DOI:
10.1039/C3AY40517F
(Critical Review)
Anal. Methods, 2014,
6, 38-56
Nanoparticles: a global vision. Characterization, separation, and quantification methods. Potential environmental and health impact
Received
28th March 2013
, Accepted 29th August 2013
First published on 30th August 2013
Abstract
Nanotechnology is a multidisciplinary science that includes scientific and technological activities at molecular and atomic scales (1–100 nm). Scientific principles and new properties can be understood and controlled when working at this scale. Nanotechnology is advancing rapidly due to the great progress achieved in various fields including electronics, mechanics, medicine, cosmetics, food, etc. This increased use of nano-sized materials leads to the release of a substantial amount of nanoparticles into the environment. To date there is a lack of standardized procedures to assess their safety and impact on the environment. Specific toxicological studies as well as characterization and quantification of nanoparticles are required to establish regulations to control field application of nanoscale materials. A classification of nanoparticles and the techniques employed in their characterization, separation, and quantification are summarized and described in this review. A global perspective on nanoparticle exposure and environmental effect is also discussed.
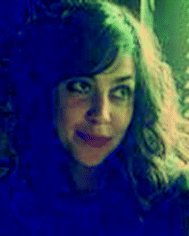 Ana López-Serrano | Ana López-Serrano Oliver, a predoctoral student in Analytical Chemistry doing her PhD on bioaccumulation of metals and nanoparticles by aquatic organisms. |
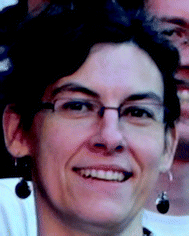 Riansares Muñoz Olivas | Riansares Muñoz Olivas has been a lecturer at the Department of Analytical Chemistry, Universidad Complutense de Madrid since 2008. Her main research topics are sample treatment methodologies as well as analytical method development for trace metal speciation. She has got more than 40 original articles on this topic. Recently, she has been focused on toxicokinetics and ecotoxicity studies in environmental aquatic media. |
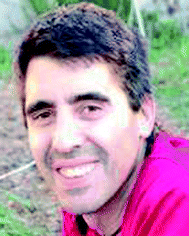 Jon Sanz Landaluze | Jon Sanz Landaluze obtained his doctorate in 2002, at the University of the Basque Country and then joined, as a post-doc, the Department of Analytical Chemistry at the Complutense University of Madrid. He obtained, in January 2013, a position as a lecturer. His main research areas deal with metal speciation, development of analytical methods for organic compound determination an (eco)toxicity studies of these metallic species in the environment. |
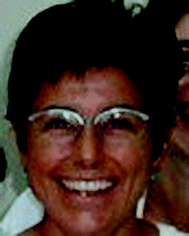 Carmen Cámara | Carmen Cámara has been a full professor in Analytical Chemistry since 1992 and leader of the research group of “Trace Metals, Speciation and Proteomics” at the Complutense University of Madrid. Her main research interest deals with trace metal speciation, functional food, nanoparticles and organic persistent contaminant determination in food and environmental samples. Recently, she has been focused on toxicokinetics studies by proteomic approaches. She has published more than 250 papers, 3 patents and several books. |
1. Introduction
Nanomaterials are defined as materials with at least one dimension between 1 and 100 nm. The dimensions of several entities are shown and compared in Fig. 1 to help explain the concept of nanosize. Nanoparticles (NPs) play an important role within the nanotechnology sector and are of great scientific interest.1 NPs have specific physico-chemical properties that differ from their bulk material. Their high surface
:
volume ratio causes an exponential increase in the molecule's reactivity. Therefore, the electronic, optical, and chemical properties as well as the mechanical characteristics can greatly differ. The high confinement of electrons causes an increase in the bandgap of semiconductors, optical absorption, and photoluminescence. Furthermore, mechanical properties such as elasticity, hardness, ductility also change. Some NPs can exert high catalytic activity and are used to accelerate oxidation–reduction reactions of certain pollutants. Due to their minute size, NPs can improve the antibacterial properties as close contact with bacterial membranes is possible.2
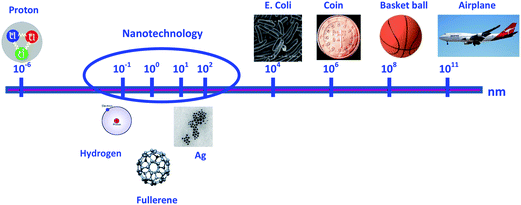 |
| Fig. 1 Comparative sizes of various entities to perceive nanoscale measurements. | |
Because of above-mentioned extraordinary properties, nanotechnology is one of the fastest growing technological sectors and with high economic impact due to its application in a wide range of areas including cosmetics, biomedicine, analysis, food and food packaging, bioremediation, paints, coatings, electronics, catalysis, and materials sciences.3 Some nanoscale materials have been used for decades (e.g., in window glass, sunglasses, car bumpers, and paints), whereas others are newly discovered (e.g., in sunscreens and cosmetics, textiles, coatings, sports goods, explosives, propellants, and pyrotechnics) or their applications are currently under development (e.g., in batteries, solar cells, fuel cells, light sources, electronic storage media, display technologies, bioanalysis and biodetectors, drug delivery systems, and medical implants). A spectacular increase in nanomaterial production has occurred in the last few years as illustrated in Fig. 2. It is worth noting that the main area where nanotechnology is being applied is in health and fitness, or in other words to benefit human well-being. However, not much is known about the adverse effects of these new products.
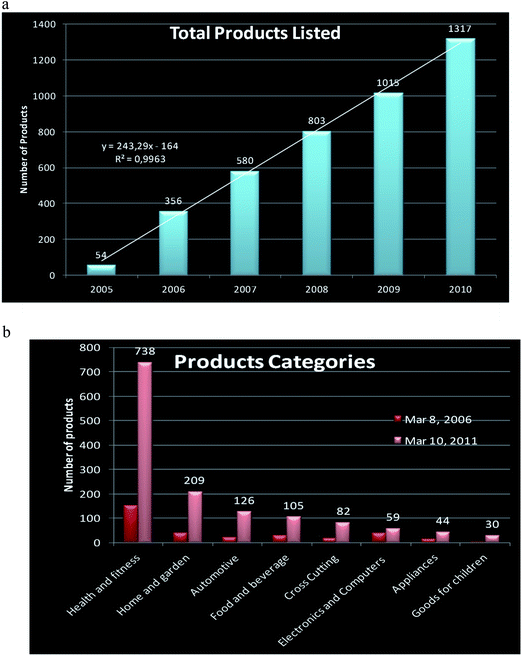 |
| Fig. 2 Production of nanomaterials over the last few years and the potential near future projections. 2a: total products listed; 2b: product categories. Extracted from http://www.nanotechproyect.org/inventories/consumer. | |
Exhaustive fate and behaviour research studies, as well as studies on their toxicological effects are advisable before increasing and promoting NP production and developing new applications. To date, little is known regarding their safety for humans. Some studies have revealed that the same properties that make NPs so unique, could also be responsible for potential harmful effects on the environment and human health.4,5 Present and future research is being focused on the interactions of the different types of NPs with aquatic organisms,6,7 plants,8,9 and humans.10,11 Very often, the problem of NPs is their unpredictable behaviour. In this context, NP determination in environmental and biological samples is a major concern. Development of accurate and robust methodologies for the characterization of particle size distribution, chemical composition, shape, etc. and quantification of NPs and their forms under real conditions are necessary. Sample treatment methods to ensure NP stability preventing their aggregation and degradation are also mandatory in order to assess their possible risks.12
An overview on NP classification, as well as their diverse applications and analytical characterization, separation, and detection methodologies will be presented in this review. Furthermore, and aiming to understand their impact on the environment and health, some NP-related effects on samples obtained from the environment will be discussed. The review also discusses the present knowledge and the future actions needed to prevent irreversible damage. Fig. 3 summarizes the issues addressed in this review.
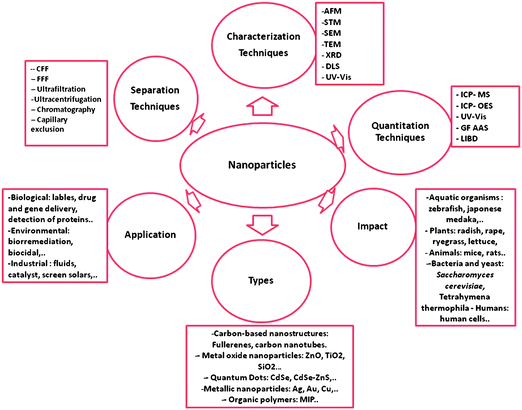 |
| Fig. 3 Categories for nanoparticles. | |
2. Nanoparticle classification and applications
A wide range of NPs has been described. They can be classified based on different parameters, their origin (natural or anthropogenic), chemical composition (organic and inorganic), formation (biogenic, geogenic, anthropogenic, and atmospheric), their size, shape and characteristics, their applications in research and industry, etc.
2.1. On the basis of their chemical composition
2.1.1. Carbon-based nanostructures.
Carbon-based nanostructures, made up of pure carbon, are classified into two main groups: (a) fullerenes: compounds containing at least 60 carbon atoms and b) carbon nanotubes (CNTs). Fullerene, C60, a ball made up of 60 carbon atoms is the simplest form known as “buckminsterfullerene”. C60 is a spherical molecule with the carbon atoms arranged at the vertices of a truncated icosahedron structure.13 There are other less stable forms such as C70, C76, C78, and C80 used in a multitude of biological and medical applications. It has been observed that fullerenes have antiviral activity, and are also capable of penetrating and establishing links in the catalytic sites of some enzymes. For instance, complex formation was demonstrated between HIV protease (essential for the survival of the virus) and fullerenes, which inhibited protease activity. Fullerenes lodge the active site residues of the viral protease with strong van der Waals interactions on the enzyme hollow surface.14
Carbon nanotubes (CNTs).
A large variety of CNTs with different properties are available, which are synthesized by different methods. These nanotubes have good electrical, mechanical, and chemical properties and they are very useful for electronics, polymer industry, energy sector, and medicine.15 CNTs have many applications such as: elimination of pathogens, natural organic matter, and cyanobacterial toxins from water systems due to their high adsorption capacities. Their fibrous shape and large external surface area can be easily accessed by biological contaminants.16
2.1.2. Metal oxide NPs.
This group includes a variety of transient metal oxides (Fe2O3, CuO, TiO2, ZnO, and CeO), and SiO2 combining the special properties of these elements with the high reactivity of NPs. They are being employed in an extensive variety of consumer products such as cosmetics and sunscreens, as catalysts, and in biomedicine.17–19 The engineered Al2O3 NPs have commercial applications in catalysis and in heat transfer fluids.20,21 As an example, the special superparamagnetic properties of iron oxide NPs have been widely used in numerous in vivo applications such as magnetic resonance imaging contrast enhancement, tissue repair, immunoassay, detoxification of biological fluids, drug delivery, etc.22
2.1.3. Quantum dots (QDs).
Quantum dots (QDs) are auto-fluorescent semiconductor nanocrystals that have been widely employed for in vivo biomedical imaging.23–26 Due to their quantum confinement, QDs show some unique and fascinating optical properties, such as sharp and symmetrical emission spectra, high quantum yield, special chemical properties and high photo-stability. The simplest chemical constituents of QDs are binary metal complexes, CdSe, CdS, CdZn, etc. being the most widely used for biological labelling in a variety of animal cells. Other QDs can be formed by combination such as CdSe–ZnS core–shell nanocrystals and are employed as bioactive fluorescent probes in sensing, imaging, immunoassays, and other diagnostic applications.
2.1.4. Elemental metallic NPs.
This group involves some inorganic NPs mainly composed of noble elements (Au, Ag), but also some transition metals (Fe, Zn) with many applications: transport, catalysis, optical sensors, bioremediation, solar panels, surgery, detection of biomolecules, etc. Silver NPs have unique antimicrobial activity due to the close contact between the Ag nucleus and the cell walls causing their disruption.27–30 Their applications have become silver nanoparticles in the largest and fastest growing class of nanomaterials. Nanoscale zero-valent iron remediation has been used for contaminated groundwater and soils because of the high adsorption capacity and reactivity.31 Gold NPs are normally employed in optical sensors.32,33 They have recently become an important goal as elemental tags in proteomics because gold NPs can be conjugated with biomolecules through their functional groups and serve as chemical anchors and elemental markers.34
2.1.5. Organic polymers.
There are organic polymeric NPs that can be highly stable in contact with biological fluids. Their polymeric nature permits the attainment of desired properties such as controlled and sustained drug release. It has been shown that biodegradable polymeric NPs with appropriate surface modifications can deliver drugs of interest beyond the blood–brain barrier for diagnostic and therapeutic applications in neurological disorders.35 Their use as modifiable and/or imprinted polymers has shown a high specificity for binding to the recognition site for given chemical compounds. Therefore, they are widely used in other areas like solid phase extraction, catalysis, medicine, clinical analysis, environmental monitoring, and sensors. For instance, molecularly imprinted polymer NPs synthesized for human rhinovirus immunoglobulins result in artificial antibodies with improved selectivity and sensitivity.36
2.2. On the basis of their application area
The classification and applications of NPs are summarized in Table 1.
Table 1 Classification and main applications of nanoparticles
Nanoparticle |
Chemical composition |
Applications |
Principle of application |
References |
Carbon nanotubes (CNTs) |
Pure carbon |
Environmental |
Adsorption of pollutants from contaminated sites |
17, 47
|
Increasing growing rate of plants |
16, 48
|
Energetic |
Alternative energy storage media |
16
|
Construction |
Increase the strengths of materials to build spacecrafts, space elevators, artificial muscles, and land and sea vehicles |
56
|
Medical |
Selective reactivity with certain biomolecules |
16
|
Fullerenes |
Pure carbon |
Medical |
Selective reactivity for antiviral activity |
14, 15
|
nAPU |
Polyurethane |
Environmental |
Remediation of soils contaminated with PAHs |
46
|
Metal oxides |
TiO2 and ZnO |
Cosmetics |
Filter UV radiation |
52
|
TiO2 |
Construction building |
Photocatalytic capacity of semiconductor materials |
54
|
Silica |
Constructing building |
Increase the strength of cementitious composites |
55
|
TiO2 |
Paint industry |
Whitening pigments |
18
|
TiO2 |
Environmental |
Positive effects on strength and growth of plants |
49
|
CeO |
Energetic |
Catalysts that increase fuel efficiency |
19
|
CuO and Al2O3 |
Energetic |
Improvement of thermal conductivity |
50
|
CuO |
Energetic |
Catalysts |
20
|
Iron oxides |
Biomedical |
Superparamagnetic properties |
21
|
Sensors for microbial detection |
45
|
Quantum dots (QDs) |
CdSe |
Medical diagnosis |
Luminescence properties for labelling bacteria |
36
|
CdS |
Biomedical imaging |
Biomarkers |
22–25
|
CdSe/ZnS |
Bioactive fluorescence; immunoassay applications |
36, 37
|
Metallic nanoparticles |
Ag |
Consumer products |
Antimicrobial properties |
26–29
|
Sensors |
Localized surface plasmon resonance |
26–29
|
Biomedical |
Antibacterial activity |
40
|
Au |
Sensors |
Surface plasmon resonance |
31, 43, 44
|
Au |
Proteomic studies |
Conjugation with biomolecules |
32, 33
|
Fe |
Environmental |
Detoxification of organic pollutants |
30
|
Polymers |
Alginate/chitosan |
Biomedical |
Slow drug delivery |
35
|
Agroindustrial |
Encapsulated, adsorbed or dispersed bioactive compounds maintaining their structure, activity and releasing over a longer time |
51
|
2.2.1. Biological applications.
This is one of the areas with the highest NP production and application. NPs may be used as (a) biological labels, because of their size (generally within the same size range as proteins) and fluorescent properties, particularly quantum dots. NPs form the core of nano-biomaterials and interact with the biological target through non-covalent interactions. The approaches used to construct these nano-biomaterials are based on their biocompatibility, antigen detection, shape recognition, and fluorescent signalling. They can also be used for bacterial detection.25 Also, CdS NP tracers can be conjugated with specific bacteria; this combination allows the detection of DNA hybridization for immunological assays;37 (b) drug and gene delivery; the inclusion of drugs or genes into NPs protects them from degradation and improves cellular uptake.38,39 Silver NPs entrapped in montmorillonite were administered to mice, proving that they can offer novel advantages over current agents used as drug carriers;40 (c) protein ultrasensitive electrochemical detection, by employing nano-sized biosensors with high versatility and inherent electrochemical advantages.41 Quantum dots have been used for rapid and sensitive detection of prostate-specific antigen (PSA) in human serum,42 achieving detection limits of 20 pg mL−1; (d) determining DNA structure, by forming DNA conjugates and exploiting their unique optical and electronic properties. This way, gold NPs are able to distinguish between target-free and target-bound oligonucleotides via surface plasmon resonance43 or can detect target-response structural variations of DNA;44 (e) microbial monitoring and detection, offering an attractive alternative for in vitro and in vivo identification of molecular targets. Iron oxide nanosensors have been shown to be very sensitive for the quantification of biomolecular targets in cell lysates and tissue extracts.45
2.2.2. Environmental applications.
This is one of the most attractive and promising applications within the nanotechnology sector. (a) Bioremediation, since NPs have the particular property of having a high adsorption capacity. For example, amphiphilic polyurethane (APU) NPs have been used to remediate soils contaminated with PAHs. These NPs have been proved to have high affinity for phenanthrene and hydrophilic surfaces promoting particle mobility in soils.46 Furthermore, carbon nanotubes serve as nanosorbents with exceptional adsorption properties and used for removing heavy metals, organic pollutants, and biological impurities;47 (b) acceleration of growth in some plants; it has been demonstrated that carbon nanotubes can significantly accelerate germination and growth of tomato seeds.48 Nanostructures were found to penetrate tomato seeds and affect their germination and growth rates. The germination was found to be drastically increased in the presence of CNTs. Another interesting study reported the positive effects of nano-TiO2 (rutile) on spinach seed strength and growth, suggesting their use in agricultural applications.49 The strength came from the fact that nano-TiO2 facilitates chlorophyll formation, and enhances rubisco activity and the photosynthetic rate during the growth stages of spinach. (c) Agroindustrial applications: NPs are being used to encapsulate, adsorb or disperse bioactive compounds through the particle matrix. NP-based release systems are able to maintain the structures and activities of the associated substances, and release the substances over a longer period. For example alginate/chitosan NPs are used to encapsulate paraquat, a non-selective contact herbicide with important secondary effects on the photosynthesis of plants. Alginate/chitosan NPs have been prepared as carrier systems for the herbicide paraquat resulting in more effective reduction of its negative impacts. The formulation of herbicides with NPs offers an increased effect of the chemical on specific targets, while reducing problems of environmental toxicity.50
2.2.3. Industrial applications.
NPs have been used in different industrial branches over the last two decades. (a) Heat transfer: NPs exhibit high thermal conductivity that can be used in heat transfer. Thus, industrial heat transfer uses NPs in fluids such as water, ethylene glycol, or engine oil to produce a new class of engineered fluids;51 (b) food industry and nutrients, e.g., lipid-, protein- or polysaccharide based particles that are able to disperse hydrophobic β-carotenes in beverages, to entrap enzymes for cheese production, and to fortify dairy products with vitamins increasing their nutritional quality and aiding their digestion process;52 (c) general personal care consumer products such as sunscreens, cosmetics including deodorants, soaps, toothpastes, shampoos, hair conditioners, anti-wrinkle creams, moisturizers, face powders, lipsticks, blush, eye shadows, nail polishes, perfumes, and after-shave lotions in which NPs are already being used.4 (d) Construction and building materials: the most relevant application of nanomaterials in the construction industry relates to the photocatalytic capacity of semiconductor materials. Titanium dioxide (TiO2) NPs are applied onto surfaces and through their volume incorporation in the conventional asphalt mixtures with tailored photocatalytic properties. The TiO2 NPs reduce building facade maintenance costs and also the pollution effects, particularly important in metropolis with high population density, by their photocatalytic and self-cleaning effects.53 Moreover, NPs are being used to increase the strength and durability of cementation composites. Silica NPs increase the compression strength of cement pastes since they contribute to denser microstructures.54 Materials containing carbon nanotubes may be also strong enough to build spacecrafts, space elevators, artificial muscles, and land and sea vehicles.55
3. Nanoparticle analysis
Chemical composition, structure, size, and shape play a major role in the particular and unique properties that characterize NPs and their environmental and health impact. Several studies have tried to establish which characteristics of the NPs are required for hazard identification. The authors have emphasised the need to carry out NP characterization in a reliable and robust way.56–58 So far, there are no validated analytical methods for this purpose. In order to elucidate the mechanisms of toxicity of NPs, to underpin the processes of their environmental fate and behaviour, and to be able to extrapolate results between NPs, it is essential to characterise the materials used in the different studies as far as possible. Many of the analytical techniques that have been used in NP characterization are reviewed here.
3.1. Size determination
3.1.1. Microscopic techniques.
Microscopic techniques are the most employed characterization tools as they create surface images using a physical probe that scans the specimen. Some of the used microscopic techniques are: (i) Atomic Force Microscopy (AFM), which provides qualitative and quantitative information on many physical properties including size, morphology, surface texture, and roughness. This technique overestimates the dimensions of the NPs when the geometry of the tip is larger than the NPs themselves;59 (ii) Scanning Tunneling Microscopy (STM) allows the chemical identification of the atoms and molecules that make up the NPs. STM can operate in a ultra-high vacuum allowing characterization in liquid and gaseous suspensions;60 (iii) Scanning and Transmission Electron Microscopy (SEM and TEM) provide information about the sample's surface, crystal structure, elemental composition, size, shape, and other properties such as electrical conductivity.61 However, most of these imaging techniques can lead to imaging artefacts, due to previous treatment of the sample (coating, drying, staining, freezing, and embedding) before measuring and also due to the vacuum conditions in the sample chamber;62 (iv) Environmental scanning electron microscopy (ESEM) is an approximation; currently the imaging of non-modified samples is still not possible;63 (v) WetSEM technology, in which stainless steel capsules are used and equipped with an electron-transparent membrane so that wet samples can be placed into the capsules and imaged in a standard SEM. However, there are still drawbacks to this technique.59
3.1.2. Light scattering techniques.
Light scattering techniques, such as Dynamic Light Scattering (DLS), are commonly used to determine particle size. Furthermore it is a nonintrusive, sensitive, and powerful analytical tool that is commonly employed for the characterization of macromolecules and colloids in solution.64,65
3.1.3. X-ray-based methods.
X-ray-based methods such as X-ray absorption (XAS), X-ray fluorescence (XRF), X-ray photoelectron spectroscopy (XPS), and X-ray diffraction (XRD) are in general highly surface-specific and can provide information on surface properties and coatings, crystallographic structure or elemental composition. X-ray spectroscopy is often combined with SEM and TEM for the assessment of the elemental composition and quantitative analysis.66,67
3.1.4. Spectroscopic techniques.
Spectroscopic techniques, such as UV-visible, are commonly used in NPs with surface plasmon resonance by collective oscillations of their conduction band electrons in response to electromagnetic waves. This allows obtaining information on NP size, aggregation, structure, stabilization, and surface chemistry.68 Metal NPs display specific absorbance bands in their spectra when the incident light enters into resonance with the conduction band electrons on their surface. For example, Ag-NPs exhibit a characteristic absorbance band, in the UV region, due to the excitation mode of their surface plasmons, which is dependent on NP size.69
3.1.5. Nanoparticle tracking analysis (NTA).
Nanoparticle tracking analysis (NTA) is an innovative system for sizing particles from about 30 to 1000 nm, with the lower detection limit being dependent on the refractive index of the NPs. This technique allows direct visualisation of a liquid NP suspension. The technology comprises a metalized optical element illuminated by a laser beam at the surface and a conventional optical microscope fitted with a low cost camera and a dedicated analytical software package. It is employed to control release or the precise delivery to a specific target of drugs encapsulated with NPs.70
3.1.6. Hyperspectral imaging.
Hyperspectral imaging works by scattering the obliquely incident visible and near-infrared light in an enhanced darkfield background. The sample information is shown in terms of the spatial distribution as well as spectral characteristics unique to each nanoparticle type, at a sensitivity of a single nanoparticle (size <10 nm). Hyperspectral imaging with enhanced darkfield microscopy is employed for detection and characterization of engineered NPs in environmental systems, facilitating studies on fate and transformation of these particles in water samples. This includes the ability to map the presence and location of nanomaterials in a wide range of environments.71 In addition, hyperspectral imaging is used to characterize unique surface chemistry and functional groups added to nanomaterials. One of their most common uses is imaging of NPs inside cells.72
3.2. Extraction, separation, and fractionation
Instability of NPs is well known. They can be very reactive depending on the composition of the surrounding media or physico-chemical parameters. NPs can aggregate, change their initial chemical form, etc. Furthermore, these properties can change during sample manipulation, storage, residence within the biological system, among other reasons, and these changes may greatly influence nanoparticle uptake and/or behaviour. Several techniques are used for a suitable extraction, separation, and fractionation of NPs during pre-quantification. Some of the most employed techniques are briefly explained below.
3.2.1. Cloud Point Extraction (CPE).
Cloud point extraction (CPE) used for extracting NPs is a critical step in their analytical processing. There are several methods for this purpose, although many of them still need to be tuned up. Besides the matrix effects in environmental samples, the low concentration of NPs usually requires an enrichment step prior to their analytical determination. One approach for NP quantification is CPE, which consists in adding a surfactant to the sample at a concentration that exceeds the critical micelle concentration. At a temperature higher than that for a specific cloud point, the surfactant forms micelles in which non-polar substances are encapsulated. Since the density of the micelles is higher than that of water, they settle after some time, a process that is usually accelerated by centrifugation. Up to now, this methodology has been applied for determining silver NPs in water, allowing their separation from ionic silver.73,74
3.2.2. Cross-flow filtration (CFF).
NPs are fractionated based on their particle size according to their diffusion coefficients through a very thin open channel. Recirculation of the sample prevents clogging, concentration polarization, and other artefacts caused by traditional dead-end filtration.75 As an illustrative example, cross-flow filtration of a nano-sized nickel catalyst suspension was used in combination with ceramic membranes achieving 100% separation of the NPs and no adverse effects were observed during the catalysis.
3.2.3. Field-flow fractionation (FFF).
Field-flow fractionation (FFF) is an emergent analytical technique for size separation of natural and inorganic NPs. The two main principles are sedimentation (SedFFF) and asymmetric flow field-flow fractionation (AF4). Separation of high-molecular mass compounds, from macromolecules (low nm range) to NPs and μm-size particles, is achieved by the interaction of sample components with an external generated field that is applied perpendicularly to the direction of the mobile phase flow. It is fast and non-destructive although some NP aggregation within the channel may occur. In addition, it can be coupled with a second flow stream resulting in flow field-flow fractionation (F4), where a greater separation is accomplished.76,77 Elution time under identical processing conditions is solely related to particle size and follows a linear correlation. The combination of AF4 techniques with UV and ICP-MS detectors allows the determination of particle-size distribution and quantification of metallic NPs.78 Due to their nature, NPs can change under environmental conditions and sample treatment; sampling and sample preparation have to be optimised to prevent these changes. Stabilization of NPs and ionization efficiency in the ICP can be improved by adding surfactants or methanol to the carrier solution.79 AF4 requires optimization of a relatively large set of parameters such as cross-flow rate, injection/focusing time, equilibration-cleaning of the membrane surface, sample pH, etc., to achieve an efficient particle size separation. A further advantage of F4 is the possibility to couple the separation channel with several in-line detectors. Some recently published studies investigated the possibilities of AF4 with a multidetector approach coupled with UV-Vis, light scattering, and ICP-MS, for size fractionation chemical analysis of gold and silver NPs.80 Although very versatile, F4 is hampered by the need to optimize a relatively large set of parameters to provide ideal running conditions for all particles present in the sample. This fractionation technique was tested as a representative example with a mixture of polystyrene colloids in three different nominal sizes, 19, 50, and 102 nm, obtaining quantitative data for trace particle concentration by Laser-Induced Breakdown Spectroscopy (LIBS).81
3.2.4. Ultrafiltration (UF).
Using membranes with pore sizes in the nm range allows separation of NPs of different sizes without much perturbation of the sample. This technique is fast and requires little sample preparation.60 Some studies carried out with silver NPs proved that the ultrafiltration method was more efficient and effective, providing greater size control, concentration, and aggregation state in comparison with conventional isolation methods such as ultracentrifugation.82 The disadvantages of using ultrafiltration are the possible interactions with the ultrafiltration membrane as well as poor size resolution. A particular ultrafiltration procedure is tangential flow ultrafiltration that uses a series of membrane models with pores ranging from 1 nm to 100 μm in a single-step procedure.83
3.2.5. Ultracentrifugation (UC).
Ultracentrifugation (UC) is a very versatile and powerful tool for NP characterization and fractionation. It may be applied to a very wide range of molecular masses (25 kDa to 1.5 MDa). The sample is subjected to a vacuum at a controlled speed and temperature while its concentration distribution is recorded at set time intervals. This technique allows analysis of the different fractions and obtaining information about their size and behaviour in a specific medium. Sample perturbation is low but, similar to UF, low-size resolution is achieved.84,85
3.2.6. Chromatographic techniques.
Size exclusion chromatography (SEC).
Size exclusion chromatography (SEC) has been historically used to separate different compounds on the basis of size and shape, but in the case of NPs some inherent problems such as degradation or losses by irreversible adsorption can be very important. Addition of surfactants in the mobile phase may reduce the mentioned adsorption problems but this new agent can result in a lack of separation resolution.86
Hydrodynamic chromatography (HDC).
The separation takes place in the inter-particle channels of narrow open packed capillaries, or in wider capillaries with non-porous packing materials, that create capillary routes. Larger components elute sooner than smaller ones. Sample components are separated in the cartridge according to the different eluent velocities experienced by the different particles, as a consequence of the velocity gradient created in the inter-particle spaces of the packed bed of the effective capillaries.87
Reversed-phase high-performance liquid chromatography (RP-HPLC).
A method using this technique has been developed for the speciation of engineered Ag-containing NPs, allowing the complete simultaneous and precise determination of Ag bound NPs and ionic silver species.88
3.2.7. Capillary electrophoresis (CE).
Capillary electrophoresis (CE) measures the electrophoretic mobility of NPs based on their charge and size distribution in the sample, when an external electric field is applied. Ions move towards the electrode of opposite charge. The separation would be achieved by the mobility of the species depending not only on the solvent medium, but also on the charges, sizes, and shapes of the NPs. The ability of capillary electrophoresis as a separating tool for mixed NPs according to their sizes as well as the nature of materials has been demonstrated. However, as the amount of the sample used is rather low a high concentration of NPs is needed.89
3.3. Quantification
NP quantification is the third but decisive step to understand the wide range of processes in which they may be involved. The main bottleneck for NP quantification is the lack of validated analytical methods and standards. Currently, research focuses on the characterization of NPs, while information on quantification is very scarce. Furthermore, the size of NPs is far from being uniform and generally coupling of separation with quantification analytical techniques is required to obtain a fraction within a desirable size range, and also to distinguish between NPs and other species that can reach the detector. The most frequently used analytical techniques are summarized below and are all based on elemental determination coupled with one of the previously described fractionation techniques.
3.3.1. Inductively coupled plasma mass spectrometry (ICP-MS).
Inductively coupled plasma mass spectrometry (ICP-MS) is a highly sensitive analytical technique widely used for ultra-trace metal determination in a wide range of samples. It is being used for the determination of NPs and their corresponding ionic forms in biological samples exposed with the aim to evaluate the different toxicity of both compounds. However, the quantification of NPs by ICP-MS is usually related to solution chemistry since NPs have to be digested into a soluble speciated state. ICP-MS cannot differentiate NPs or dissolved forms unless a physical separation is previously done. Only in a few cases, and very dependent on NP size and shape, the nebulization rate of ICP-MS can avoid the need to dissolve the nanoparticles. Some authors90 have used ICP-MS to determine quantum dots in mice, as a primary quantification method, by monitoring Cd isotopes. Single-walled carbon nanotubes (SWCNTs) can also be quantified by ICP-MS using CNT-associated nickel as the probe.91 Other NPs quantified by ICP-MS are gold92 and nickel NPs.93 Several improvements have been proposed over the last few years for enhancing NP analysis using ICP-MS. One clear example is the introduction of single particle (sp)-ICP-MS for size distribution determination of NPs in colloidal suspensions.94 In single particle analysis, the analyte is spatially concentrated, in comparison to a solution of a soluble form of the same analyte. When one particle is introduced into the ICP, the atoms of the analyte produce a flash of gaseous ions in the plasma, which are measured as a single pulse by the detector. Meanwhile, the technique has been improved, e.g., by using micro-droplet sample introduction devices,95 to achieve better detection limits. The principle of sp-ICP-MS is based on transient signal spike counting statistics of highly diluted samples, which requires a fast data acquisition. The major advantage of sp-ICP-MS is the relatively low instrumental effort, which makes the technique easily accessible in every ICP-MS laboratory. However, sp-ICP-MS analysis is limited by the time resolution of the mass spectrometer used.96 Another recent analytical strategy is the detection by ICP-MS using isotopically modified NPs. This procedure allows low detection limits and an adequate monitoring of their fate and transformation in the sample of interest.97 In addition, recently new instrumentation has been developed for the analysis of gold NPs, using a customized electrospray-differential mobility analyzer (ES-DMA) to achieve real-time upstream size discrimination before ICP-MS detection.98 DMA, also known as scanning ion mobility spectrometry, is a high-resolution size classification technique relevant to the analysis of discrete (dispersed) nano-scale species such as NPs, viruses, proteins, and DNA.
3.3.2. Inductively coupled plasma optical emission spectroscopy (ICP-OES).
Inductively coupled plasma optical emission spectroscopy (ICP-OES) is also a powerful analytical technique that is being employed in NP analysis.99,100 Concentrations at the μg L−1 are perfectly detected with this technique. An analytical method to simultaneously detect three varieties of NPs using an ICP-OES (CdSe/ZnS, Au, and Fe3O4) has been developed.101 ICP-OES has been widely used as a tool for assessing in vitro nanotoxicity. Uptake of transferrin-coated gold NPs of different shapes and sizes in mammalian cells has been studied. Finally, a mathematical equation to predict the relationship of AuNP exocytosis and size was developed. These models would have implications in nanotoxicity studies.102
3.3.3. Liquid chromatography-mass spectrometry (LC-MS).
The separation of nanomaterials from the sample is based on the rates at which they elute from a stationary phase typically over a mobile phase gradient. Differing affinities of the mixture's components for the stationary and mobile phases lead to their separation. This technique has drastically improved in sensitivity, specificity and reliability. It is one of the most common techniques employed for the analysis of fullerenes in environmental matrices. First, they have to be extracted from the sample to facilitate their determination by LC/MS since the water solubility of unmodified C60 is low. The extraction of fullerenes has been achieved by exploiting their solubility in toluene. nC60 concentrations as low as 300 ng L−1 in water were quantified using the solid-phase extraction (SPE) separation method.103 High-performance liquid chromatography (HPLC) coupled with mass spectrometry at a negatively charged m/z of 720 is also used to quantify C60–toluene extracts providing specificity to differentiate fullerenes based on the m/z of charged molecules.104 Recently, the quantification of fullerenes is carried out with approximation of this analytical methodology using more sensitive approaches such as: liquid chromatography-tandem mass spectrometry (LC-MS/MS)105 and liquid chromatography-electrospray ionisation coupled to mass spectrometry (LC-ESI-MS) in the negative ion mode.106
3.3.4. Laser-induced breakdown spectroscopy (LIBS).
Laser-induced breakdown spectroscopy (LIBS) uses the light emitted from laser-generated microplasma, which is analyzed to determine the elemental composition of a material.107 When a solid nanoparticle passes through the focal volume of a pulsed laser, the power density required to induce breakdown of the dielectric properties of the solution is lower than that for pure water. If the laser energy is correctly tuned, plasma formation will only occur when a nanoparticle passes through the focal volume of the optical cell. LIBS provides the possibility of multi-element microanalysis of bulk and residue samples at the parts-per million-range with little or no sample preparation. LIBS is a non-destructive technique and is extremely sensitive to small NPs with detection limits within the ng dm−3 range.108 LIBS is so sensitive that most samples have to be previously diluted. However, LIBS cannot discriminate between different sizes of NPs and even, different nanoparticle compositions have different breakdown probabilities (instrument responses). Therefore it is not possible to use one set of calibration standards for different types of NPs This drawback implies the need for using quite homogeneous dispersions. A good example of this technique for NP quantification is the work published by Thang et al. to determine colloidal NPs of different sizes, 19, 50, and 102 nm after FFFF.109
3.3.5. Graphite furnace atomic absorption spectroscopy (GF-AAS).
Graphite furnace atomic absorption spectroscopy (GF-AAS) allows direct injection of NPs into the graphite tube with minimum manipulation of the sample. Silver ion release r from NPs by GF-AAS and UV-Vis spectroscopy was evaluated.110 The UV-Vis spectra obtained with 300–700 nm allowed detection of NPs modified in size, morphology, and stability over time and GF-AAS was employed to develop kinetic models on the behaviour of AgNPs in natural waters. New advances in this technique point towards the use of high-resolution continuum source GF-AAS. It offers enhanced features for the detection and correction of spectral interferences, as well as for expanding the linear range. This last characteristic becomes significant when direct solid sampling is attempted because it is not feasible to dilute the sample if the analyte content exceeds the upper limit of the linear range.111 Moreover, carbon-based nanomaterials in biological samples are commonly determined with this technique by measuring the low content of the cobalt catalyst that remains bound to CNT after purification.112,113
Characterization, fractionation, and quantification techniques of the most used NPs are summarized in Table 2. After reviewing the techniques mostly used for NP analysis, we believe that several techniques must be simultaneously used in order to get a complete picture of these complex analytes.
Table 2 Nanoparticle characterization, separation, and quantification techniques
|
Characterization technique |
Information provided |
Microscopic techniques |
AFM |
Size, morphology, surface texture, electrical and mechanical properties |
STM |
Elemental and molecular composition |
SEM |
Surface, size, shape morphology, crystallographic composition, elemental composition, electrical conductivity |
TEM |
X-ray based techniques |
XAS, XRF, XPS, XRD |
Surface, crystallographic, and elemental composition |
Light scattering |
DLS |
Particle size |
Spectroscopy techniques |
UV-visible |
Size, aggregation, structure, surface chemistry |
|
|
Fractionation technique
|
Principle of separation
|
Filtration techniques |
CFF |
Diffusion coefficients through an open channel |
FFF |
Interaction with an external and perpendicular field |
UF |
Diffusion through a membrane |
|
UC |
Deposition of particles at controlled speed and under vacuum |
|
CE |
Electrophoretic mobility under an external electrical field |
Chromatographic techniques |
SEC |
Interaction with the stationary phase |
HDC |
Routes formed by open capillaries packed with non-porous materials |
|
|
Quantification technique
|
Analytical performances
|
Plasma techniques |
ICP-MS |
Low detection limits; isotopic analysis; multi-element analysis |
ICP-OES |
Simultaneous NP analysis; relative low detection limits |
|
LIBS |
Multi-element microanalysis; little or no sample preparation |
|
GF-AAS |
Direct NP injection; little or no sample preparation |
4. Difficulties associated with the instability of nanoparticles
NPs differ from conventional dissolved chemicals in their heterogeneous distribution in size, shape, surface charge, composition, degree of dispersion, persistence, mobility, bioavailability, etc. Before analyzing the potential effects of NPs, their chemical behaviour, e.g., their ability to oxidize, reduce, and dissolve in biological media, leading to the release of toxic ions, must be evaluated. Because the toxicity of NPs is not well understood yet and their initial chemical form can be affected by different external factors, it is essential to consider their stability, or more precisely, their lack of stability.114,115 In order to predict the potential risk of exposure to NPs, a complete understanding of several additional aspects such as their release into air, water and soil as well as their persistence and mobility among compartments is necessary.116
A key parameter to assess would be the coexistence of NPs and their ionic forms, most probably with different fate and transport characteristics, and independent or synergistic toxicity pathways. Parameters such as the release rate of ionic forms, their aggregation, solubility, and stabilization in different media are frequently evaluated.117 Similarly, sample preparation may be critical and should ideally be optimized to find the right balance between reducing the complexity of sample handling and maintaining its representativeness. NPs are usually stabilized by adding capping agents or adjusting certain parameters such as pH, temperature, and UV light.118 Some relevant examples are described below.
Liu et al. investigated the effects of dissolved oxygen, pH, temperature, salt content, and natural organic matter on ion release kinetics and particle persistence in aqueous nanosilver colloids.119 The study led to an interesting finding: a simple nAg colloid consisted of three silver forms, Ag0 NPs, free Ag+ (including any soluble complexes), and surface-adsorbed Ag+. It was also predicted that AgNPs would not persist in environmental compartments containing significant dissolved oxygen. On the contrary, it would be oxidized to ionic silver. However, this redox process occurs slowly; therefore, NPs may persist long enough to open new pathways for silver partitioning and transport. Furthermore, the presence of natural ligands and ionic strength in the environment plays an important role in NP toxicity because they can interact with ligands forming different types of complexes. The presence of ligands such as sulphide in wastewater treatment may affect NP toxicity to nitrifying organisms, influencing the oxygen uptake rate measurements.120 Addition of some ligands such as S−2, Cl−, PO4−3, and EDTA reduced nanosilver toxicity, due to a decrease of their bioavailability, more effectively than that caused by Ag+.
The effect of adding stabilizing agents to avoid the release of both ions and NP agglomeration has been evaluated by different authors. A study on AgNP stability and persistence in natural freshwaters and synthetic aquatic media of citrate-capped AgNPs showed that a significant fraction of the initially singly dispersed AgNPs was agglomerated and decanted in the sediments, while a small fraction remained in solution.121 This finding showed the potential risk of AgNPs due to the high transport rate into estuarine waters and high availability to aquatic organisms. Surfactants, as stabilizing agents, mainly prevent the agglomeration122 of various types of metallic NPs, which remain stable for more than three months when NPs are synthesized in the presence of apiin (apigenin-7-apiosyl-glucoside, a green biologically compatible agent). Poly(vinylpyrrolidone) (PVP) has been reported to be a good stabilizing agent for selenium NPs in aqueous solution,123 also leading to highly regular morphologies and small diameters in comparison to those synthesized without PVP.
TiO2 NPs tend to aggregate and settle in an aqueous medium.124 Studies on the effects of environmental conditions such pH, ionic strength, organic matter, etc., are a predominant research topic with these highly unstable NPs in order to clarify their mobility and stability in complex aqueous matrixes. The use of different coating agents is being applied for NPs that tend to agglomerate easily. Humic and fulvic acids,125 polyethylene glycol (PEG),126 mono- and binary-ion systems naturally present in natural waters111,127 are among the tested stabilizing agents. However, in many cases, the results do not fit the expectations.
5. Exposure to nanoparticles: health and environmental impact
5.1. Exposure to nanoparticles
The increasing production and uses of NPs have raised concern about the release of engineered nanomaterials to the air, water, and soils due to their potential toxicity impact. To be able to minimize their negative effects, research on this matter is urgently needed, e.g., assessment of NP fate, behaviour, and biological effects.128
There are two main types of exposure: (i) external exposure, bioavailability of NPs in the surrounding environment of an organism; and (ii) internal exposure, NPs that have been taken by the organism and can be metabolized, and transferred to different organs and tissues. In both cases, it is important to characterize the concentration of the expected NPs that may be present in the air, water, and soil, and how the human population can be directly or indirectly exposed by consuming food that has accumulated NPs.129 To assess the external exposure risk, accurate measurements of environmental concentrations, speciation, and mobility are required; studies on internal exposure need to quantify uptake, metabolism and NP excretion.130
When NPs are present in the air, the primary route of human exposure is by inhalation. The common sources of anthropogenic NP inhalation are probably motor vehicle emissions in an urban environment or fossil fuel combustion. NP exposure, uptake, distribution, and degradation from the environment in different sectors have been recently discussed.13 Occupational exposure must be also considered, as well as some industrial activities in which exposure to NPs may occur. The following fields can be representative examples: nanotechnology sector, chemical and pharmaceutical companies, powder-handling processes including paint, pigment, and cement manufacture, and other processes where NPs are by-products. Furthermore, cutaneous exposure is an important entry route because nanomaterials have been used in cosmetics and pharmaceutical compounds for many years. To date, knowledge on NP impact is very poor. Currently, most research is focused on NP skin penetration using different formulations of cosmetics. Finally yet importantly, ingestion exposure should also been considered. NPs can translocate from the lumen of the intestinal tract via aggregation of intestinal lymphatic tissue. Most of the studies focused on ingestion exposure concluded that the smaller the NPs the greater the danger due to their high permeation.131
5.2. Nanoparticle-related health and environmental impact
Once the NPs are released into the environment, assessment of their potential harm in the different organisms must be considered. The impact of NPs will be assessed by evaluating their toxicity and bioaccumulation in living organisms. There are several parameters that provide risk assessment for any toxic compound: LC50 (lethal concentration of a contaminant that will kill half of the sample population), EC50 (effective concentration of the toxin inducing a response between the baseline and maximum after some specified exposure time), NOEC (not-observed-effect-concentrations), BCF (bioconcentration factor defined as the ratio between the toxicant concentration in the biological tissue and the surrounding medium). Some examples of recent studies performed with NPs are summarized in Table 3 and discussed below.
Table 3
In vivo toxicity studies using different types of nanoparticles
Organism tested |
Type of NPs |
Conclusion |
Reference |
Nitrifying bacteria |
nAg |
Sulphide ligands reduce their toxicity |
107
|
Luminous bacteria |
nAu |
Toxicity decreases with increasing nanoparticle size |
132
|
CNTs |
Tetrahymena thermophila (protozoan) |
nZnO |
Toxic effects are caused by their solubilization fractions |
133
|
Sacharomyces cerevisiae
|
nCuO |
134
|
Drosophila melanogaster
|
nAg |
Impact on glutathione levels |
135
|
nTiO2 |
Decreased superoxide dismutase levels |
Lumbriculus variegatus
|
Fullerenes |
Decreased feeding rate and depuration |
136
|
Pseudokirchneriella subcapitata (microalgae) |
nZnO |
CuO > ZnO > TiO2 (toxicity)Toxicity is directly related to nanoparticle solubility |
8
|
nTiO2 |
nCuO |
Red algae |
nCu |
Bioaccumulation increases with nanoparticle concentration |
138
|
Radish, rape, ryegrass, lettuce, corn, cucumber |
nAl, nAl2O3, nZn, nZnO, MWCNT |
Growth inhibition with ZnO on ryegrass and corn |
137
|
Allium cepa
|
nZnO |
Elongation decrease of A. cepa roots by both the cobalt and the zinc oxide NPs |
139
|
nCoO |
Hydra vulgaris
|
MWCNTs |
MWCNTs could cause chromosomal aberrations, DNA fragmentation and apoptosis in Allium root cells |
140
|
Inorganic and organic |
EC-50 ranged from 0.1 to 1 mg to inorganic NPs and 1–10 mg L−1 for carbon nanotubes in hydra organisms |
142
|
Zebrafish embryos |
QDs |
QDs caused changes of cell morphology, leading to cell and animal death |
143
|
nAg |
Several toxicity parameters (mortality, hatching delay…) highly dependent on NP concentration |
66, 144
|
Medaka fish |
Coated nAg |
Dysmorphology and embryo viability dependent on nanoparticle shape and size |
86, 145
|
nNi |
Dysmorphology and embryo viability dependent on nanoparticle shape and size |
86,145,146
|
TiO2 |
Reduction in embryo viability |
nAg/Ag+ |
Cellular DNA damage, carcinogenic agents |
93
|
Fathead minnows |
nAg/Ag+ |
Larvae abnormalities induced by both species. Higher mortality with Ag+ |
147, 148
|
Daphnia magna
|
nAg, nCu, nNi, nTiO2 |
Toxic effects except for TiO2. Lower LC50 for nAg |
117
|
Arenicola marina
|
nTiO2 |
Genotoxic due to generation of radical species |
150
|
CNTs |
Not accumulated or excreted |
Mice |
nAu |
Body weight, hematocrit decrease with nAu concentration |
35,151,152
|
Accumulation in brain under intraperitoneal administration |
Elevated liver levels under intravenous administration |
|
Superparamagnetic nFe2O3 |
Nanoparticles entered and were accumulated in the breast cancer cells as a function of particle size and exposure time |
153
|
CNTs |
Production of ROS species and DNA damage |
154
|
Polymeric |
Autophagy, apoptosis, oxidative stress |
155, 156
|
Al2O3 |
Pathological alteration and inflammatory diseases |
157
|
Humans |
nAg |
Increase of ROS species with depletion of ATP |
158,159
|
Oxidative stress by depletion of GSH |
Buckyballs |
Death cell, (HDF) and (HepG2) cells, exposed to nanoC60, caused by the generation of oxygen radicals. |
160
|
QD's |
Skin permeability depending on QD physicochemical properties |
162
|
CdTe |
Damage to the mitochondria membrane and nucleus mediated by ROS |
163
|
CeO |
Reduces cancer cell viability; alternatives for cancer chemotherapy |
164
|
5.2.1. Bacteria and yeast.
They are commonly employed as indicators to assess the toxicity of several emergent chemicals. Choi et al. used the nitrifying bacteria present in wastewater to evaluate AgNP toxicity by measuring the oxygen uptake rate.120 They noted that some ligands, e.g., sulphide, effectively reduce nanosilver toxicity as a consequence of a decrease of their bioavailability by forming a chemical silver complex. Zheng et al. showed that toxicity of AuNP and carbon nanotubes in luminous bacteria was due to their ability to enter and aggregate into cells, the toxicity decreasing with the size of NPs.132 Speciation studies on the protozoan Tetrahymena thermophila exposed to ZnO and CuO NPs and their respective soluble oxides revealed in all cases a similar or moderately higher toxicity of NPs than that for oxide forms. The toxic effect of both NPs was attributed to their solubilization fraction (the toxicity of ZnO NPs being significantly higher than that of CuO NPs).133 Similar results were obtained in another study with the unicellular eukaryotic yeast Saccharomyces cerevisiae.134
5.2.2. Invertebrates.
There are a few toxicity studies within this category of organisms, but they are interesting because they may act as a pollution source to higher organisms. Drosophila melanogaster is the currently investigated model in relation to human health, particularly because it is specifically relevant to model oxidative stress response.3 Different effects were found in a study performed by Posgai et al.135 Reproductive effort and viability were influenced by NP size, coatings, and antioxidant matter; nanosilver biochemical toxicity was higher than that of titanium dioxide NPs; both NPs had comparable effects on superoxide dismutase levels, while nanosilver had a much greater impact on glutathione, GSH, levels; nanosilver toxicity was partially or completely solved by applying vitamin C to the fly. Lumbriculus variegates is a worm that has been used to study the extent to which fullerene-spiked sediments may cause adverse effects.136 In this report, sediments were spiked with 10 and 50 mg fullerenes per kg sediment dry mass and toxicity assessed using the following endpoints: mass change, reproduction, number of organisms, feeding rates, and morphological changes using transmission electron microscopy (TEM). Fullerenes did not cause any impact on the survival or reproduction regarding the control group but their feeding rate and depuration were reduced for worms exposed to themselves. It is noteworthy that fullerenes accumulated in the digestive tract but they were not absorbed by gut epithelial cells.
5.2.3. Plants.
This group of living organisms is highly affected by NP release and consequently can provide unique information regarding transport pathways. Similarly, they can be an important bioaccumulation pathway into the food chain. The main studies carried out with plants have reported the effects caused on seed germination, and root and plant growth. A study about these considerations in several types of plants (radish, rape, ryegrass, lettuce, corn, and cucumber) revealed that none of the used NPs (multi-walled carbon nanotube, aluminum, alumina, zinc, and zinc oxide) caused any effect on seed germination except in the case of Zn and ZnO NPs which inhibited ryegrass and corn species respectively.137 Another study using Cu NPs showed their bioavailability and toxicity.138 It was also shown that bioaccumulation increased with increasing concentration of copper NPs. An interesting work to discuss the mode of entry, formation, transport, and effects of different NPs in plants, as well as their ultimate effects as a consequence of their intensive use nowadays has been already published.9Pseudokirchneriella subcapitata microalgae have been the target organisms to carry out the studies on ZnO, TiO2 and, CuO NP toxicity in comparison to bulk formulations of metal oxides such as ZnSO4 and CuSO4.8 From the results it was concluded that zinc oxides were equally toxic in the bulk and in nano formulations and TiO2 and CuO NPs themselves were significantly more toxic to algae. The highest toxicity was exhibited by nano-ZnO followed by nano-CuO and nano-TiO2. The fact that CuO NPs are also more soluble and more toxic than bulk CuO demonstrates that the toxic effect was solely due to copper ions. In contrast, nano-TiO2 formed aggregates that were entrapped by algae cells. Thus, solubility proved to be a key issue in the toxicity of metal-containing nanoparticles in plants. An indicator organism commonly used in phytotoxicity studies of NPs is Allium cepa that has a well-developed root system in a hydroponic culture. Ghodake et al. noted several adverse effects when A. cepa was exposed to fresh solutions of zinc and cobalt oxide NPs.139 The nano-sized cobalt and zinc oxide particles are able to permeate A. cepa roots and affect the roots' elongation, metabolism and genetic materials. The phytotoxicity of the oxide NPs was evident and increased with the increasing concentrations. Genotoxicity of multi-walled carbon nanotubes, MWCNTs, was also studied on A. Cepa. The internalization of MWCNTs within the plant cells was confirmed by the presence of a large number of black dots distributed throughout the cytoplasm. MWCNTs caused chromosomal aberrations, DNA fragmentation and apoptosis in Allium root cells. These findings also indicate the importance to consider the plants as an important component of the ecosystem when evaluating toxicity of engineered nanomaterials. Another organism model in nanoscience is the freshwater Cnidaria Hydrozoa. Hydra is sensitive to a range of pollutants and has been used as a biological indicator of water pollution.140 Hydra is one of the most sensitive species among the aquatic model organisms. Blaise et al. examined the toxicity of 11 NPs (copper zinc iron oxide, nickel zinc iron oxide, yttrium iron oxide, titanium dioxide, strontium ferrite, indium tin oxide, samarium oxide, erbium oxide, holmium oxide, fullerene-C60 and single-walled carbon nanotube or SWCNT) in a test battery of aquatic biotests.141 They found values of EC50 in the 0.1–1 mg L−1 range for inorganic NPs: copper and zinc iron oxides, indium tin oxides and holmium oxide NPs; and EC50 in the 1–10 mg L−1 range for single-wall carbon nanotubes. Tino et al. studied the toxicological effects of fluorescent CdTe QDs, presenting different coating, thioglycolic acid and glutathione, on Hydra vulgaris.142 The effects on animal behaviour and morphology were investigated over different incubation times and LC50 values were quantitatively estimated. They demonstrated that the interaction of both types of QDs with Hydra induced progressive changes in cell morphology, leading finally to cell and animal death after 72 hours of exposure. This cytotoxicity was associated with QD exposure time and concentration and with the surface chemistry and coating of the QD.
5.2.4. Aquatic organisms.
The toxicity and impact studies carried out with NPs have been performed mostly in different aquatic organisms. Several models to predict acute toxicity to aquatic vertebrates have been employed.
Due to its high homology with the human genome, the freshwater Zebrafish is commonly employed as a vertebrate model to evaluate nanotoxicity. Studies on AgNP transport and effects carried out at the early embryonic stage revealed that biocompatibility and toxicity of AgNPs were highly dependent on their concentration or dose.143 A similar study, in which AgNPs66 were used, showed the direct dependence of some deleterious effects in zebrafish embryos (by means of mortality, hatching delay, pericardial oedema and heart rate) on NP concentration. NP size and shape have been reported as two parameters affecting toxicity. A study with nickel NPs showed that the toxicity by NPs of different sizes, i.e., dendritic aggregates larger than 60 nm and soluble nickel, was very similar. However, they noted that nickel NP shape had greater influence than size on toxicity.86 Another well-established parameter associated with toxicity is the addition of capping agents employed in NP stabilization. Powers et al. compared the effects of ionic silver, citrate-coated AgNPs, and polyvinylpyrrolidone-coated Ag-NPs in zebrafish embryos and larvae.144 The dysmorphology and loss of viability in the embryos were less remarkable with the exposure to AgNPs in comparison with exposure to Ag+; however, AgNPs led to neurodevelopment impairment highly dependent on particle coating and size. Similar experiments were performed by testing sub-lethal effects of TiO2 bulk and TiO2 NPs in the same fish species.145 Results showed a noticeable increase of Ti concentration for both chemical forms of titanium. Interestingly, concentrations returned to control levels by the end of the experiment. Furthermore, limited oxidative stress and organic pathology were observed for an exposure time of 14 days, but both chemical forms led to a decreased embryo viability.
Medaka fish is a species very similar to zebrafish that is also extensively used in toxicity studies. The toxic behaviour and environmental impact of AgNPs were evaluated with Medaka fish.93 Changes in the expression of stress-related genes after exposure to different concentrations and various silver species (AgNPs and AgNO3) were evaluated. Both ionic and AgNPs caused cellular and DNA damage, being carcinogenic agents and responsible for oxidative stress. AgNPs induced certain genes related to metal detoxification/metabolism regulation and radical scavenging action, while ionic silver induced an inflammatory response and a metallic detoxification process in the liver of the fish.
The fathead minnow is another living organism used for testing the effects of AgNPs in comparison to dissolved ions.146 No relation between NP size and mortality rates was found. Interestingly, the evaluation of two different procedures for NP preparation (sonication and stirring) showed significant increase in embryo mortality when exposed to sonicated NPs with respect to nanoparticles that had been previously stirred.147 Furthermore, the same authors noticed higher toxicity when the silver species was AgNO3 in comparison with AgNPs, which induced concentration-dependent larval abnormalities, mostly oedema.
Daphnia species (magna, duplex) are an ecologically and relevant group of organisms, as an important part of the food chain.148 These aquatic organisms have been chosen to cover a range of trophic and taxonomic levels in many reports. For instance, the exposure to several NPs (silver, copper, nickel, and titanium dioxide) caused toxicity in all cases, with AgNPs being the most toxic in terms of LC50. On the other hand, titanium dioxide NPs did not cause toxicity in any of the organisms tested.117 Considering LC50, filter-feeding invertebrate organisms showed higher susceptibility to nanometals than larger organisms. The authors conclude that the toxicity of nanometals does not appear to be a generic response to nanosize particle exposure, but rather, each particular nanometal has an intrinsic property conferring toxicity. Thus, chemical composition of NPs seems to be a relevant parameter that should be taken into consideration when assessing toxicity.
Arenicola marina (lugworm) is a particular organism used for evaluating its exposure to the NPs present in the sediments. The lugworm ingests large amounts of sediments; for this reason significant effects should be expected. Galloway et al. evaluated the adverse effects from sediments containing high amounts of nano-titanium dioxide and carbon nanotubes for A. marina.149 A significant impact was observed in organisms exposed to TiO2 NPs; however, nanotubes were not found in the gut lumen after a 24 hour starving period, suggesting that these particles either remain in the sediment or pass through the gut and are excreted. In contrast, TiO2 NPs, with a high tendency to form aggregates, were genotoxic due to the generation of free radical species that can react with most DNA components.
5.2.5. Mice.
The mouse has become the model experimental animal par excellence, and there is a huge amount of literature available on it. Furthermore, the increasing use of NPs in biomedicine has promoted more studies on toxic effects in the last few decades. The effects of gold nanoparticles, AuNPs, have been studied in these animals through the assessment of animal survival, weight, haematology, morphology, and organ index.35 Results have showed that low concentrations of AuNPs do not cause any obvious decrease in body weight or induce an appreciable toxicity; but higher concentrations (500–2000 μg kg−1) induced loss in body weight and a decrease in red blood cells. Intraperitoneal administration of different AuNP concentrations revealed an increase in gold concentration in organs, while it remained constant in blood.150 Thus, the AuNPs administered intraperitoneally were absorbed into systemic circulation and distributed into tissues, indicating that AuNPs have a high capacity to accumulate in tissues. The brain was the organ with the highest accumulation capacity, suggesting a non-saturable uptake of AuNPs from the blood to the brain. Additionally, an efficient clearance of AuNPs from the body occurred at elevated doses. However, intravenous administration of AuNPs revealed accumulation in several organs such as the kidneys, lungs, brain, liver, and spleen, but especially liver.151 Authors noted that PEG-coated AuNPs induced acute inflammatory response and apoptosis in the liver of mice.
The sub-cellular accumulation of superparamagnetic iron oxide NPs has been studied in breast tumours and peripheral organs.152 Cluster formation of high-density NPs in cancer cells and their accumulation via conjugation to receptors was observed. These results showed that iron NP conjugates have potential applications in breast cancer detection and treatment.
Carbon nanotube toxicity has also been assessed in mice.153 The cytotoxicity and genotoxicity were among the parameters studied, as well as the inflammatory response and intracellular production of ROS. An intracellular production of ROS and DNA damage were detected and attributed to oxidative DNA damage. Carbon nanotubes caused necrosis and oxidative stress due to incomplete phagocytosis or mechanical damage of the plasma membrane. The results obtained by TEM evidenced that macrophages were able to phagocytize and internalize the nanotubes in phagolysosomes. Therefore, it was shown that genotoxicity of CNT's was greater than that of carbon black particles, causing double- or single-strand damage. Other experiments performed with organic polymeric NPs, carried out using rat macrophage cell lines, evidenced uptake upon incubation for 2 h at the μg L−1 concentration level,154 as well as signs of autophagy, apoptosis, and oxidative stress.155 These results indicate that polymeric NPs are able to cross the mitochondrial membranes and enter the organelles inducing a cascade of events such as the unbalance of the oxidant/antioxidant homeostasis and the modification of the gene and protein expression.
Aluminum oxide NPs have been studied at the nanoscale level due to their easy access to the central nervous system. Effects of these NPs have been evaluated in rat brains after peripheral exposure.156 Li et al. analyzed the activation of microglia and astrocytes (dominant and major immune cells in the CNS considered a key factor in many pathological alterations and inflammatory diseases) after exposure to aluminium oxide NPs. The authors observed that nanoscale aluminum oxide could induce the activation of these cells in various regions in the brains of rats. Furthermore, NPs were able to produce significant inflammatory effects in the brain of the rat, while both nanoscale and non-nanoscale aluminum oxide NPs had almost the same inflammatory effect on the liver and kidney.
5.2.6. Humans.
This is the final link in the food chain for toxicity and bioavailability tests. Despite the fact that there are not many research works, some conclusions are related to oxidative stress and apoptosis caused by some types of NPs when the exposure occurs at elevated concentration levels. Some studies carried out with silver NPs in human cells resulted in: (i) an increase of intracellular ROS and mitochondrial damage associated with ATP157 depletion, and (ii) oxidative stress as a consequence of the depletion of glutathione (GSH), which led to the increase of lipid peroxidation.158 Carbon based nanomaterials also present ability to generate oxygen radicals responsible for cell death. Sayes et al. demonstrated the formation of superoxide species in human cells treated with several water soluble fullerene species. It was the first cytotoxicity study of buckballs in human cells.159 Several water-soluble fullerenes (sublimed C60 and C60(OH)24, C3 and Na+2−3[C60O7–9(OH)12–15](2–3)-) were tested in two different human cell lines, human dermal fibroblasts (HDF) and human liver carcinoma (HepG2) cells. For cells exposed to nano-C60 cell death occurred because of lipid oxidation caused by the generation of oxygen radicals; more highly derivatized C60 systems were not as facile at generating these species and thus had lower cellular toxicity. Their findings contributed to the strategy for enhancing the toxicity of fullerenes for certain applications such as cancer therapeutics, as well as remediation for the possible unwarranted effects of pristine fullerenes.
On the other hand, quantum dots have been the focus of interest for different human cell line studies due to the increased use of these NPs for diagnostic and biomedical purposes.160 Ryman-Rasmussen et al. studied the ability of quantum dots to penetrate skin.161 Different coatings and structural characteristics were evaluated. The level of penetration and kinetics were highly dependent on the nature of the NP coatings. They speculated with a passive diffusion mechanism of entry, most likely via an intercellular route of passage, meaning that the skin is permeable to nanomaterials depending on diverse physicochemical properties. Cytotoxicity of CdTe QDs has been investigated in a human breast cancer cell line (MCF-7).162 Several biological responses were determined by assessing cell apoptosis: plasma membrane stability, mitochondrial and nuclear damage, generation of ROS, release of cytochrome C in mitochondria. The final observations revealed damage to the mitochondria membrane and nucleus mediated by ROS (that could be prevented by treatments with some antioxidants). Another study performed in lung cancer cells after exposure to cerium oxide NPs showed decreased cell viability depending on NP dose and exposure time.163
An interesting biomedical research work on the selectivity and toxicity of ZnO NPs towards Gram-positive bacteria and cancer cells was proposed by Premanathan et al.164 Their findings support the fact that ZnO induced toxicity in a specific cell and their proliferation were dependent on NP characteristics. The obtained results may be of relevant clinical interest as novel alternatives for cancer chemotherapy. The wide variability of observed NP effects confirms that unexpected and harmful responses in biological organisms exposed to NPs may occur. Thus, further thorough studies on their wide range of effects are needed before continuing with their extensive production and uses.
6. Conclusions
The available information on toxicity and NP accumulation by living organisms is still confusing and the available results are difficult to compare. Here we have included several studies assessing the potential risk of NPs, as well as the controversial effects found indicating that many important factors affecting the toxicity of NPs are not yet well understood. Despite many studies being assessed to evaluate the impact from NPs, there are no clear conclusions on their associated risks. Further studies need to be developed in order to understand and predict their possible impact and hazards to health and environment.
Development of analytical methodologies for nanomaterial characterization and quantification as well as their monitoring in different environmental and biological samples are mandatory. The techniques that provide the most relevant information are: TEM, which provides the most direct information on size distribution and shape of particles but can alter NP properties; DLS, which allows obtaining measurement rapidly, but has several pitfalls because dust particles or small amounts of large aggregates may cause interferences; ultrafiltration and ultracentrifugation, which give sufficient information regarding NP stability in the media; ICP-MS is a good quantification technique when coupled to AF4 or other separation methods, etc. The scientific community agrees that using only one technique is not enough to get a complete picture of the difficulties caused by NPs. Another key subject is the need for the implementation of prediction models on their fate and behaviour within the environment. Further environmental risks by these particles will have to be tested under regulatory schemes such as REACH, to set regulations fixing the limits at which nanomaterials can be found in the environment.
To avoid irreversible consequences on health and on the environment, studies on the impact of NPs must be carried out before looking for other applications of these novel materials. Furthermore, the elaboration of an appropriate legislation, as well as establishing laws regulating their release, in order to avoid further episodes of possible negative consequences on humans and other organisms, is mandatory.
7. References
- Y. Ju-Nam and R. J. Lead, Sci. Total Environ., 2008, 400, 396–414 CrossRef CAS PubMed.
- E. K. Rushton, J. Jingkun, L. Stephen, S. Eberly, V. Castranova, P. Biswas, A. Elder, X. Han, R. Gelein, J. Finkelstein and G. Oberdoerster, J. Toxicol. Environ. Health, Part A, 2010, 73(5–6), 445–461 CrossRef CAS PubMed.
- A. Nel, T. Xia, L. Madler and N. Li, Science, 2006, 311, 622–627 CrossRef CAS PubMed.
- S. Arora, J. M. Rajwade and K. M. Paknikar, Toxicol. Appl. Pharmacol., 2012, 258, 151–165 CrossRef CAS PubMed.
- K. Donaldson, V. Stone, C. L. Tran, W. Kreyling and P. J. Borm, Occup. Environ. Med., 2004, 61(9), 727–728 CrossRef CAS PubMed.
- R. J. Griffitt, J. Luo, J. Gao, J. C. Bonzango and D. S. Barber, Environ. Toxicol. Chem., 2008, 27(9), 1972–1978 CrossRef CAS PubMed.
- V. Aruoja, H. C. Dubourguier, K. Kasemets and A. Kahru, Sci. Total Environ., 2009, 407, 1461–1468 CrossRef CAS PubMed.
- R. Nair, S. H. Varghese, G. Baiju, T. Maekawa, Y. Yoshida and D. S. Kumar, Plant Sci., 2010, 179, 154–163 CrossRef CAS PubMed.
- X. Ma, J. Geiser-Lee, Y. Deng and A. Kolmakov, Sci. Total Environ., 2010, 408, 3053–3061 CrossRef CAS PubMed.
- M. A. Dobrovolskaia, A. K. Patri, J. Zheng, J. D. Clogston, N. Ayub, P. Aggarwal, B. W. Neun, J. B. Hall and S. E. McNeil, Nanomed.: Nanotechnol., Biol. Med., 2009, 5, 106–117 CrossRef CAS PubMed.
- J. Robbensa, C. Vanparysb, I. Nobelsb, R. Blustb, K. Van Hoeckec, C. Janssenc, K. De Schamphelaerec, K. Rolandd, G. Blanchardd, F. Silvestre, V. Gillardin, P. Kestemontd, R. Anthonissene, O. Toussaintf, S. Vankoningsloof, C. Saoutf, E. Alfaro-Morenog, P. Hoetg, L. Gonzalezh, P. Dubrueli and P. Troisfontaines, Toxicology, 2010, 269, 170–181 CrossRef PubMed.
- G. Oberdörster, A. Maynard, K. Donaldson, V. Castranova, J. Fitzpatrick, K. Ausman, J. Carter, B. Karn, W. Kreyling, D. Lai, S. Olin, N. Monteiro-Riviere, D. Warheit and H. Yang, Part. Fibre Toxicol., 2005, 2(8), 1–35 Search PubMed.
- H. V. Kroto, J. R. Heath, S. C. O'Brien, R. F. Curl and R. E. Smalley, Nature, 1985, 318, 162–163 CrossRef CAS.
- S. H. Friedman, D. L. De Camp, R. P. Sijbesma, G. Srdanov, F. Wudl and G. L. Kenyon, J. Am. Chem. Soc., 1993, 115, 6506–6509 CrossRef CAS.
- N. C. Mueller and B. Nowack, Environ. Sci. Technol., 2008, 42, 4447–4453 CrossRef CAS.
- K. K. Upadhyayula, S. Deng, M. C. Mitchell and G. B. Smith, Sci. Total Environ., 2009, 408, 1–13 CrossRef PubMed.
- H. Jang and S. Kim, J. Nanopart. Res., 2007, 3, 141–147 CrossRef.
- M. Auffan, J. Rose, T. Orsiere, M. de Meo, A. Thill, O. Zeyons, O. Proux, A. Masion, P. Chaurand, O. Spalla, A. Botta, M. R. Wiesner and J. Y. Bottero, Nanotoxicology, 2009, 3(2), 161–171 CrossRef CAS.
- K. Zhou, R. Wang, B. Xu and Y. Li, Nanotechnology, 2006, 17, 3939–3943 CrossRef CAS.
- J. Cho, M. S. Joshi and C. T. Sun, Compos. Sci. Technol., 2006, 66, 1941–1952 CrossRef CAS PubMed.
- Y. Ding, H. Chen, Z. Musina, Y. Jin, T. Zhang, S. Witharana and W. Yang, Phys. Scr., 2010, 139, 014078 CrossRef.
- A. K. Gupta and M. Gupta, Biomaterials, 2005, 6, 3995–4021 CrossRef PubMed.
- D. R. Larson, W. R. Zipfel, R. M. Williams, S. W. Clark, M. P. Bruchez and F. W. Wise, Science, 2003, 300, 1434–1436 CrossRef CAS PubMed.
- M. Bruchez, M. Moronne, P. Gin, S. Weiss and A. P. Alivisatos, Science, 1998, 281, 2013–2016 CrossRef CAS.
- W. C. W. Chan and S. Nie, Science, 1998, 281, 2016–2018 CrossRef CAS.
- H. Mattoussi, J. M. Mauro, E. R. Goldman, G. P. Anderson, V. C. Sundar, F. V. Mikulec and M. G. Bawendi, J. Am. Chem. Soc., 2000, 122(49), 12142–12150 CrossRef CAS.
- B. Ferreira da Silva, S. Perez, P. Gardinalli, R. K. Singhal, A. Mozeto and D. Barceló, TrAC, Trends Anal. Chem., 2011, 30(3), 528–540 CrossRef CAS PubMed.
- A. R. Shahverdi, A. Fakhimi, H. R. Shahverdi and S. Minaian, Nanomed.: Nanotechnol., Biol. Med., 2007, 3(2), 168–171 CrossRef CAS PubMed.
- T. M. Benn and P. Westerhoff, Environ. Sci. Technol., 2008, 42, 4133–4139 CrossRef CAS.
- B. Sadeghi, M. Jamali, Sh. Kia, A. Amininia and S. Ghafari, Int. J. Nano Dimens., 2010, 1(2), 119–124 Search PubMed.
- K. D. Grieger, A. Fjordboge, N. B. Hartmann, E. Eriksson, P. L. Bjerg and A. Baun, J. Contam. Hydrol., 2010, 118(3–4), 165–183 CrossRef CAS PubMed.
- Y. Wu and S. Hu, Bioelectrochemistry, 2007, 70, 335–341 CrossRef CAS PubMed.
-
Colloidal, in Gold: Principles, Methods, and Applications, ed. M. A. Hayat, Academic Press, San Diego, 1989, vol. 1–2 Search PubMed.
- X. D. Zhang, H. Y Wu, D. Wu, Y. Y. Wang, J. H. Chang, Z. B. Zhai, A. M. Meng, P. X. Liu, L. A. Zhang and F. Y. Fan, Int. J. Nanomed., 2010, 5, 771–781 CrossRef CAS PubMed.
- C. Roney, P. Kulkarni, V. Arora, P. Antich, F. Bonte, A. Wu, N. M. Mallikarjuana, S. Manohar, H. F. Liang, A. R. Kulkarni, H. W. Sung, M. Sairam and T. M. Aminabhavi, J. Controlled Release, 2005, 108, 193–214 CrossRef CAS PubMed.
- R. Schirhagl, U. Latif, D. Podlipna, H. Blumenstock and F. L. Dickert, Anal. Chem., 2012, 84(9), 3908–3913 CrossRef CAS PubMed.
- J. Wang, G. Liu, R. Polsky and A. Merkoçi, Electrochem. Commun., 2002, 4(9), 722–726 CrossRef CAS.
- D. Panatarotto, C. D. Protidos, J. Hoebeke, F. Brown, E. Kramer, J. P. Briand, S. Muller, M. Prato and A. Bianco, Chem. Biol., 2003, 10, 961–966 CrossRef PubMed.
- K. Bowman and K. W. Leona, Int. J. Nanomed., 2006, 1(2), 117–128 CrossRef CAS.
- S. C. G. Kiruba Daniel, V. Tharmaraj, T. A. Sironmani and K. Pitchumani, Appl. Clay Sci., 2010, 48, 547–551 CrossRef PubMed.
- J. M. Nam, C. C. Thaxton and C. A. Mirkin, Science, 2003, 301, 1884–1886 CrossRef CAS PubMed.
-
Y. Lin, J. Wang, H. Wang, H. Wu and Z. Tang, in Chemical Sensors 8: Chemical Sensors and Analytical Systems. ECS Transactions, ed. R. Mukundan, et al., 2008, vol. 16:11, pp. 477–482 Search PubMed.
- R. Mahtab, J. P. Rogers and C. J. Murphy, J. Am. Chem. Soc., 1995, 117, 9099–9100 CrossRef CAS.
- L. Wang, J. Zhang, X. Wang, Q. Huang, D. Pan, S. Song and C. Fan, Gold Bull., 2008, 41(1), 37–41 CrossRef CAS.
- J. M. Pérez, F. J. Simeone, Y. Saeki, L. Josephson and R. Weissleder, J. Am. Chem. Soc., 2003, 125(34), 10192–10193 CrossRef PubMed.
- W. Tungittiplakorn, L. W. Lion, C. Cohen and A. Y. Kim, Environ. Sci. Technol., 2004, 38(5), 1605–1610 CrossRef CAS.
- V. K. K. Upadhyayula, S. Deng, G. B. Smith and M. C. Mitchell, Water Res., 2009, 43, 148–156 CrossRef CAS PubMed.
- M. Khodakovskaya, E. Dervishi, M. Mahmood, Y. Xu, Z. Li, F. Watanabe and A. S. Biris, ACS Nano, 2009, 3(10), 3221–3227 CrossRef CAS PubMed.
- L. Zheng, F. Hong, S. Lu and C. Liu, Biol. Trace Elem. Res., 2005, 104(1), 83–92 CrossRef CAS.
- M. D. S. Silva, D. S. Cocenza, R. Grilloa, N. F. S. de Meloa, P. S. Tonello, L. C. Oliveira, D. L. Cassimiro, A. H. Rosa and L. F. Fraceto, J. Hazard. Mater., 2011, 190(1–3), 366–374 CrossRef CAS PubMed.
-
S. U. S. Choi, and J. A. Eastman, Contract W-31-109-ENG-38 of the Energy Technology Division and Materials Science Division, San Francisco, CA, ASME International Mechanical Engineering Congress & Exposition, November 12-17, San Francisco, CA, 1995.
- R. Peters, G. ten Dam, H. Bouwmeester, H. Helsper, G. Allmaier, F. vd Kammer, R. Ramsch, C. Solans, M. Tomainová, J. Hajslova and S. Weigel, TrAC, Trends Anal. Chem., 2011, 30(1), 100–112 CrossRef CAS PubMed.
- J. O. Carneiro, S. Azevedo, V. Teixeira, F. Fernandes, E. Freitas, H. Silva and J. Oliveira, Constr. Build. Mater., 2013, 38, 594–601 CrossRef PubMed.
- M. Berra, F. Carassiti, T. Mangialardi, A. E. Paolini and M. Sebastiani, Constr. Build. Mater., 2012, 35, 666–675 CrossRef PubMed.
-
C. Kumar, Nanomaterials-Toxicity, Health and Environmental Issues, Wiley-VCH, Weinheim, Germany, 2006 Search PubMed.
- V. Stone, B. Nowack, A. Baun, N. van den Brink, F. von der Kammer, M. Dusinska, R. Handy, S. Hankin, M. Hassellöv, E. Joner and T. F. Fernández, Sci. Total Environ., 2010, 408, 1745–1754 CrossRef CAS PubMed.
- T. P. J. Linsinger, Q. Chaudhry, V. Dehalu, P. Delahaut, A. Dudkiewicz, R. Grombe, F. von der Kammer, E. H. Larsen, S. Legros, K. Loeschner, R. Peters, R. Ramsch, G. Roebben, K. Tiede and S. Weigel, Food Chem., 2013, 138, 1959–1966 CrossRef CAS PubMed.
- S. A. Love, M. A. Maurer-Jones, J. W. Thompson, Y. S. Lin and C. L. Haynes, Annu. Rev. Anal. Chem., 2012, 5, 181–205 CrossRef CAS PubMed.
- P. A. Maurice, Colloids Surf., A, 1996, 107, 57–75 CrossRef CAS.
- G. Friedbacher and H. Fuchs, Pure Appl. Chem., 1999, 71(7), 1337–1357 CrossRef CAS.
-
J. I. Goldstein, D. E. Newbury, P. Echlin, D. C. Joy and A. D. Roming, Scanning electron microscopy and X-Ray Microanalysis, Plenum Press, New York, 2nd edn, 1992 Search PubMed.
- K. Tiede, S. P. Tear, H. David and A. B. A. Boxall, Water Res., 2009, 43, 3335–3343 CrossRef CAS PubMed.
- B. Ferreira da Silva, S. Pérez, P. Gardinalli, R. K. Singhal, A. A. Mozeto and D. Barceló, TrAC, Trends Anal. Chem., 2011, 30(3), 528–540 CrossRef CAS PubMed.
- B. Zanetti-Ramos, M. B. Fritzen-Garcia, C. S. de Oliveira, A. A. Pasa, V. Soldi, R. Borsali and T. B. Creczynski-Pasa, Mater. Sci. Eng., C, 2009, 29, 638–640 CrossRef CAS PubMed.
-
B. Chu, Laser Light Scattering, Academic Press, New York, 2nd edn, 1991 Search PubMed.
- D. Mavrocordatos, W. Pronk and M. Boller, Water Sci. Technol., 2004, 50, 9–18 CAS.
- J. T. Nurmi, P. G. Tratnyek, V. Sarathy, D. R. Baer, J. E. Amonette, K. Pecher, C. M. Wang, J. C. Linehan, D. W. Matson, R. L. Penn and M. D. Driessen, Environ. Sci. Technol., 2005, 39, 1221–1230 CrossRef CAS.
- M. C. Daniel and D. Astruc, Chem. Rev., 2004, 104, 293–346 CrossRef CAS PubMed.
- P. V. Asharani, Y. L. Wu, Z. Gong and S. Valiyaveettil, Nanotechnology, 2008, 19, 1–8 CrossRef PubMed.
- V. Filipe, A. Hawe and W. Jiskoo, Pharm. Res., 2010, 27(5), 796–810 CrossRef CAS PubMed.
- A. R. Badireddy, M. R. Wiesner and J. Liu, Environ. Sci. Technol., 2012, 46(18), 10081–10088 CAS.
-
N. Fairbairn, R. Fernandes, R. Carter, T. J. Elliott, A. G. Kanaras and O. L. Muskens, Colloidal Nanocrystals for Biomedical Applications, Proc. SPIE 8595, 22 February 2013, vol. VIII, p. 859501, DOI:10.1117/12.981941.
- J. B. Chao, J. F. Liu, S. J. Yu, Y. D. Feng, Z. Q. Tan, R. Liu and Y. G. Yin, Anal. Chem., 2011, 83, 6875–6882 CrossRef CAS PubMed.
- G. Hartmann, C. Hutterer and M. Schuster, J. Anal. At. Spectrom., 2013, 28, 567–572 RSC.
- Z. Zhaoxiang, L. Weixing, X. Weihong and X. Nanping, Sep. Purif. Technol., 2011, 76, 223–230 CrossRef PubMed.
- A. Ulrich, S. Losert, N. Bendixen, A. Al-Kattan, H. Hagendorfer, B. Nowack, C. Adlhart, J. Ebert, M. Lattuada and K. Hungerbühler, J. Anal. At. Spectrom., 2012, 27, 1120–1130 RSC.
-
M. Hassellöv, F. Vn der Kammer and R. Becket, in Environmental Colloids and Particles: Behaviour, Structure and Characterization, ed. K. J. Wilkinson and J. R. Lead, Wiley, Chichester, 2007, pp. 223–276 Search PubMed.
- V. Nischwitz and H. Goenaga-Infante, J. Anal. At. Spectrom., 2012, 27, 1084–1092 RSC.
- A. B. Schmidt, K. Lieschner, N. Hadrup, A. Mortensen, J. Slot, C. Bender and E. H. Larsen, Anal. Chem., 2011, 83, 2461–2468 CrossRef PubMed.
- H. Hagendorfer, R. Kaegi, M. Parlinska, B. Sinnet, C. Ludwig and A. Ulrich, Anal. Chem., 2012, 84(6), 2678–2685 CrossRef CAS PubMed.
- N. M. Thang, R. Knopp, H. Geckeis, J. I. Kim and H. P. Beck, Anal. Chem., 2000, 72, 1–5 CrossRef CAS.
- J. C. Trefry, J. L. Monahan, K. M. Weaver, A. J. Meyerhoefer, M. M. Markopolous, Z. S. Arnold, D. P. Wooley and L. E. Pavel, J. Am. Chem. Soc., 2010, 132, 10970–10972 CrossRef CAS PubMed.
- G. Dalwadi and V. Bruce Sunderland, Drug Dev. Ind. Pharm., 2007, 33(9), 1030–1039 CrossRef CAS PubMed.
- D. M Luykx, R. J. B. Peters, S. M. Ruth and H. Bouwmeester, J. Agric. Food Chem., 2008, 56(18), 8231–8247 CrossRef PubMed.
- A. Bootz, V. Vogel, D. Schubert and J. Kreuter, Eur. J. Pharm. Biopharm., 2004, 57(2), 369–375 CrossRef CAS.
- W. Guor-Tzoi, L. Fu-Ken and C. R. Wang, Anal. Chem., 1999, 71, 2085–2091 CrossRef PubMed.
- A. Williams, E. Varela, E. Meehan and K. Tribe, Int. J. Pharm., 2002, 242, 295–299 CrossRef CAS.
- J. Soto-Alvaredo, M. Montes-Bayón and J. Bettmer, Anal. Chem., 2013, 85, 1316–1321 CrossRef CAS PubMed.
- W. M. Hwang, C. Y. Lee, D. W. Boo and J. G. Choi, Bull. Korean Chem. Soc., 2003, 24(5), 684–686 CrossRef CAS.
- R. S. H. Yang, L. W. Chang, J. P. Wu, M. H. Tsai, H. J. Wang, Y. C. Kuo, T. K. Yeh, C. S. Yang and P. Lin, Environ. Health Perspect., 2007, 115(9), 1339–1343 CrossRef CAS PubMed.
- I. P. Matthews, C. J. Gregory, G. Aljayyoussi, C. J. Morris, I. Mcdonald, B. Hoogendoorn and M. Gumbleton, Environ. Toxicol. Pharmacol., 2013, 3(5), 461–464 CrossRef PubMed.
- A. Scheffer, C. Engelhard, M. Sperling and W. Buscher, Anal. Bioanal. Chem., 2008, 390, 249–252 CrossRef CAS PubMed.
- C. Ispas, D. Andreescu, A. Patel, D. V. Goia, S. Andreescu and K. N. Wallace, Environ. Sci. Technol., 2009, 43, 6349–6356 CrossRef CAS.
- C. Degueldre and P. Y. Favarger, Talanta, 2004, 62(5), 1051–1054 CrossRef CAS PubMed.
- S. Gschwind, L. Flamigni, J. Koch, O. Borovinskaya, S. Groh, K. Niemax and D. Gunther, J. Anal. At. Spectrom., 2011, 26(6), 1166–1174 RSC.
- J. Tuoriniemi, G. Cornelis and M. Hassellöv, Anal. Chem., 2012, 84, 3965–3972 CrossRef CAS PubMed.
- J. Gigault and V. A. Hackley, Anal. Chim. Acta, 2013, 763, 57–66 CrossRef CAS PubMed.
- S. Elzey, D.-H. Tsai, L. L. Yu, M. R. Winchester, M. E. Kelley and V. A. Hackley, Anal. Bioanal. Chem., 2013, 405, 2279–2288 CrossRef CAS PubMed.
- J. S. Suleiman, B. Hu, X. Pu, C. Huang and Z. Jiang, Microchim. Acta, 2006, 159(3–4), 379–385 Search PubMed.
- Y. J. Chae, C. H. Pham, J. Lee, E. Bae, J. Yi and M. B. Gu, Aquat. Toxicol., 2009, 94(4), 320–327 CrossRef CAS PubMed.
- H. Fischer, S. Fournier-Bidoz, K. Pang and W. Chan, NanoBiotechnology, 2007, 3(1), 46–54 CrossRef CAS PubMed.
- B. D. Chithrani and W. C. W. Chan, Nano Lett., 2007, 7, 1542–1550 CrossRef CAS PubMed.
- Z. Chen, P. Westerhoff and P. Herckes, Environ. Toxicol. Chem., 2008, 27(9), 1852–1859 CrossRef CAS.
- C. Wanga, C. Shanga and P. Westerhoff, Chemosphere, 2010, 80, 334–339 CrossRef PubMed.
- R. Kubotaa, M. Taharaa, K. Shimizu, N. Sugimoto, A. Hirose and T. Nishimura, Toxicol. Lett., 2011, 206, 172–177 CrossRef PubMed.
- C. W. Isaacson, C. Y. Usenko, R. L. Tanguay and J. A. Field, Anal. Chem., 2007, 79, 9091–9097 CrossRef CAS PubMed.
-
D. A. Cremers and L. J. Radziemski, Handbook of Laser-Induced Breakdown Spectroscopy, John Wiley & Sons, Ltd, West Sussex, England, 2006 Search PubMed.
- M. Hasselöv, J. W. Readman, J. F. Ranville and K. Tiede, Ecotoxicology, 2008, 17, 344–361 CrossRef PubMed.
- N. M. Thang, R. Knopp, H. Geckeis, J. I. Kim and H. P. Beck, Anal. Chem., 2000, 72, 1–5 CrossRef CAS.
- J. Liu and R. H. Hurt, Environ. Sci. Technol., 2010, 44, 2169–2175 CrossRef CAS PubMed.
- M. Resano, A. C. Lapeña and M. A. Belarra, Anal. Methods, 2013, 5, 1130–1139 RSC.
- J. Pauluhn, Toxicol. Sci., 2010, 113(1), 226–242 CrossRef CAS PubMed.
- J. Muller, F. Huaux, N. Moreau, P. Misson, J.-F. Heiliera, M. Delos, M. Arras, A. Fonseca, J. B. Nagy and D. Lison, Toxicol. Appl. Pharmacol., 2005, 207(3), 221–231 CrossRef CAS PubMed.
- B. Joo, R. Thio, D. Zhou and A. A. Keller, J. Hazard. Mater., 2011, 189, 556–563 CrossRef PubMed.
- M. Tejamaya, I. Römer, R. C. Merrifield and J. R. Lead, Environ. Sci. Technol., 2012, 46, 7011–7017 CrossRef CAS PubMed.
-
C. O. Robichaud, D. Tanzil and M. R. Wiesner, in Environmental Nanotechnology. Applications and Impacts of Nanomaterials, ed. M. R. Wiesner and J. Y. Bottero, McGraw Hill, New York, 2007, pp. 481–524 Search PubMed.
- Y. Zhang, Y. Chen, P. Westerhoff, K. Hristovski and J. C. Crittenden, Water Res., 2008, 42(8–9), 2204–2212 CrossRef CAS PubMed.
- J. Jiang, G. Oberdörster and P. Biswas, J. Nanopart. Res., 2009, 11, 77–89 CrossRef CAS.
- J. Li and R. H. Hurt, Environ. Sci. Technol., 2010, 44, 2169–2175 CrossRef PubMed.
- O. Choi, T. E. Clevenger, B. Deng, R. Y. Surampalli, L. Ross and Z. Hu, Water Res., 2009, 43, 1879–1886 CrossRef CAS PubMed.
- S. L. Chinnapongse, R. I. MacCuspie and V. A. Hackley, Sci. Total Environ., 2011, 409, 2443–2450 CrossRef CAS PubMed.
- J. Kasthuri, S. Veerapandian and N. Rajendiran, Colloids Surf., B, 2009, 68, 55–60 CrossRef CAS PubMed.
- W. Zhang, Z. Chen, H. Liu, L. Zhang, P. Gao and D. Li, Colloids Surf., B, 2001, 88, 196–201 CrossRef PubMed.
- S. Li and W. Sun, J. Hazard. Mater., 2011, 197, 70–79 CrossRef CAS PubMed.
- D. Lin, J. Ji, Z. Long, K. Yang and F. Wu, Water Res., 2012, 46, 4477–4487 CrossRef CAS PubMed.
- G. D. Venkatasubbu, S. Ramasamy, V. Ramakrishnan and J. Kumar, Adv. Powder Technol., 2013 DOI:10.1016/j.apt.2013.01.008.
- F. von der Kammer, S. Ottofuelling and T. Hofmann, Environ. Pollut., 2010, 158, 3472–3481 CrossRef CAS PubMed.
- B. Nowack and T. D. Bucheli, Environ. Pollut., 2007, 150, 5–22 CrossRef CAS PubMed.
- M. A. Maurer-Jones, I. L. Gunsolus, C. J. Murphy and C. L. Haynes, Anal. Chem., 2013, 85, 3036–3049 CrossRef CAS PubMed.
- R. J. Griffitt, J. Luo, J. Gao, J. C. Bonzango and D. S. Barber, Environ. Toxicol. Chem., 2008, 27(9), 1972–1978 CrossRef CAS PubMed.
- SCENIHR: European Commission Health-Consumer Protection Directorate General. http://ec.europa.eu/health/scientific_committees/emerging/index_en.htm.
- H. Zheng, L. Liu, Y. Long, L. Wang, K. P. Ho and K. Y. Wong, Anal. Sci., 2010, 26, 125–128 CrossRef CAS.
- M. Mortimer, K. Kasemets and A. Kahru, Toxicology, 2010, 269, 182–189 CrossRef CAS PubMed.
- K. Kasemets, A. Ivask, H. C. Dubourguier and A. Kahru, Toxicol. in Vitro, 2009, 23, 1116–1122 CrossRef CAS PubMed.
- R. Posgai, C. B. Cipolla-McCulloch, K. R. Murphy, S. M. Hussain, J. J. Rowe and M. G. Nielsen, Chemosphere, 2011, 85, 34–42 CrossRef CAS PubMed.
- K. Pakarinena, E. J. Petersenb, M. T. Leppänena, J. Akkanena and J. V. K. Kukkonena, Environ. Pollut., 2011, 159, 3750–3756 CrossRef PubMed.
- D. Lin and B. Xing, Environ. Pollut., 2007, 150(2), 243–250 CrossRef CAS PubMed.
- W. M. Lee, Y. J. An, H. Yoon and H. S. Kweon, Environ. Toxicol. Chem., 2008, 27(9), 1915–1921 CrossRef CAS.
- G. Ghodake, Y. Deuk Seo and D. S. Lee, J. Hazard. Mater., 2011, 186, 952–955 CrossRef CAS PubMed.
- D. A. Holdway, K. Lok and M. Semaan, Environ. Toxicol., 2001, 16(6), 557–565 CrossRef CAS PubMed.
- C. Blaise, F. Gagne, J. F. Ferard and P. Eullaffroy, Environ. Toxicol., 2008, 23, 591–598 CrossRef CAS PubMed.
- A. Tino, A. Ambrosone, L. Mattera, V. Marchesano, A. Susha, A. Rogach and C. Tortiglione, Int. J. Biomater., 2011, 2011, 1–8 Search PubMed.
- K. J. Lee, P. D. Nallathamby, L. M. Browning, C. J. Osgood and X. H. N. Xu, ACS Nano, 2007, 28(1–2), 133–143 CrossRef PubMed.
- C. M. Powers, T. A. Stlotkin, F. J. Seidler, A. R. Badireddy and S. Padilla, Neurotoxicol. Teratol., 2011, 33(6), 708–714 CrossRef CAS PubMed.
- C. S. Ramsden, T. B. Henry and R. D. Handy, Aquat. Toxicol., 2013, 126, 404–413 CrossRef CAS PubMed.
- A. Mathis and R. J. F. Smith, Anim. Behav., 1993, 46(4), 645–656 CrossRef.
- G. L. Loring, F. N. Ronald, F. Turco, J. W. Bickham and M. S. Sepúlveda, Ecotoxicology, 2010, 19, 185–195 CrossRef PubMed.
- D. M. M. Adema, Hydrobiologia, 1978, 59(2), 135–140 CrossRef.
- T. Galloway, C. Lewis, I. Dolciotti, B. D. Johnston, J. Moger and F. Regoli, Environ. Pollut., 2010, 158, 1748–1755 CrossRef CAS PubMed.
- C. Lasagna-Reeves, D. Gonzalez-Romero, M. A. Barria, I. Olmedo, A. Clos, V. M. Sadagopa-Ramanujam, A. Urayama, L. Vergara, M. J. Kogan and C. Soto, Biochem. Biophys. Res. Commun., 2010, 393, 649–655 CrossRef CAS PubMed.
- W. S Cho, M. Cho, J. Jeong, M. Choi, H. Y. Cho, B. S. Han, S. H. Kim, H. O. Kim, Y. T. Lim, B. H. Chung and J. Jeong, Toxicol. Appl. Pharmacol., 2009, 236, 16–24 CrossRef PubMed.
- J. Zhou, C. Leuschner, C. Kumar, J. F. Hormes and W. O. Soboyejo, Biomaterials, 2006, 27, 2001–2008 CrossRef CAS PubMed.
- M. L. Di Giorgio, S. di Buchianico, A. M. Ragnelli, P. Aimola, S. Santucci and A. Poma, Mutat. Res., Genet. Toxicol. Environ. Mutagen., 2011, 722, 20–31 CrossRef CAS PubMed.
- H. Eidi, O. Joubert, C. Némos, S. Grandemange, B. Mograbi, B. Foliguet, J. Tournebize, P. Maincent, A. Le Faou, I. Aboukhamis and B. H. Rihn, Int. J. Pharm., 2012, 422, 495–503 CrossRef CAS PubMed.
- T. Yoshimori, Biochem. Biophys. Res. Commun., 2004, 313, 453–458 CrossRef CAS PubMed.
- X.-b. Li, H. Zheng, Z.-r. Zhang, M. Li, Z.-y. Huang, H. J. Schulesener, Y.-y. Li and S.-q. Xu, Nanomed.: Nanotechnol., Biol. Med., 2009, 5, 473–479 CrossRef CAS PubMed.
- S. Arora, J. Jain, J. M. Rajwade and K. M. Palknikar, Toxicol. Lett., 2008, 179, 93–100 CrossRef CAS PubMed.
- P. V. AshaRani, M. G. Low Kah, M. P. Hande and S. Valiyaveettil, ACS Nano, 2009, 3, 279–290 CrossRef CAS PubMed.
- C. M. Sayes, J. D. Fortner, W. Guo, D. Lyon, A. M. Boyd, K. D. Ausman, Y. J. Tao, B. Sitharaman, L. J. Wilson, J. B. Hughes, J. L. West and V. L. Colvin, Nano Lett., 2004, 4(20), 1881–1887 CrossRef CAS.
- Y. T. Lim, S. Kim, A. Nakayama, N. E. Stott, M. G. Bawendi and J. V. Frangioni, Mol. Imaging, 2003, 2(1), 50–64 CrossRef CAS.
- J. P. Ryman-Rasmussen, J. E. Riviere and N. A. Monteiro-Riviere, Toxicol. Sci., 2006, 91(1), 159–165 CrossRef CAS PubMed.
- J. Lovric, S. J. Cho, F. M. Winnlk and D. Maysinger, Chem. Biol., 2005, 12, 1227–1234 CrossRef CAS PubMed.
- W. Lin, Y. Huang, X. D. Zhou and Y. Ma, Int. J. Toxicol., 2006, 25, 451–457 CrossRef CAS PubMed.
- M. Premanathan, K. Karthikeyan, K. Jeyasubramanian and G. Manivannan, Nanomed.: Nanotechnol., Biol. Med., 2011, 7, 184–192 CrossRef CAS PubMed.
|
This journal is © The Royal Society of Chemistry 2014 |
Click here to see how this site uses Cookies. View our privacy policy here.