparticles in vivo, J. Controlled Release, 2007, 120, 41–50 CrossRef CAS PubMed.
- C. R. Kaiser, M. L. Flenniken, E. Gillitzer, A. L. Harmsen, A. G. Harmsen, M. A. Jutila, T. Douglas and M. J. Young, Biodistribution studies of protein cage nanoparticles demonstrate broad tissue distribution and rapid clearance in vivo, Int. J. Nanomedicine, 2007, 2, 715–733 CAS.
- M. A. Bruckman, L. N. Randolph, A. Vanmeter, S. Hern, A. J. Shoffstall, R. E. Taurog and N. F. Steinmetz, Biodistribution, pharmacokinetics, and blood compatibility of native and PEGylated tobacco mosaic virus nano-rods and -spheres in mice, Virology, 2014, 449, 163–173 CrossRef CAS PubMed.
- Y. Koyama, T. Barrett, Y. Hama, G. Ravizzini, P. L. Choyke and H. Kobayashi, In vivo molecular imaging to diagnose and subtype tumors through receptor-targeted optically labeled monoclonal antibodies, Neoplasia, 2007, 9, 1021–1029 CrossRef CAS.
Footnote |
† Electronic supplementary information (ESI) available: Plant photography and quantitative data. See DOI: 10.1039/c3bm60277j |
|
This journal is © The Royal Society of Chemistry 2014 |