DOI:
10.1039/C3BM60204D
(Paper)
Biomater. Sci., 2014,
2, 390-398
A new method to cross-link a polyplex for enhancing in vivo stability and transfection efficiency†
Received
30th August 2013
, Accepted 18th October 2013
First published on 27th November 2013
Abstract
Disulfide-exchange was found to cross-link the polyplex of disulfide-containing poly(amido amine) and pDNA with heating of the polyplex solution over a short time. The cross-linked polyplexes based on disulfide-containing poly(amido amine) have excellent stability under physiological salt conditions, and have significantly enhanced transfection activity in the serum media compared to non-cross-linked polyplexes. In vivo, ICR mice were injected with the polyplex through the tail vein, the results show that the transfection efficiency of the cross-linked polyplex is higher than that of the non-cross-linked variety. Furthermore, the polyplex containing Cy5 labelled DNA was also injected into the mice to illustrate the stability and distribution of the polyplex, cross-linked polyplexes show a much brighter luminescence than the non-cross-linked ones. This method does not need a cross-linker or catalyst, and there are no impurities produced, it may be an elegant approach to resolve the dilemma of in vivo application of a DNA polyplex, with excellent stability whilst in circulation and a rapid unpacking of the polyplex inside the cells.
1. Introduction
In the past decades, gene therapy has attracted a great deal of attention due to its potential application in treating certain acquired or inherited diseases including AIDS, cancer, genetic disorders, etc.1–4 A successful gene therapy is largely dependent on the vectors that can efficiently deliver genes to target cells.3,5 Though viral vector systems show higher gene transfection efficiencies than non-viral gene carrier systems, potential risks of viral systems include wild type virus regeneration, immunogenicity and cancer,6–8 thereby the safety concerns regarding the use of a virus vector in humans makes a non-viral delivery system an attractive alternative.9,10 Several polymer-based materials including poly(L-lysine) (PLL),11 poly(ethylene imine) (PEI),12 natural polymers,13 poly(beta-amino ester),14 cationic aliphatic polyesters,15–17 have been used as vectors for delivering genes into cells. Though these polymer-based vectors often exhibit successful gene delivery in vitro, they do not work very well in vivo.18 To deliver genes to target cells, a series of both extra- and intracellular obstacles should be overcome, including DNA condensation, cellular uptake, endosome escape, and DNA release in the cytoplasm. In vivo, gene delivery systems that work through a systemic route demand high stability in circulating blood and an efficient gene expression at the target site. Generally, systemic delivery of a gene is hindered by complex instability under physiological conditions.19–21 The physiological salt and serum proteins readily bind with such polyplexes, which promotes premature dissociation of polyplexes and the release of entrapped DNA.22 Consequently, extracellular stability is crucial to gene delivery in vivo, and a strategy is needed to keep the polyplex structure stable in the extracytoplasmic environment, while inducing efficient release of entrapped DNA from the polyplexes after its movement into the cytoplasmic compartment. PEGylation is one of the predominant methods used to reduce dissociation and prolong circulation time.23,24 However, PEG on the surface of a polyplex can decrease the cellular uptake, and there is still a possibility of unfavorable dissociation before entering the cytoplasm of the target cells through the interaction with negatively charged biomacromolecules in the entity.20,21 Kataoka et al. reported a promising approach of reversibly cross-linking polyplex nanoparticles with disulfide bonds, which are cleavable in reductive intracellular environments,25 where glutathione concentration is 50–1000 times higher than that in the extracellular milieu.26 In their study, a thiolated poly(ethylene glycol)-poly(L-lysine) block copolymer (PEG-PLL) was prepared, subsequently the polyplex nanoparticles were cross-linked, and the results show that the reversibly cross-linked polyplex systems have a high stability in extracellular environments and an effective releasing capacity of pDNA in the intracellular compartment for efficient transfection. However, the cross-linking procedure is complex, it needs incubation of the polyplex solution overnight, and dialysis of the polyplex solution against Tris-HCl containing dimethyl sulfoxide for 24 h to remove impurities, followed by 2 days of additional dialysis for removal of dimethyl sulfoxide. Here, we report a new and easy method for reversibly cross-linking a polyplex via heating the polyplex solution (as shown in Scheme 1) by using the self-cross-linking of disulfide-containing polymers.27–37 We prepared a disulfide-containing hyperbranched cationic polymer, it can self-cross-link in a short time without the need of any catalyst or cross-linking agent. The polyplexes of the hyperbranched cationic polymers and pDNA can rapidly self-cross-link under mild conditions (as shown in Scheme 1). The advantages are that there is no need for any oxidation agent or cross-linking agent, and that the cross-linking reaction is fast under mild conditions.
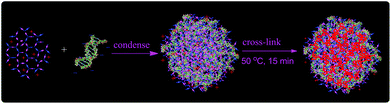 |
| Scheme 1 The procedure of disulfide-containing poly(amido amine) condensing DNA and cross-linking the formed polyplex. | |
2. Experimental section
2.1. Materials
N,N-Dimethyldipropylenetriamine (DMDPTA, 99% Aldrich), dithiothreitol (DTT, >98%, Sigma-Aldrich), ethidium bromide (EtBr, >95%, Alfa-Aesar), 3-[4,5-dimethylthiazol-2-yl]-2,5-diphenyltetrazolium bromide (MTT, >98% Solarbio), and branched polyethylenimine (bPEI, MW 25 kDa, Aldrich) were used as received. Plasmid DNA, gWiz high expression luciferase (gWiz-Luc), containing luciferase reporter gene was purchased from Aldevron. Sodium hydroxide was purchased from Sinopharm Chemical Reagent Co. Methanol (99.8%, Sigma-Aldrich), acetone (99.5%, Aldrich) were used as received without further purification. Cy5-labeled DNA was obtained from Sangon.
2.2. Synthesis of N,N′-cystaminebisacrylamide (CBA)38
Cystamine dihydrochloride (5.8 g, 25 mmol) was dissolved in 50 mL water in a 250 mL flask. An aqueous solution of NaOH (10 mL, 10 M) and a solution of acryloyl chloride (4.7 g, 50 mmol) in dichloromethane (5 mL) were added dropwise at the same time under stirring at 0 °C. The reaction was performed for 3 h at room temperature after the addition of acryloyl chloride. The product was obtained by filtration and crystallization from ethyl acetate.
2.3. Synthesis of disulfide-containing hyperbranched poly(amido amine)
Hyperbranched poly(amido amine) was synthesized by Michael addition polymerization of equal molar amounts of N,N-dimethyldipropylenetriamine (DMDPTA) and N,N′-cystaminebisacrylamide (CBA). Typically, CBA (0.520 g, 2.0 mmol) was added into a small vial containing DMDPTA (0.318 g, 2.0 mmol) in a methanol–water mixture (3.5 mL, 8/2, v/v). Polymerization was carried out for 3 days at 60 °C. The polymer was isolated by precipitation with acetone. The obtained polymer has a molecular weight and PDI of 71.0 kDa and 1.2, respectively.
2.4. Characterization
1H NMR and 13C NMR studies were performed on a Bruker spectrometer (300 MHz). The weight-average molecular weight (Mw), number-average molecular weight (Mn) and polydispersity index of the polymers were determined by size exclusion chromatography (SEC) using a Shimadzu LC-10ADVP liquid chromatograph equipped with a Polymer Labs PL gel 5 μm mixed C column. The system was equipped with a refractometer. DMF was used as the eluent at a flow rate of 1.0 mL min−1 and temperature of 35 °C. The determination of hydrodynamic diameters was performed by dynamic light scattering using a Malvern Zetasizer Nano ZS90 instrument (Malvern Co.) equipped with a 4 mW He–Ne laser (λ = 633 nm) at a 90° scattering angle. The hydrodynamic diameter DH was calculated using the Stokes–Einstein equation as DH = KBT/3πηDt, where KB is the Boltzmann constant, T is the absolute temperature, η is the sample viscosity, and Dt is the diffusion coefficient.
2.5. Polyplex formation
Polyplex consisting of plasmid DNA and disulfide-containing hyperbranched poly(amido amine) was prepared in PBS (10 mM) at pH 7.4. Briefly, the polymer solution was added rapidly to the DNA, and mixed by vortex, which was followed by incubation for 20 minutes at room temperature prior to use. When N/P ratios were investigated, the concentration of DNA was 20 μg mL−1, and the N/P ratios were between 20 and 40.
2.6. Cross-linking polyplexes
NaOH was used to tune the pH of the polyplex solution to ∼9.0, and polyplex solution was incubated at 50 °C for 15 min, subsequently, the pH of the polyplex solution was tuned back to 7.4 from 9.0.
2.7. The stability of the polyplex nanoparticles in the presence of NaCl
The polyplex solution was adjusted to 20 μg mL−1 of DNA and 2.5 μg mL−1 of EtBr by adding 10 mM PBS (pH 7.4) and EtBr. Then NaCl was added to adjust the NaCl concentration to 150 mM. Fluorescence was measured and set to 100% using an excitation wavelength of 510 nm and an emission wavelength of 590 nm, and relative fluorescence intensity was translated to a ratio of the relative fluorescence as time went on. Relative fluorescence intensity was calculated as follows:
Fr = (Fsample − F0)t/(Fsample − F0)t=0 |
where Fsample was fluorescence of the sample, F0 was fluorescence of the background, and t was time.
2.8. Agarose gel electrophoresis assay
Polyplexes were loaded onto a 0.8% agarose gel, then the assay was run for 50 min at 90 V in a TAE (Tris-acetate-EDTA) running buffer. The gel was soaked in 0.5 μg mL−1 EtBr for 20 min. The gel was visualized under UV illumination on a Kodak Gel Logic 100 Imaging System.
2.9.
In vitro transfection
Transfection efficiency with gWiz-Luc plasmid DNA was tested in 3 cell lines as follows: cells were seeded at a density of 30
000 cells per well on 48-well cell culture plates, 24 hours prior to the transfection experiment. The polyplexes were prepared as described above, and 25 μL solution was added to each well containing 150 μL of DMEM (Dulbecco's Modified Eagle's Medium) with or without serum. After 4 h of incubation, the transfection mixture was removed and the cells were cultured for an additional 24 h in fresh full DMEM media. To determine the level of luciferase expression, the culture medium was discarded and cell lysate was harvested after incubation of cells for 30 min at room temperature in 100 μL of cell lysis reagent buffer (Promega). To measure the luciferase content, 100 μL of luciferase assay buffer (20 mM glycylglycine (pH 8), 1 mM MgCl2, 0.1 mM EDTA, 3.5 mM DTT, 0.5 mM ATP, 0.27 mM coenzyme) was automatically injected into 20 μL of cell lysate, and the luminescence was integrated over 10 s using a single tube Sirius luminometer. Total cellular protein in the cell lysate was determined by the bicinchoninic acid (BCA) protein assay using a calibration curve constructed with standard bovine serum albumin solutions (Pierce). The luciferase transfection results are expressed in relative light units (RLU) per mg of cellular protein. Unless stated otherwise, the results are expressed as mean RLU mg−1 of protein ±SD of triplicate experiments.
2.10. Animals
Male ICR mice (Vital River Laboratories, Beijing, China) were used at the age of 7–8 weeks, and received care in compliance with the guidelines outlined in the Guide for the Care and Use of Laboratory Animals. The procedure was approved by the University of Science and Technology of China Animal Care and Use Committee. Three mice per sample were used for one condition.
2.11.
In vivo transfection
Animals were injected with the complexes (20 μg DNA, 200 μL per mouse) through the tail vein. Organs including lung, liver, spleen, heart and kidneys were dissected 48 h after the injection. Lysis buffer was added to the collected organs. Each organ was homogenized for 15–20 s with the tissue at 20
000 rpm and for 10 min at 12
000 rpm, to make tissue homogenate, which was then centrifuged in a microcentrifuge. An aliquot of the supernatant (50 μL) was used for the luciferase activity assay. The protein concentration of each tissue extract was determined by a standard protein assay. Luciferase activity in each sample was normalized to represent relative light units (RLU mg−1) of the extracted protein.
2.12.
In vivo distribution of polyplex
The Cy-5 DNA–cationic polymer polyplex was injected into the mice through the tail vein. The mice were sacrificed 48 h after injection. Lung, liver, spleen, heart and kidneys were excised and subjected to fluorescent image analysis using a Xenogen IVIS Lumina system (Caliper Life Sciences, Alameda, CA).
3. Results and discussion
The main goal of this study was to develop a simple method to reversibly cross-link polyplexes to enhance their stability in the extracytoplasmic environment. We prepared a disulfide-containing hyperbranched polycation because this polymer has low cytotoxicity (Fig. 1A) and high gene transfection efficiency, which can condense DNA to form polyplex, and the formed polyplex can be easily cross-linked via disulfide exchange when heat is applied. The disulfide cross-linked polyplex is stable in circulating blood, and DNA can be efficiently released after these polyplexes move into a cell, which is believed to be a prerequisite for efficient in vivo transfection.38
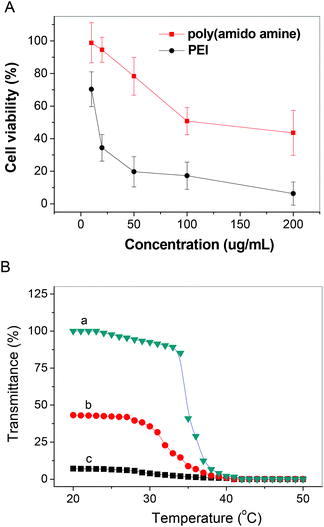 |
| Fig. 1 (A) The cytotoxicity of the prepared disulfide-containing hyperbranched poly(amido amine), and (B) the change of transmittance of the disulfide-containing hyperbranched poly(amido amine) solution (5.0 mg mL−1) in 10 mM PBS (pH is 9.0) with temperature: (a) the change of transmittance of the disulfide-containing hyperbranched poly(amido amine) solution with temperature with increasing temperature, (b) the change of transmittance of the disulfide-containing hyperbranched poly(amido amine) solution with temperature with decreasing temperature after the solution incubated at 50 °C for 10 min, (c) the change of transmittance of the disulfide-containing hyperbranched poly(amido amine) solution with temperature in decreasing temperature style after the solution was incubated at 50 °C for 15 min. | |
3.1. Synthesis of bioreducible hyperbranched poly(amido amine)
We employed a Michael addition polymerization of DMDPTA and CBA to prepare a disulfide-containing hyperbranched poly(amido amine) (as shown in Scheme 2). In this type of Michael addition polymerization, the amine reactivity typically fits the following order: secondary amine (original) > primary amine > secondary amine (formed),38 and the temperature has a large effect on the amine reactivity. Based on previous findings, the reaction of an equal molar amount of a triamine monomer and a bisacrylamide monomer produces hyperbranched polymers under high temperature.27 The branched structure of the formed polymer is verified by 13C NMR. The polymer was dialyzed against distilled water acidified with HCl to remove low molecular weight fractions and to convert the polymers to their hydrochloride salt. This hyperbranched poly(amido amine) is temperature-responsive because N,N′-dimethylamine can hydrate at low temperature in aqueous solution, but easily dehydrate at high temperature. For the similar temperature-responsive hyperbranched poly(amido amine) without a disulfide bond, the transmittance of solution can go back to 100% when temperature goes back to 20 °C. The transmittance of the disulfide-containing poly(amido amine) solution decreases with increasing temperature, but the transmittance only returns to 40% when the solution temperature goes back to 20 °C after the solution has been at 50 °C for 10 min, the transmittance remained almost unchanged after the solution temperature goes back to 20 °C after the solution has been at 50 °C for 15 min (Fig. 1). All of these results indicate that the cross-linking of the hyperbranched poly(amido amine) can be finished in 15 min.
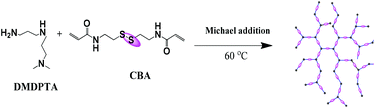 |
| Scheme 2 The synthesis of the disulfide-containing hyperbranched poly(amido amine). | |
3.2. Cross-linking the polyplex
Although many disulfide-containing polymers have shown a high gene transfection efficiency, it is difficult to reversibly cross-link the polyplexes. Here, we cross-linked disulfide-contained hyperbranched poly(amido amine) polyplexes simply via heating of a polyplex solution. The heating time has a large effect on the size of the polyplex as shown in Fig. 2. The size of the polyplex increases from ∼200 nm to 1500 nm when the heating time was increased from 20 min to 100 min. But the size changed very little within 25 min as shown in Fig. 2. Cells can easily uptake the particles with sizes below 200 nm, therefore, the required cross-linking time is ∼15 min.
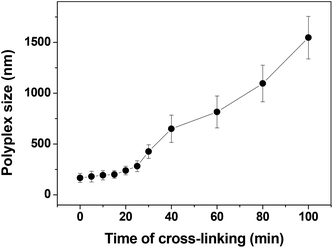 |
| Fig. 2 The size change of the disulfide-containing hyperbranched poly(amido amine) polyplex with heating time. | |
3.3. The stability of the cross-linked polyplex
The polyplex formed via the non-covalent interaction of a negatively-charged DNA and positively-charged polymer, it is not stable under physiological conditions. Low stability has been a major obstacle for many cationic polymeric formulations to be applied in vivo. Therefore, cross-linking the polyplex against the counter anion exchange reaction may play a key role in the dissociation of the polyplex in the intracellular compartment. The stability of the cross-linked polyplex was thus evaluated by the EtBr exclusion assay, the size change in dynamic light scattering (DLS) and agarose gel electrophoresis. It is clear that both cross-linked and non-cross-linked polyplexes could fully condense DNA at different N/P ratios. The effect of counter anion-induced dissociation of the polyplex in non-reductive conditions was evaluated by agarose gel electrophoresis. Obviously, under the non-reductive conditions (Fig. 3, lane 5 and lane 11), the bands of the free DNA were observed for the non-cross-linked polyplex in the presence of NaCl. But the free DNA bands were not detected in the presence of NaCl (Fig. 3, lanes 2 and 8) for all the cross-linked polyplexes, indicating the substantial stabilization of the cross-linked polyplex against the exchange reaction. Adding NaCl to the non-cross-linked systems in the presence of ethidium bromide led to a substantial increase in the fluorescence (Fig. 4A), whereas the change of fluorescence intensity observed for cross-linked polyplex systems was very small (Fig. 4A), which suggests that the cross-linking inhibits the ability of NaCl to bind polycation and regenerate ethidium bromide binding sites in the DNA. The particle size of the polyplexes was also investigated by DLS as shown in Fig. 4. Both particle size of the cross-linked and non-cross-linked polyplexes are ∼200 nm at beginning. In the presence of 150 mM NaCl, the sizes of all cross-linked polyplexes showed very little change after 2 h (Fig. 4B), but a big increase of polyplex sizes was observed for non-cross-linked polyplexes in the presence of 150 mM NaCl in 2 h. Therefore, the stability of the polyplex was greatly improved by cross-linking.
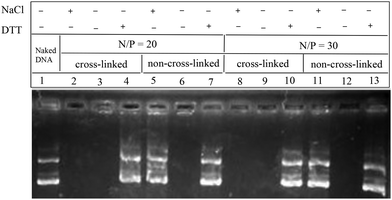 |
| Fig. 3 Agarose gel electrophoresis of polyplex prepared at an N/P ratio of 20/1 and 30/1. | |
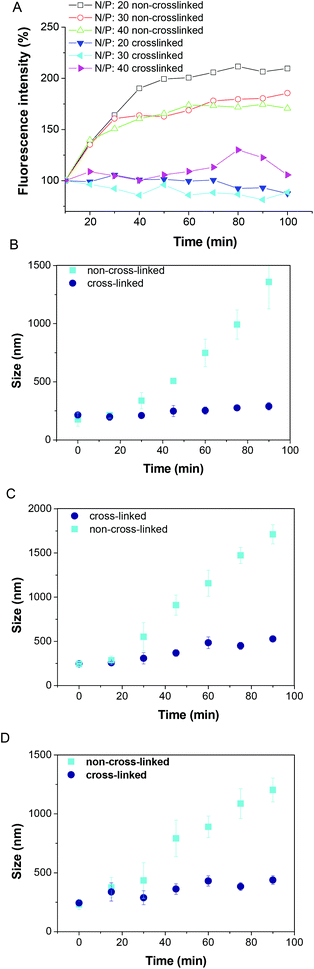 |
| Fig. 4 Restoration of ethidium bromide fluorescence of the cross-linked polyplex and non-cross-linked polyplex in the presence of NaCl in PBS (A), and the size change of polyplex at an N/P ratio of 20 (B), 30 (C) and 40 (D) in the presence of NaCl in PBS. | |
However, overly stable polyplexes are not desirable either, because, for efficient transfection, DNA has to be released into the nucleus. To investigate whether disulfide-cross-linked polyplexes would unpack under an intracellular mimicking reductive environment, an agarose gel electrophoresis experiment was carried out in the presence of 10 mM DTT. Obviously, the bands of the free DNA were observed for all of the cross-linked systems (Fig. 3, lane 4 and lane 10). Hence, these polyplexes on one hand may have excellent stability in circulation and on the other hand may be rapidly decomplexed to release DNA inside cells to achieve superior transfection.
3.4.
In vitro transfection
Since cross-linking time affects the sizes of polyplexes, and thereby affects cell uptake, in vitro transfection activity for the polyplexes obtained with different cross-linking times was carried out. In vitro transfection activity of polyplexes based on disulfide-cross-linked poly(amido amine) was assessed in COS-7 cells by luciferase assay. The disulfide-cross-linked poly(amido amine) polyplexes were obtained with different heating times (cross-linking time), and were used in the transfection experiments performed in a 10% serum-containing medium with N/P ratios of 10/1. It is clear that the cross-linking time has a large effect on transfection activity. For the polyplexes prepared with a short cross-linking time, the transfection activity is low, as the polyplexes are not completely cross-linked, and they can dissociate before moving into a cell in the presence of 10% serum; for the polyplexes prepared with a long cross-linking time, the transfection activity is low, as the polyplexes aggregated into large sized polyplexes, which do not easily get taken up by cells. The cross-linked polyplex has the highest transfection efficiency at a cross-linking time of ∼15 min (as shown in Fig. 5). It should be noted that ∼2 orders of magnitude enhancement of transfection efficiency has been observed for the cross-linked poly(amido amine) polyplex.
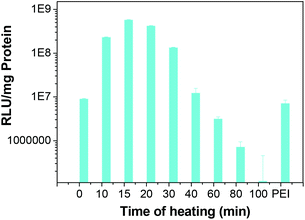 |
| Fig. 5 Transfection activity of polyplexes in COS-7 cells at different cross-linking times with 10% serum. | |
Transfection efficiency of the polyplexes with 0%, 2%, 5% and 10% serum was studied (Fig. 6) using COS-7 cells. The cross-linked polyplexes have a little higher transfection efficiency than that of non-cross-linked polyplexes without serum. With 2%, 5% or 10% serum, all the non-cross-linked poly(amido amine) polyplexes and PEI polyplex showed much lower transfection activity compared to those polyplexes without serum. With 10% serum, the transfection efficiency of non-cross-linked polyplexes is ∼1/400 of that of the cross-linked ones. The low transfection activity may result from the serum dissociating the polyplexes before moving into the cells. With 2% serum, the cross-linked polyplexes showed almost the same transfection activity as those without serum. With 5% serum, the cross-linked polyplex at an N/P of 20 showed a negligible decrease in transfection activity, the cross-linked polyplexes at an N/P of 30 and 40 showed a small decrease in transfection activity. With 10% serum, the cross-linked polyplexes showed a high transfection efficiency while the non-cross-linked poly(amido amine) polyplexes and PEI polyplex showed a very low transfection efficiency.
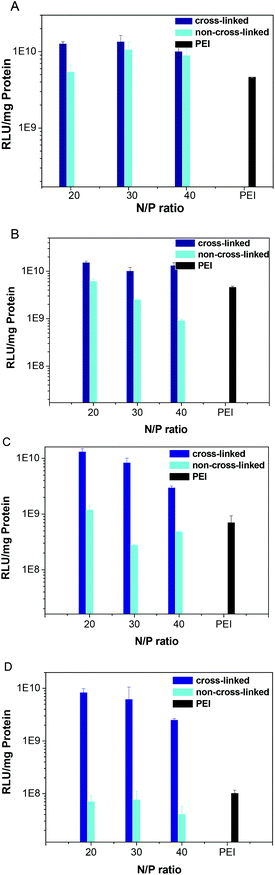 |
| Fig. 6 Transfection efficiency of both cross-linked and non-cross-linked polyplexes at the N/P ratio of 20, 30 and 40 in COS-7 cells with 0% (A), 2% (B), 5% (C), 10% serum (D). | |
Furthermore, the transfection experiments were also conducted in HeLa and CHO cells. It is clear that the cross-linked polyplexes show a much higher transfection activity than those of non-cross-linked polyplexes (Fig. 7 and 8). Disulfide-cross-linking greatly enhance the stability of the polyplex. In this case, cross-linking could effectively protect DNA from the ion exchange reaction, hence the DNA could be successfully delivered to the cells.
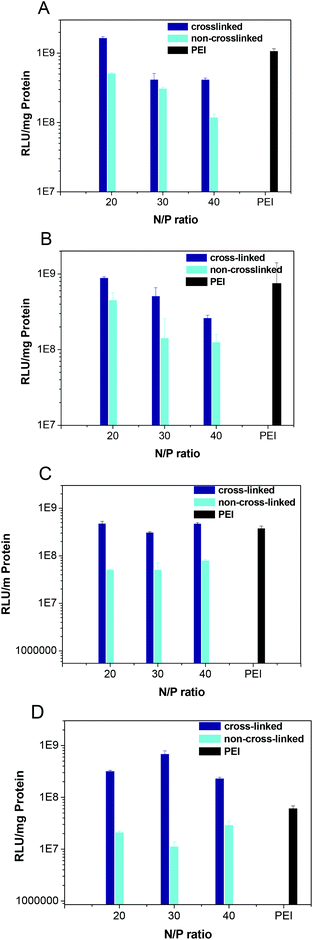 |
| Fig. 7 Transfection efficiency of both cross-linked and non-cross-linked polyplexes at an N/P ratio of 20, 30 and 40 in HeLa cells with 0% (A), 2% (B), 5% (C), 10% serum (D). | |
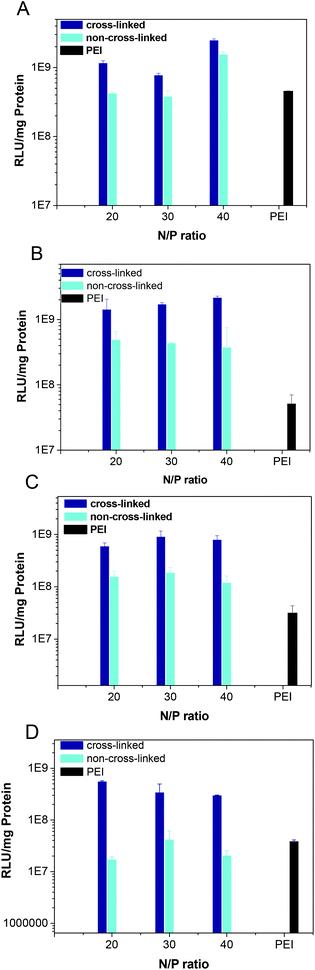 |
| Fig. 8 Transfection efficiency of both cross-linked and non-cross-linked polyplexes at an N/P ratio of 20, 30 and 40 in CHO cells with 0% (A), 2% (B), 5% (C), 10% serum (D). | |
It is known that there are many proteins in the blood, which could dissociate the polyplex. Thus, in order to gain high transfection efficiency, the stability is extremely crucial. In vivo transfection was conducted on ICR mice through tail vein injection. It is clear that the transfection efficiency of the cross-linked polyplex is higher than that of the non-cross-linked polyplex as shown in Fig. 9. Furthermore, the Cy5-labeled DNA polyplex was also injected into the mice through the tail vein to illustrate the stability and distribution of the polyplex. In Fig. 10, the cross-linked polyplex containing Cy5 labeled DNA shows much brighter luminescence than non-cross-linked polyplex.
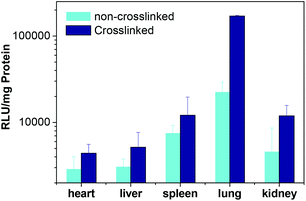 |
| Fig. 9 Transfection efficiency of both cross-linked and non-cross-linked polyplexes in different organs. | |
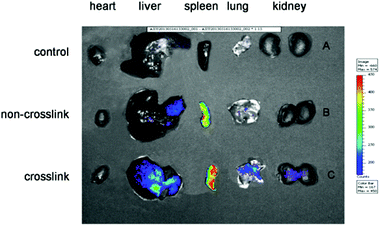 |
| Fig. 10 Fluorescent images of organs (A) the control, (B) non-cross-linked polyplex with Cy5 labeled DNA, (C) cross-linked polyplex with Cy5 labeled DNA. | |
4. Conclusions
We have demonstrated an easy and new method to cross-link disulfide-containing poly(amido amine) polyplexes. This disulfide-exchange cross-linking method can be carried out under mild conditions in a short time, it does not need a cross-linker or catalyst, and no impurities are produced. The cross-linked polyplexes based on disulfide-containing poly(amido amine) have excellent stability under physiological salt conditions, and have significantly enhanced transfection activity in the serum media compared to non-cross-linked polyplexes. This study indicates that cross-linking polyplexes via disulfide-exchange may be an elegant approach to reversibly cross-link polyplexes for enhancing their stability in circulation.
Acknowledgements
Financial support from National Natural Science Foundation of China (51033005, 21074121, 21090354 and 81171829), Provincial Natural Science Foundation of Anhui (KJ2011ZD04), and the Program for New Century Excellent Talents in Universities (NCET-08-0520) is gratefully acknowledged.
Notes and references
- K. Sutliff, Science, 2011, 333, 1686–1686 CrossRef.
- L. W. Seymour and A. J. Thrasher, Nat. Biotechnol., 2012, 30, 588–593 CrossRef CAS PubMed.
- W. F. Anderson, Nature, 1998, 392, 25–30 CrossRef CAS PubMed.
- L. Naldini, Science, 2009, 326, 805–806 CrossRef CAS PubMed.
- I. M. Verma and N. Somia, Nature, 1997, 389, 239–242 CrossRef CAS PubMed.
- N. Cartier, S. Hacein-Bey-Abina, C. C. Bartholomae, G. Veres, M. Schmidt, I. Kutschera, M. Vidaud, U. Abel, L. Dal-Cortivo, L. Caccavelli, N. Mahlaoui, V. Kiermer, D. Mittelstaedt, C. Bellesme, N. Lahlou, F. Lefrere, S. Blanche, M. Audit, E. Payen, P. Leboulch, B. l'Homme, P. Bougneres, C. Von Kalle, A. Fischer, M. Cavazzana-Calvo and P. Aubourg, Science, 2009, 326, 818–823 CrossRef CAS PubMed.
- A. Donsante, D. G. Miller, Y. Li, C. Vogler, E. M. Brunt, D. W. Russell and M. S. Sands, Science, 2007, 317, 477–477 CrossRef CAS PubMed.
- S. Hacein-Bey-Abina, C. Von Kalle, M. Schmidt, M. P. McCcormack, N. Wulffraat, P. Leboulch, A. Lim, C. S. Osborne, R. Pawliuk, E. Morillon, R. Sorensen, A. Forster, P. Fraser, J. I. Cohen, G. de Saint Basile, I. Alexander, U. Wintergerst, T. Frebourg, A. Aurias, D. Stoppa-Lyonnet, S. Romana, I. Radford-Weiss, F. Gross, F. Valensi, E. Delabesse, E. Macintyre, F. Sigaux, J. Soulier, L. E. Leiva, M. Wissler, C. Prinz, T. H. Rabbitts, F. Le Deist, A. Fischer and M. Cavazzana-Calvo, Science, 2003, 302, 415–419 CrossRef CAS PubMed.
- W. J. Kim and S. W. Kim, Pharm. Res., 2009, 26, 657–666 CrossRef CAS PubMed.
- J.-P. Behr, Acc. Chem. Res., 2012, 45, 980–984 CrossRef CAS PubMed.
- M. Molas, R. Bartrons and J. C. Perales, Biochim. Biophys. Acta, Gen. Subj., 2002, 1572, 37–44 CrossRef CAS.
- A. Aigner, D. Fischer, T. Merdan, C. Brus, T. Kissel and F. Czubayko, Gene Ther., 2002, 9, 1700–1707 CrossRef CAS PubMed.
- S. Kommareddy and M. Amiji, Bioconjugate Chem., 2005, 16, 1423–1432 CrossRef CAS PubMed.
- D. G. Anderson, D. M. Lynn and R. Langer, Angew. Chem., Int. Ed., 2003, 42, 3153–3158 CrossRef CAS PubMed.
- Z. H. Zhang, L. C. Yin, Y. X. Xu, R. Tong, Y. B. Lu, J. Ren and J. J. Cheng, Biomacromolecules, 2012, 13, 3456–3462 CrossRef CAS PubMed.
- C. H. Jones, C. K. Chen, M. Jiang, L. Fang, C. Cheng and B. A. Pfeifer, Mol. Pharmaceutics, 2013, 10, 1138–1145 CrossRef CAS PubMed.
- C. K. Chen, C. H. Jones, P. Mistriotis, Y. Yu, X. N. Ma, A. Ravikrishnan, M. Jiang, S. T. Andreadis, B. A. Pfeifer and C. Cheng, Biomaterials, 2013, 34, 9688–9699 CrossRef CAS PubMed.
- F. J. Verbaan, G. W. Bos, C. Oussoren, M. C. Woodle, W. E. Hennink and G. Storm, J. Drug Deliv. Sci. Tech., 2004, 14, 105–111 CAS.
- M. Harada-Shiba, K. Yamauchi, A. Harada, I. Takamisawa, K. Shimokado and K. Kataoka, Gene Ther., 2002, 9, 407–414 CrossRef CAS PubMed.
- D. Oupicky, R. C. Carlisle and L. W. Seymour, Gene Ther., 2001, 8, 713–724 CrossRef CAS PubMed.
- D. Oupicky, K. A. Howard, C. Konak, P. R. Dash, K. Ulbrich and L. W. Seymour, Bioconjugate Chem., 2000, 11, 492–501 CrossRef CAS PubMed.
- P. R. Dash, M. L. Read, L. B. Barrett, M. Wolfert and L. W. Seymour, Gene Ther., 1999, 6, 643–650 CrossRef CAS PubMed.
- C. H. Zhu, M. Zheng, F. H. Meng, F. M. Mickler, N. Ruthardt, X. L. Zhu and Z. Y. Zhong, Biomacromolecules, 2012, 13, 769–778 CrossRef CAS PubMed.
- H. Petersen, P. M. Fechner, A. L. Martin, K. Kunath, S. Stolnik, C. J. Roberts, D. Fischer, M. C. Davies and T. Kissel, Bioconjugate Chem., 2002, 13, 845–854 CrossRef CAS PubMed.
- K. Miyata, Y. Kakizawa, N. Nishiyama, A. Harada, Y. Yamasaki, H. Koyama and K. Kataoka, J. Am. Chem. Soc., 2004, 126, 2355–2361 CrossRef CAS PubMed.
- A. Meister and M. E. Anderson, Annu. Rev. Biochem., 1983, 52, 711–760 CrossRef CAS PubMed.
- C. Y. Hong, Y. Z. You, D. C. Wu, Y. Liu and C. Y. Pan, J. Am. Chem. Soc., 2007, 129, 5354–5355 CrossRef CAS PubMed.
- U. L. Rahbek, A. F. Nielsen, M. D. Dong, Y. Z. You, A. Chauchereau, D. Oupicky, F. Besenbacher, J. Kjems and K. A. Howard, J. Drug Targeting, 2010, 18, 812–820 CrossRef CAS PubMed.
- Y. Z. You, C. Y. Hong and C. Y. Pan, Adv. Funct. Mater., 2007, 17, 2470–2477 CrossRef CAS.
- Y. Z. You, D. S. Manickam, Q. H. Zhou and D. Oupicky, J. Control. Release, 2007, 122, 217–225 CrossRef CAS PubMed.
- L. V. Christensen, C. W. Chang, W. J. Kim, S. W. Kim, Z. Y. Zhong, C. Lin, J. F. J. Engbersen and J. Feijen, Bioconjugate Chem., 2006, 17, 1233–1240 CrossRef CAS PubMed.
- C. Lin, C. J. Blaauboer, M. M. Timoneda, M. C. Lok, M. van Steenbergen, W. E. Hennink, Z. Y. Zhong, J. Feijen and J. F. J. Engbersen, J. Controlled Release, 2008, 126, 166–174 CrossRef CAS PubMed.
- L. V. Christensen, C. W. Chang, J. W. Yockman, R. Conners, H. Jackson, Z. Y. Zhong, J. Feijen, D. A. Bu and S. W. Kim, J. Controlled Release, 2007, 118, 254–261 CrossRef CAS PubMed.
- Y. Wang, C. Y. Hong and C. Y. Pan, Biomacromolecules, 2013, 14, 1444–1451 CrossRef CAS PubMed.
- Z.-Q. Yu, J.-T. Sun, C.-Y. Pan and C.-Y. Hong, Chem. Commun., 2012, 48, 5623–5625 RSC.
- D.-C. Wu, X. J. Loh, Y.-L. Wu, C. L. Lay and Y. Liu, J. Am. Chem. Soc., 2010, 132, 15140–15143 CrossRef CAS PubMed.
- Z. K. Wang, L. H. Wang, J. T. Sun, L. F. Han and C. Y. Hong, Polym. Chem., 2013, 4, 1694–1699 RSC.
- J. Chen, C. Wu and D. Oupicky, Biomacromolecules, 2009, 10, 2921–2927 CrossRef CAS PubMed.
Footnote |
† Electronic supplementary information (ESI) available. See DOI: 10.1039/c3bm60204d |
|
This journal is © The Royal Society of Chemistry 2014 |