DOI:
10.1039/C3BM60166H
(Paper)
Biomater. Sci., 2014,
2, 131-138
In vitro differentiation of functional human skeletal myotubes in a defined system†
Received
2nd July 2013
, Accepted 4th September 2013
First published on 25th September 2013
Abstract
In vitro human skeletal muscle systems are valuable tools for the study of human muscular development, disease and treatment. However, published in vitro human muscle systems have so far only demonstrated limited differentiation capacities. Advanced differentiation features such as cross-striations and contractility have only been observed in co-cultures with motoneurons. Furthermore, it is commonly regarded that cultured human myotubes do not spontaneously contract, and any contraction has been considered to originate from innervation. This study developed a serum-free culture system in which human skeletal myotubes demonstrated advanced differentiation. Characterization by immunocytochemistry, electrophysiology and analysis of contractile function revealed these major features: (A) Well defined sarcomeric development, as demonstrated by the presence of cross-striations. (B) Finely developed excitation–contraction coupling apparatus characterized by the close apposition of dihydropyridine receptors on T-tubules and ryanodine receptors on sarcoplasmic reticulum membranes. (C) Spontaneous and electrically controlled contractility. This report not only demonstrates an improved level of differentiation of cultured human skeletal myotubes, but also provides the first published evidence that such myotubes are capable of spontaneous contraction. Use of this functional in vitro human skeletal muscle system would advance studies concerning human skeletal muscle development and physiology, as well as muscle-related disease and therapy.
1. Introduction
Many human diseases are related to skeletal muscle (SKM) disorders. The most common conditions are inherited muscular dystrophy1 and inflammatory myopathy,2 both characterized by progressive muscle wasting and weakness. However, muscle disorders are also associated with other diseases such as amyotrophic lateral sclerosis (ALS),3 spinal muscular atrophies4 and various metabolic diseases.5 To better understand, control and finally cure such diseases, mature and functional models of SKM are essential. While some animal models have been developed for in vivo study,6,7 modeling of such conditions in human cells is limited to in vitro systems.8
Most in vitro SKM models developed to date utilize rodent muscle tissue as the cell source and produce myotubes capable of functional contraction.9,10 In addition, combining these cells with Microelectromechanical systems (MEMs) allows muscle performance to be monitored with high resolution and efficiency, which is important for drug screening.11 However, the species gap between rodent and human limits the application of such technology in disease investigation and drug screening. Therefore, human-based in vitro SKM systems are desirable.
Multiple human SKM culture systems have been developed that usually make use of satellite cells isolated from healthy donors or individuals with relevant diseases. The conditions for proliferating these muscle precursor cells are well established12–15 but their differentiation (satellite cells differentiate into myocytes which then fuse to form multi-nucleated myotubes) has, for the most part, been studied and described using serum-containing media systems,16–21 although serum-free media systems have also been explored.15 Human myotubes developed using these methods have been used in a plethora of applications such as the study of muscle physiology,16 development and innervation,20–23 etiological investigation and therapeutic design for muscle-related diseases.17,24–26 They have also been utilized in tissue-engineering experiments aimed at regenerative medicine transplantation15,27 and high throughput drug screening.28 However, in contrast to avian and rodent muscle cultures, published methods using human muscle, cultured aneurally in a monolayer, have yet to demonstrate advanced morphological development and contractile capability.29
Distinct sarcomeric patterning (cross-striation), and well-developed transverse (T)-tubules, the basic apparatus for muscle contraction and excitation–contraction coupling (ECC) respectively, have only been demonstrated in human myotubes co-cultured with rat spinal cord explants29 or human spinal neurons.14 In addition, despite being observed frequently in rodent SKM cultures, spontaneous contraction has not been reported for aneurally cultured human SKM.30 This observation has led to the assertion that human SKM myotubes are incapable of spontaneous contractile function unless innervated by an appropriate neuronal input.13,20,30,31 In addition, functional myofibers that can contract in response to electrical stimulation has not been reported in cultured hSKMs. To respond to the increasing demand for functional human muscle systems, we aimed to establish and validate a defined and functional in vitro human muscle culture system capable of promoting the advanced maturation of human myotubes.
2. Results
2.1. Myofiber differentiation observed by phase microscopy
The procedure for the differentiation of the SKM myotubes is described in detail in the Materials and methods section of this manuscript. Briefly, human SKM stem cells/progenitors were grown to confluence before differentiation induction (Fig. 1Ba). After switching to differentiation medium 1, myocyte fusion was initiated and multi-nuclear myotubes formed gradually in the culture (Fig. 1Bb). After a week of differentiation, the medium was switched to differentiation medium 2. If continuously left in dif 1, these myotubes will detach within a couple of days which leaves very little time for further maturation. After being switched to dif 2, the full maturation of the hSKMs was evident after one week in this composition (Fig. 1Bc) and myotubes exhibiting striated band patterns were observed by day 10 (Fig. 1Bd). This characteristic anisotropic/isotropic band patterning is due to the differential light diffraction of the organized myofibrial proteins that form sarcomeres within the myotubes and is observed in mature muscle fibers in vivo.32,33 The striated patterns indicated the formation of organized sarcomeres, the basic contractile apparatus of SKM, implying that these myofibers were structurally capable of contraction.
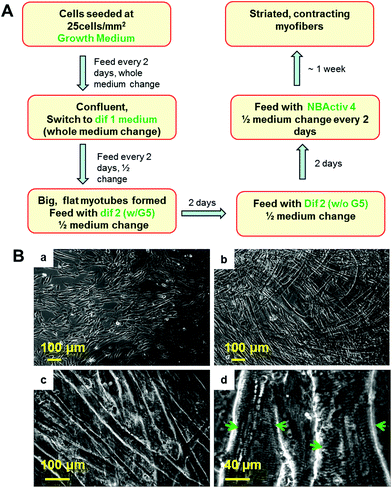 |
| Fig. 1 (A) Schematic diagram of differentiation protocol. (B) Formation and differentiation of myofibers in culture demonstrated by phase microscopy. (a) Before differentiation in growth phase. (b) Day 4 in differentiation medium 1. (c) Day 16 in differentiation medium 2. (d) Day 16 in differentiation medium 2 under higher magnification. Arrows in (d) indicate striated band patterns on differentiated myotubes. | |
Adjacent myotubes tended to align with the same orientation within these cultures, thus forming different “patterns” of myofiber clusters in the culture. This phenomenon is reminiscent of native SKM structure which is composed of many aligned myofibers that are bundled to form muscle fascicles.
2.2. Cross-striation demonstrated by immunostaining
Actin and myosin are two major proteins of the myofibril and their interaction, in response to Ca2+ influx, facilitates the power stoke of the sarcomere, thereby enabling muscle fiber contraction. Fig. 2 highlights the striated pattern of F-actin (stained using phalloidin) and MHC within myotubes cultured using the described protocol. This pattern is characteristic of actin–myosin banding in muscle fibers in vivo and indicates the potential for contraction in these in vitro fibers. Staining with phalloidin and MHC antibodies demonstrated a partial overlap (yellow), possibly indicating anisotropic bands. The non-overlapped regions mainly stained positive for actin (red), presumably corresponding to the isotropic band. Quantification based on phalloidin/MHC staining indicated that 5.6 ± 3.18% (N = 3 coverslips from 2 individual experiments) of the myotubes demonstrated a striated morphology.
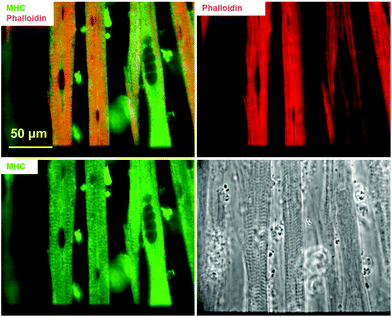 |
| Fig. 2 Immunostaining of human myotubes with myosin heavy chain (MHC) antibody F1.652 and phalloidin. The culture was 10 days after in differentiation medium 2. Myotube striation was demonstrated by both staining as well as utilizing phase microscopy. | |
2.3. Excitation contraction coupling apparatus demonstrated by immunostaining
Excitation–contraction-coupling (ECC) is a fundamental process in muscle tissue and constitutes the transformation of action potentials, propagated along myofiber membranes, into physical myotube contraction. This process is achieved through multiple steps: the propagation of membrane depolarization from sarcolemma into the T-tubule system which activates dihydropyridine receptors (DHPR), activated DHPRs then activate type 1 ryanodine receptors (RyR) located on the sarcoplasmic membrane allosterically via mechanical linkage. The opening of RyRs allows the release of Ca2+ into the cytoplasm and initiates the sliding of actin and MHC, which causes contraction. This process depends on the expression and close apposition of two calcium channels: DHPR on T-tubules and RyR on the closely apposed sarcoplasmic reticulum membrane.34,35 To investigate the development of the ECC apparatus in the SKM myotubes developed in this system, the presence of DHPR and RyR, as well as their co-localization, was analyzed by immunocytochemistry. Fig. 3 clearly illustrates both the abundant expression of these proteins, as well as their close apposition within the cultured myotubes. The observed banding (Fig. 3, arrow) highlights the close association of these two channels and suggests the formation of the triad structure that is typical of in vivo myofibers.
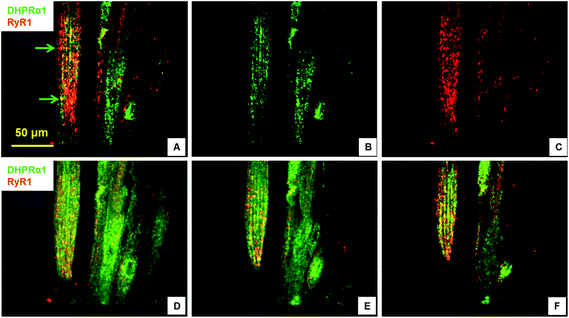 |
| Fig. 3 Co-immunostaining with DHPRα1 and RyR1 displaying the excitation and contraction coupling (ECC) apparatus. The culture was 17 days in differentiation medium 2. (A–C) Compiled confocal images. Arrows in (A) indicated co-localization of DHPR α1 with RyR1 and their striated patterns. (D–E) Examples of optical sections from the confocal image in (A). | |
2.4. Electrophysiology of cultured myotubes
The electrophysiological competence of the differentiated myotubes was evaluated using patch clamp recordings. The maintenance of a stable resting membrane potential (RP) is indicative of the correct functioning of multiple ion channels on the myotube membrane, primarily potassium and chloride channels, as well as Na+/K+ ATPase pumps. It has been reported that myotubes which are not fully differentiated have a less-hyperpolarized RP.21 The most hyperpolarized RP reported to date for cultured human SKM was achieved with a serum containing medium (−57 ± 9 mV).21 RP analysis from our culture model indicated that the myotubes generally developed a highly hyperpolarized resting potential (−56.35 ± 4.65 mV, N = 25) (Table 2). This result indicates that this serum-free culture system is comparable to the best results achieved for serum-containing medium systems in facilitating RP development of human SKM in vitro. The voltage clamp electrophysiology of the myotubes indicated the inward and outward currents characteristic of functional sodium and potassium channels (Fig. 4A, Table 2). Fig. 4B provides an example of a single action potential fired by cultured myotubes in current clamp mode. Taken together, these results indicate that the electrical properties of the cultured cells were consistent with the development of mature, electrophysiologically-competent myotubes.
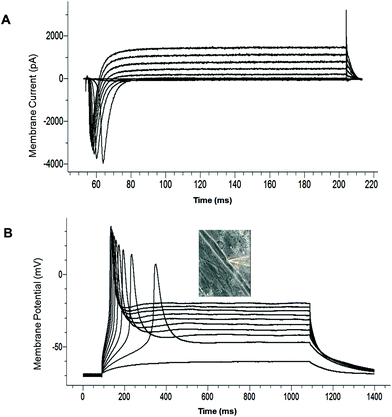 |
| Fig. 4 Patch clamp recordings demonstrating the electrophysiology properties of differentiated myotubes. (A) Example traces of a voltage-clamp recording. (B) Example traces of a current-clamp recording. The inset is a picture of the recorded cell. | |
Table 1 Composition and concentrations of differentiation media 1 and 2
Component |
Concentration |
Company |
Catalog number |
Differentiation medium 1
|
DMEM |
|
Invitrogen |
11995-040 |
Insulin |
10 μg ml−1 |
Sigma |
I 9278 |
BSA |
500 μg ml−1 |
Sigma |
A9418 |
EGF |
10 ng ml−1 |
Invitrogen |
13247-051 |
Gentamicin |
50 μg ml−1 |
Sigma |
G1272 |
Differentiation medium 2
|
Neurobasal A |
|
Invitrogen |
21103 |
B27 (50×) |
1× |
Invitrogen |
17504-044 |
Glutamax (100×) |
1× |
Invitrogen |
35050 |
GDNF |
10 ng ml−1 |
Cell Sciences |
CRG400B |
BDNF |
20 ng ml−1 |
Cell Sciences |
CRB600B |
Shh |
50 ng ml−1 |
R&D |
1845-SH-025 |
Retinoic acid |
0.1 μM |
Sigma |
R2625 |
IGF-1 |
10 ng ml−1 |
PeproTech |
100-11 |
cAMP |
1 μM |
Sigma |
A9501 |
CNTF |
5 ng ml−1 |
Cell Sciences |
CRC400A |
Neurotrophin-3 |
20 ng ml−1 |
Cell Sciences |
CRN500B |
Neurotrophin-4 |
20 ng ml−1 |
Cell Sciences |
CRN501B |
Vitronectin |
100 ng ml−1 |
Sigma |
V8379 |
Mouse laminin |
4 μg ml−1 |
Invitrogen |
23017-015 |
G5 (100×) |
1× |
Invitrogen |
17503-012 |
Agrin |
100 ng ml−1 |
R&D |
550-AG-100 |
Table 2 Electrophysiological properties of hSKM myotubes in the culture after 10–20 days in differentiation media 2
Parameters |
Average |
STDEV (N) |
Membrane resistance (MΩ) |
81.97 |
52.70 (25) |
Membrane capacitance (pF) |
184.57 |
95.84 (25) |
Resting membrane potential (mV) |
−56.35 |
4.65 (25) |
Na+ current (pA) |
−4352.93 |
1999.80 (24) |
K+ current (pA) |
1713.15 |
753.93 (24) |
Action potential (mV) |
105.87 |
18.98 (25) |
2.5. Spontaneous contraction of human myotubes in culture
To date, spontaneous contraction has not been reported from human SKM cells in vitro. This is a critical issue since contraction has been typically used as the primary indicator of functional synapse formation in cultured human muscle cells. To determine whether hSKM in our system could spontaneously contract, hSKM cultures were analyzed using phase microscopy. Occasionally, myotube contractions were observed directly after removal from the incubator; however, all contractions stopped within seconds and did not resume if left on the microscope stage. In order to ascertain whether this phenomenon was caused by the ambient temperature and/or uncontrolled CO2 levels outside of the incubator, cultures were then monitored on a time-lapse microscope with controlled temperature (37 °C) and CO2 (5%). Areas exhibiting sporadic spontaneous contractions (“contraction spots”), were observed under these conditions and the general occurrence rate of contraction spots did not alter over time.
A contraction spot was defined as a distinct group of differentiated myotube(s) contracting simultaneously at a frequency different from surrounding myotubes (Videos 1, 2, 3, ESI†). The contraction of each spot lasted various lengths of time from seconds to minutes. Across all the coverslips analyzed, 7 out of 9 coverslips cultured produced contraction spots. Each coverslip, even those from the same culture, presented different numbers of contraction spots with variations ranging from 2 to 24 (8.4 ± 10.4). Since agrin has been found as an important nerve-derived factor for muscle differentiation,20,21 it was omitted in some cultures to test its effect for the development of spontaneous function. A slightly smaller number of coverslips (7 out of 12) demonstrated contraction spots. Similar to the results discussed above, the numbers of contraction spots on each coverslip showed considerable variation (11.8 ± 18.0) and the average number was not significantly different from cultures maintained in the presence of agrin.
Crucially, and for the first time, the collected data demonstrate that human SKM is capable of spontaneous contractions in vitro. It is likely that these spontaneous contractions were not observed previously due to either the inferior maturity of the myotubes or the human myotube's high sensitivity to ambient environmental conditions.
2.6. Stimulated contraction of human myotubes in culture
The major function of muscle in vivo is to contract in response to nervous excitation. Although the collected data provided strong evidence for the structural basis of ECC in vitro, it is still unknown whether these myotubes were capable of contraction in response to controlled electrical stimulation. To address this question, hSKM cultures were mounted on the stage of an upright microscope and stimulated to induce contraction. Field stimulations (2 V, duration 40 ms, square wave) with various frequencies (0.25–1 Hz) were applied and the contractions of myotubes were recorded by videotape. As illustrated in Videos 4, 5 (ESI†), myotubes not only contracted in response to stimulation, but also contracted synchronously with the stimulation pulses. These observations provide further evidence for the high level of functional maturation achieved by myotubes cultured using the described protocol.
3. Discussion
This work details the characterization of a de novo in vitro system that facilitated the generation of functional human skeletal myotubes derived from human SKM stem cells. The advantages of this system are 2-fold: (1) the contractile functionality of the cultured myotubes, as evidenced by their advanced morphological features, including cross-striation and triad organization as well as direct observation of myotube contraction (both spontaneous and stimulated). (2) The culture model utilized serum-free media, allowing the system to be highly controlled and easily reproducible, as well as facilitating a more complete interrogation of cellular maturation and function in vitro.
The myotubes in the presented culture system exhibited an advanced level of functional maturation, highlighted by the capability of spontaneous and electrically controlled contraction, which no previously published aneural human muscle culture has provided. Comparison of our differentiation protocol to all previous reports indicates two primary modifications. First, in addition to the basic differentiation step, during which myocytes fuse and form multi-nuclear myotubes as all previous studies had shown, a second step was added incorporating a second differentiation medium. Increasing the time of culture in differentiation medium 1 without differentiation medium 2 did not generate contracting myotubes (data not shown), indicating the necessity of differentiation medium 2 for achieving mature contractile myotubes in vitro. This medium (Table 1) includes a series of trophic factors for both myotubes and neurons since it was originally developed in our laboratory for the co-culture of human SKM with human motoneurons.14 However, most of the components in this medium have also been shown to be important for the development and function of muscle.
Agrin is a nerve-derived factor that can induce post-synaptic differentiation36 and has been found to be able to promote the development of a more negative resting membrane potential,21 as well as facilitating the maturation of ECC mechanisms in cultured human myotubes.20 In addition, the laminins, a major family of glycoproteins that constitute essential components of the muscle basement membrane (a layer of extracellular matrix surrounding myofibers), are found to be important for myogenesis, maintenance and regeneration.37 Deficiencies in specific laminins, or interference in their signaling pathways during the development of myofibers and their internal cytoskeleton, can produce muscle disease phenotypes.38 For example, mutations in the laminin α 2 gene can cause congenital muscular dystrophy.39 The neurotrophins BDNF, GDNF, NT3/4, CNTF and IGF 1 have recently been shown to be important regulators of the development, maintenance, function, and regeneration of SKM fibers.40,41 Skeletal muscles not only act as an abundant source of neurotrophic support during development, but also express the appropriate receptors to facilitate their regulation in response to these factors.41,42 Sonic Hedgehog is found to be a cell-autonomous factor for the survival, myogenesis, and migration of muscle precursors during development.43,44 Similarly, retinoic acid is known to enhance myogenesis.45 In general, all the factors included in our differentiation medium 2 have demonstrated some positive effect on muscle differentiation, maturation and/or function, which may explain why the myotubes in this study developed more mature characteristics.
The second difference, as already stated, is that our media compositions do not contain serum while most previous studies used fetal bovine serum (FBS) supplementation in basal media such as aMEM, DMEM or F-14.16–21 Inclusion of FBS in differentiation medium 2 inhibited the occurrence of spontaneous contraction, but did not prevent the development of advanced morphology such as cross striations and T-tubule formation (data not shown). Consequentially, elimination of serum could only partially account for the differences observed in myotube maturation levels. This suggests therefore, that the use of differentiation medium 2 was the major causal factor for the advanced myotube differentiation demonstrated by our system. However, it also suggests that serum not only contains factors for growth and survival, but also inhibitory factors that can halt differentiation at the level of development present at the time the serum was collected. Thus, removal of serum eliminates conflicting signals in culture, to allow full maturation of the seeded cells. This observation is in line with our previous work relating to the use of serum-free media for motoneuron myelination in vitro.46
Spontaneous excitatory activity observed in neurons and muscle cells has been found to be essential for their functional maturation during development.47,48 Spontaneous neuronal activity has been observed extensively during early development in multiple tissues such as cortical,49 hippocampal,50 cerebral cortex,51 cerebellum, hindbrain, spinal cord and dorsal root ganglia, in species ranging from insects and amphibians to mammals.47 These activities are essential for many early events of neuronal maturation, including the growth of dendritic spines and axons, ion channel development, and establishment and refinement of neural circuits.52 Similarly, spontaneous contractile activity has been observed in the developing muscles of chicks,53,54 mice55 and rats.18 Additionally, the development and pruning of certain ion channels within these muscles, such as Na+ channels, Cl− channels, and Ach receptor/channels have been shown to be activity-dependent.18,56–58 Furthermore, it has been demonstrated that contractile activity is required for the expression of neonatal myosin heavy chain in embryonic chick muscle53,54 and the expression of MyoD and myogenin as well as sarcomere formation in rat myotubes.18,59 However, due to the scarce evidence for spontaneous contractions of human muscle during development, it is unknown whether the above referenced findings from other systems can be directly translated into human muscle system. Nevertheless, our findings that hSKM do spontaneously contract even without neuronal input calls for caution when using contraction as the sole evidence of innervation in future human NMJ studies.
This functional in vitro human muscle system could provide a powerful tool for research in multiple fields. It offers a valuable platform from which to investigate human muscle development and physiology, muscle-related diseases and pathology while being potentially compatible with future high throughput drug development and toxicology investigations. The defined nature of the culture protocol will facilitate the application of this system in mechanism dissection and enhances its reproducibility. The contractility of these cultured human myotubes also significantly expands the scope of their application in fields of study focused on muscle kinetics, metabolism, and sports-related muscle physiology. The media system developed in this study represents a means to achieve highly mature and functionally competent myotubes which may be of considerable benefit to tissue-engineering efforts related to skeletal muscle. These functional myotubes can also be co-cultured with motoneurons in order to study NMJ-related human physiology and etiology.14
4. Methods
4.1. DETA surface modification
Glass coverslips (6661F52, 22 × 22 mm no. 1; Thomas Scientific, Swedesboro, NJ, USA) were cleaned using HCl–methanol (1
:
1) for at least 2 hours, rinsed with deionized water, soaked in concentrated H2SO4 for at least 2 hours and rinsed with deionized water. Coverslips were boiled in nanopure water and then oven dried. The trimethoxysilylpropyldiethylenetri-amine (DETA, T2910KG; United Chemical Technologies Inc., Bristol, PA, USA) film was formed by the reaction of cleaned surfaces with a 0.1% (v/v) mixture of the organosilane in freshly distilled toluene (T2904; Fisher, Suwanne, GA, USA). The DETA coated coverslips were heated to 80 °C, then cooled to room temperature (RT), rinsed with toluene, reheated to approximately the same temperature, and then cured for at least 2 hours at 110 °C. Surfaces were characterized by contact angle and X-ray photoelectron spectroscopy as described previously.60–64 DETA's triamine functionality has a structural analog in spermidine, a known growth factor, which is a possible reason for its success as a culture substrate.63,64
4.2. Isolation of human skeletal muscle stem cells
Human SKM stem cells (hSKM SCs)/progenitors, or satellite cells, were isolated, proliferated and differentiated as described previously.15 Briefly, primary human SKM cells were isolated by needle biopsy65 and were expanded in myoblast growth medium (MGM; SkGM (Cambrex Bio Science, Walkersville, MD) plus 15% (v/v) fetal bovine serum). Biopsies were performed on adult volunteers according to procedures approved by the Institutional Clinical Review Board of the Miriam Hospital. Cell preparations on average were 70% myogenic based on desmin-positive staining.66
4.3. Cell culture – differentiation of functional myofibers
For each culture, hSKM SCs/progenitors were plated on DETA coverslips at a density of 25 cells per mm2 in hSKM growth medium (Lonza Inc., Allendale, NJ, Cat# CC-3160) and fed every 2 days by changing the whole medium. On day 7, myoblast fusion was induced by switching to differentiation medium 1 (high-glucose DMEM (Invitrogen, Carlsbad, CA) supplemented with insulin (10 μg ml−1), bovine serum albumin (BSA) (500 μg ml−1), epidermal growth factor (10 ng ml−1) and gentamicin (50 μg ml−1)). The cells were fed every 2 days by changing half of the medium. On day 7 after differentiation, the medium was changed to differentiation medium 2 (with G5) (Table 1). Two days later, cells were fed with differentiation medium 2 (without G5) by changing half of the medium. Thereafter, the cultures were fed every two days by replacing half of the medium with fresh NBactive4 (BrainBits, LLC, Springfield, IL).
4.4. Immunocytochemistry
Cells on DETA coverslips were fixed in freshly prepared 4% paraformaldehyde in phosphate buffered saline (PBS) (pH 7.2, without Mg2+, Ca2+) for 15 minutes. Cells were then washed twice with PBS for 10 minutes at room temperature and then permeabilized with 0.1% Triton X-100/PBS for 15 minutes. Non-specific binding sites were blocked using blocking buffer (5% donkey serum plus 0.5% BSA in PBS) for 45 minutes at room temperature. Cells were incubated with primary antibodies overnight at 4 °C. After being washed three times with PBS for 10 minutes, the cells were incubated with secondary antibodies for 2.5 hours at room temperature. The cells were then washed three times with PBS for 10 minutes and mounted using Vectashield (Vector laboratories, Inc., Burlingame, CA). Primary antibodies used in this study were: mouse-anti-myosin heavy chain (MHC, F1.625, 1
:
10), mouse-anti-DHPRα1 (dihydropyridine receptor α1, Millipore, Billerica, MA, 1
:
500), rabbit-anti-ryanodine receptor (Millipore, 1
:
500). Phalloidin-568 (Invitrogen, 1
:
200) was added to the secondary antibody solutions in order to facilitate actin filament visualization. The monoclonal antibody against myosin heavy chain (MHC, F1.625, 1
:
10) was obtained from the Developmental Studies Hybridoma Bank which is under the auspices of the National Institute of Child Health and Human Development (NICHD) and maintained by the University of Iowa. Secondary antibodies used in this study were: donkey-anti-mouse-488 (Invitrogen, 1
:
250) and donkey-anti-rabbit-594 (Invitrogen, 1
:
250). All antibodies were diluted in blocking buffer.
4.5. Electrophysiology
Electrophysiological properties of human myotubes were investigated after ∼10 days in differentiation medium 2 using whole-cell patch-clamp recording techniques.61 The recordings were performed in a recording chamber located on the stage of a Zeiss Axioscope 2FS Plus upright microscope.67
Recordings were made from striated, multi-nucleated myotubes. Patch pipettes with a resistance of 6–10 MΩ were made from borosilicate glass (BF 150-86-10; Sutter Instrument Company, Novato, CA) with a Sutter P97 pipette puller (Sutter Instrument Company). Current-clamp and voltage-clamp recordings were made utilizing a Multiclamp 700A amplifier (Axon, Union City, CA). The pipette (intracellular) solution contained 140 mM K-gluconate, 2 mM MgCl2, 2 mM Na2ATP and 10 mM HEPES (pH 7.2). After the formation of a gigaohm seal and membrane puncture, the cell capacitance was compensated. The series resistance was typically <23 MΩ, and it was compensated >60% using the amplifier circuitry. Signals were filtered at 3 kHz and sampled at 20 kHz using a Digidata 1322A interface (Axon instrument). Data recording and analysis were performed with pClamp8 software (Axon instrument). Membrane potentials were corrected by subtraction of a 15 mV tip potential, which was calculated using Axon's pClamp8 program. Membrane resistance and capacitance were calculated using 50 ms voltage steps from −85 to −95 mV without any whole-cell or series resistance compensation. The resting membrane potential and depolarization-evoked action potentials were recorded in current-clamp mode. Depolarization-evoked inward and outward currents were examined in voltage-clamp mode.
4.6. Monitoring myotube contraction by phase microscopy and videography
To monitor spontaneous myotube contractions in vitro, the hSKM cultures were placed in an insulated temperature controlled chamber (Okalabs) in a 6-well plate, following 9 days in differentiation medium 2. The cells were maintained in NBActiv4 medium at 37 °C, in 5.0% CO2 and 95% (or higher) humidity. Spontaneous myotube contraction was monitored and imaged on the stage of a Zeiss Axiovert microscope 200. The videos were recorded utilizing a Hamamatsu digital camera (Model C8484-05G) at a frame rate of 8 frames per s using Windows Movie Maker software.
To stimulate and observe myotube contraction, a system was assembled around an upright Olympus BX51WI electrophysiology microscope (Olympus Inc., Center Valley, PA). A coverslip with differentiated myotubes was placed inside a 35 mm dish containing NBActiv4 medium. The dish was mounted on the stage of the microscope and two silver wires were affixed parallel to each other with a separation distance of 15 mm inside the dish. The silver wires were capable of producing field stimulation pulses of varying intensities, frequencies, and waveforms. Myotube contractions were recorded with the Pinnacle Studio software (version 9.0) from Pinnacle System Inc.
5. Conclusions
In summary, this study presents an in vitro human SKM model with functionally contracting myotubes. These myotubes reached an advanced level of differentiation demonstrated by the attainment of cross-striation, T-tubule formation and contractility. This work also provides the first evidence that human myotubes in vitro are capable of spontaneous contraction. This defined, human-based, in vitro muscle system has great and wide reaching potential applications in the fields of tissue engineering, regenerative medicine, high content drug screening and the investigation of muscle physiology and disease.
This research was funded by NIH/NINDS grant R01NS050452 and NIH/NIBIB grant R01EB009429. The authors confirm that no competing financial interests exist and there has been no financial support for this research that could have influenced its outcome.
Notes and references
- P. T. Martin, Nat. Clin. Pract. Neurol., 2006, 2, 222–230 CrossRef CAS PubMed.
- J. N. Whitaker, Muscle Nerve, 1982, 5, 573–592 CrossRef CAS PubMed.
- L. Dupuis and A. Echaniz-Laguna, Curr. Drug Targets, 2010, 11, 1250–1261 CrossRef CAS PubMed.
- M. Stavarachi, P. Apostol, M. Toma, D. Cimponeriu and L. Gavrila, J. Med. Life, 2010, 3, 3–9 CAS.
- C. Angelini and C. Semplicini, Curr. Opin. Pharmacol., 2010, 10, 338–345 CrossRef CAS PubMed.
- A. Nakamura and S. Takeda, J. Biomed. Biotechnol., 2011, 2011, 184393 Search PubMed.
- C. A. Collins and J. E. Morgan, Int. J. Exp. Pathol., 2003, 84, 165–172 CrossRef CAS PubMed.
- H. Vandenburgh, Tissue Eng., Part B, 2010, 16, 55–64 CrossRef CAS PubMed.
- M. Das, K. Wilson, P. Molnar and J. J. Hickman, Nat. Protocols, 2007, 2, 1795–1801 CAS.
- M. Das, J. W. Rumsey, N. Bhargava, M. Stancescu and J. J. Hickman, Biomaterials, 2009, 30, 5392–5402 CrossRef CAS PubMed.
- K. Wilson, M. Das, K. J. Wahl, R. J. Colton and J. Hickman, PLoS One, 2010, 5, e11042 Search PubMed.
- D. Eberli, S. Soker, A. Atala and J. J. Yoo, Methods, 2009, 47, 98–103 CrossRef CAS PubMed.
- T. Mars, K. J. Yu, X.-M. Tang, A. F. Miranda, Z. Grubic, F. Cambi and M. P. King, J. Comp. Neurol., 2001, 438, 239–251 CrossRef CAS PubMed.
- X. Guo, M. Gonzalez, M. Stancescu, H. H. Vandenburgh and J. J. Hickman, Biomaterials, 2011, 32, 9602–9611 CrossRef CAS PubMed.
- L. Thorrez, J. Shansky, L. Wang, L. Fast, T. VandenDriessche, M. Chuah, D. Mooney and H. Vandenburgh, Biomaterials, 2008, 29, 75–84 CrossRef CAS PubMed.
- N. Nikolić, S. Skaret Bakke, E. Tranheim Kase, I. Rudberg, I. Flo Halle, A. C. Rustan, G. H. Thoresen and V. Aas, PLoS One, 2012, 7, e33203 Search PubMed.
- E. Palma, M. Inghilleri, L. Conti, C. Deflorio, V. Frasca, A. Manteca, F. Pichiorri, C. Roseti, G. Torchia, C. Limatola, F. Grassi and R. Miledi, Proc. Natl. Acad. Sci. U. S. A., 2011, 108, 20184–20188 CrossRef CAS PubMed.
- E. K. Dutton, A. M. Simon and S. J. Burden, Proc. Natl. Acad. Sci. U. S. A., 1993, 90, 2040–2044 CrossRef CAS.
- N. Gajsek, M. Jevsek, T. Mars, K. Mis, S. Pirkmajer, J. Brecelj and Z. Grubic, Chem.-Biol. Interact., 2008, 175, 50–57 CrossRef CAS PubMed.
- E. Bandi, M. Jevsek, T. Mars, M. Jurdana, E. Formaggio, M. Sciancalepore, G. Fumagalli, Z. Grubic, F. Ruzzier and P. Lorenzon, Am. J. Physiol.: Cell Physiol., 2008, 294, C66–C73 CrossRef CAS PubMed.
- M. Jurdana, G. Fumagalli, Z. Grubic, P. Lorenzon, T. Mars and M. Sciancalepore, Cell. Mol. Neurobiol., 2009, 29, 123–131 CrossRef CAS PubMed.
- T. Mars, M. P. King, A. F. Miranda, W. F. Walker, K. Mis and Z. Grubic, Neuroscience, 2003, 118, 87–97 CrossRef CAS PubMed.
- C. Jacobson, D. Duggan and G. Fischbach, Proc. Natl. Acad. Sci. U. S. A., 2004, 101, 12218–12223 CrossRef CAS PubMed.
- P. F. Pradat, A. Barani, J. Wanschitz, O. Dubourg, A. Lombes, A. Bigot, V. Mouly, G. Bruneteau, F. Salachas, T. Lenglet, V. Meininger and G. Butler-Browne, Amyotroph. Lateral Scler., 2011, 12, 264–271 CrossRef CAS PubMed.
- K. Mis, T. Mars, M. Golicnik, M. Jevsek and Z. Grubic, J. Mol. Neurosci., 2006, 30, 31–32 CrossRef CAS PubMed.
- S. Braun, B. Croizat, M. C. Lagrange, J. M. Warter and P. Poindron, Lancet, 1995, 345, 694–695 CrossRef CAS.
- C. A. Powell, B. L. Smiley, J. Mills and H. H. Vandenburgh, Am. J. Physiol.: Cell Physiol., 2002, 283, C1557–C1565 CrossRef CAS PubMed.
- H. Vandenburgh, J. Shansky, F. Benesch-Lee, K. Skelly, J. M. Spinazzola, Y. Saponjian and B. S. Tseng, FASEB J., 2009, 23, 3325–3334 CrossRef CAS PubMed.
- V. Askanas, H. Kwan, R. B. Alvarez, W. K. Engel, T. Kobayashi, A. Martinuzzi and E. F. Hawkins, J. Neurocytol., 1987, 16, 523–537 CrossRef CAS PubMed.
- Z. Grubic, R. Komel, W. F. Walker and A. F. Miranda, Neuron, 1995, 14, 317–327 CrossRef CAS PubMed.
- N. Gajsek, M. Jevsek and Z. Grubic, J. Mol. Neurosci., 2006, 30, 27–28 CrossRef CAS PubMed.
- B. E. Flucher, J. L. Phillips, J. A. Powell, S. B. Andrews and M. P. Daniels, Dev. Biol., 1992, 150, 266–280 CrossRef CAS PubMed.
- A. Kontrogianni-Konstantopoulos, M. A. Ackermann, A. L. Bowman, S. V. Yap and R. J. Bloch, Physiol. Rev., 2009, 89, 1217–1267 CrossRef CAS PubMed.
- B. E. Flucher, S. B. Andrews and M. P. Daniels, Mol. Biol. Cell, 1994, 5, 1105–1118 CrossRef CAS PubMed.
- B. E. Flucher, M. Terasaki, H. Chin, T. J. Beeler and M. P. Daniels, Dev. Biol., 1991, 145, 77–90 CrossRef CAS PubMed.
- S. J. Burden, J. Neurobiol., 2002, 53, 501–511 CrossRef CAS PubMed.
- J. R. Sanes, J. Biol. Chem., 2003, 278, 12601–12604 CrossRef CAS PubMed.
- B. L. Patton, Microsc. Res. Tech., 2000, 51, 247–261 CrossRef CAS PubMed.
- A. Helbling-Leclerc, X. Zhang, H. Topaloglu, C. Cruaud, F. Tesson, J. Weissenbach, F. M. Tome, K. Schwartz, M. Fardeau and K. Tryggvason,
et al.
, Nat. Genet., 1995, 11, 216–218 CrossRef CAS PubMed.
- K. Sakuma and A. Yamaquchi, J. Biomed. Biotechnol., 2011, 1–12 CrossRef PubMed.
- G. Chevrel, R. Hohlfeld and M. Sendtner, Muscle Nerve, 2006, 33, 462–476 CrossRef CAS PubMed.
- E. V. Pitts, S. Potluri, D. M. Hess and R. J. Balice-Gordon, Int. Anesthesiol. Clin., 2006, 44, 21–76 CrossRef PubMed.
- J. K.-H. Hu, E. McGlinn, B. D. Harfe, G. Kardon and C. J. Tabin, Genes Dev., 2012, 26, 2088–2102 CrossRef CAS PubMed.
- C. Anderson, V. C. Williams, B. Moyon, P. Daubas, S. Tajbakhsh, M. E. Buckingham, T. Shiroishi, S. M. Hughes and A.-G. Borycki, Genes Dev., 2012, 26, 2103–2117 CrossRef CAS PubMed.
- C. Krueger and F. M. Hoffmann, PLoS One, 2010, 5, e15511 CAS.
- J. W. Rumsey, M. Das, M. Stancescu, M. Bott, C. Fernandez-Valle and J. J. Hickman, Biomaterials, 2009, 30, 3567–3572 CrossRef CAS PubMed.
- W. J. Moody and M. M. Bosma, Physiol. Rev., 2005, 85, 883–941 CrossRef CAS PubMed.
- J. M. William, J. Neurobiol., 1998, 37, 97–109 CrossRef.
- L. Galli and L. Maffei, Science, 1988, 242, 90–91 CAS.
- L. Menendez de la Prida and J. V. Sanchez-Andres, Neuroscience, 2000, 97, 227–241 CrossRef CAS PubMed.
- A. K. McCabe, S. L. Chisholm, H. L. Picken-Bahrey and W. J. Moody, J. Physiol., 2006, 577, 155–167 CrossRef CAS PubMed.
- C. Shatz and M. Stryker, Science, 1988, 242, 87–89 CAS.
- E. Bandman and R. C. Strohman, J. Cell Biol., 1982, 93, 698–704 CrossRef CAS PubMed.
- L. C. Cerny and E. Bandman, J. Cell Biol., 1986, 103, 2153–2161 CrossRef CAS PubMed.
- K. J. M. Boonen, K. Y. Rosaria-Chak, F. P. T. Baaijens, D. W. J. van der Schaft and M. J. Post, Am. J. Physiol.: Cell Physiol., 2009, 296, C1338–C1345 CrossRef CAS PubMed.
- A. Klarsfeld, R. Laufer, B. Fontaine, A. Devillers Thiéry, C. Dubreuil and J. P. Changeux, Neuron, 1989, 2, 1229–1236 CrossRef CAS PubMed.
- R. D. Heathcote, J. Physiol., 1989, 414, 473–497 CrossRef CAS.
- J. Offord and W. A. Catterall, Neuron, 1989, 2, 1447–1452 CrossRef CAS PubMed.
- P. G. De Deyne, Am. J. Physiol.: Cell Physiol., 2000, 279, C1801–C1811 CAS.
- M. Das, N. Bhargava, C. Gregory, L. Riedel, P. Molnar and J. J. Hickman, In Vitro Cell Dev. Biol. Anim., 2005, 41, 343–348 CrossRef CAS PubMed.
- M. Das, P. Molnar, H. Devaraj, M. Poeta and J. J. Hickman, Biotechnol. Prog., 2003, 19, 1756–1761 CrossRef CAS PubMed.
- M. Das, J. W. Rumsey, C. A. Gregory, N. Bhargava, J. F. Kang, P. Molnar, L. Riedel, X. Guo and J. J. Hickman, Neuroscience, 2007, 146, 481–488 CrossRef CAS PubMed.
- M. Kaeberlein, Nat. Cell Biol., 2009, 11, 1277–1278 CrossRef CAS PubMed.
- T. Eisenberg, H. Knauer, A. Schauer, S. Buttner, C. Ruckenstuhl, D. Carmona-Gutierrez, J. Ring, S. Schroeder, C. Magnes, L. Antonacci, H. Fussi, L. Deszcz, R. Hartl, E. Schraml, A. Criollo, E. Megalou, D. Weiskopf, P. Laun, G. Heeren, M. Breitenbach, B. Grubeck-Loebenstein, E. Herker, B. Fahrenkrog, K.-U. Frohlich, F. Sinner, N. Tavernarakis, N. Minois, G. Kroemer and F. Madeo, Nat. Cell Biol., 2009, 11, 1305–1314 CrossRef CAS PubMed.
- J. V. Hennessey, J. A. Chromiak, S. Dellaventura, J. Guertin and D. B. Maclean, J. Appl. Physiol., 1997, 82, 1739–1742 CAS.
- C. Powell, J. Shansky, M. Del Tatto, D. E. Forman, J. Hennessey, K. Sullivan, B. A. Zielinski and H. H. Vandenburgh, Hum. Gene Ther., 1999, 10, 565–577 CrossRef CAS PubMed.
- B.-X. Gao and L. Ziskind-Conhaim, J. Neurophysiol., 1998, 80, 3047–3061 CAS.
Footnote |
† Electronic supplementary information (ESI) available. See DOI: 10.1039/c3bm60166h |
|
This journal is © The Royal Society of Chemistry 2014 |