DOI:
10.1039/C3AN01736B
(Paper)
Analyst, 2014,
139, 285-289
Selective and sensitive detection of acetylcholinesterase activity using denatured protein-protected gold nanoclusters as a label-free probe†
Received
11th September 2013
, Accepted 17th October 2013
First published on 18th October 2013
Abstract
Based on the fluorescence quenching of novel denatured protein-protected gold nanoclusters, a label-free detection method of acetylcholinesterase (AChE) activity has been developed. Using denatured bovine serum albumin (dBSA), in which 35 cysteine residues can interact polyvalently with Au nanoclusters (AuNCs) as a stabilizing agent, water-soluble and stable fluorescent gold nanoclusters were synthesized. The fluorescence of the AuNCs was quenched by thiocholine that was produced from the AChE hydrolysis of S-acetylthiocholine iodide (ACTI) to detect the AChE activity. The linear range of the method was 0.005–0.15 U mL−1. The limit of detection (LOD) was 0.02 mU mL−1. Other enzymes and metal ions, i.e., GPT, γ-GT, GOx, K+, Ca2+ and Na+, showed minimal interference. Using the fluorescence probe, satisfactory results for the detection of the AChE activity in human serum were obtained.
1. Introduction
Acetylcholinesterase (AChE: E.C.3.1.1.7) catalyzes the hydrolysis of acetylcholine (ACh), a neurotransmitter substance which functions in certain parts of the nervous system. ACh plays an important role in the physiological activity.1,2 A low level of acetylcholine is highly associated with Alzheimer's disease (AD), since the hydrolysis of acetylcholine catalyzed by acetylcholinesterase (AChE) can promote the aggregation of amyloid-β-peptide which plays a crucial role in the development of AD.3 In other words, AChE shows a close relationship with cholinergic dysfunction, as well as Parkinson's and Alzheimer's diseases.1 Based on the above situations, it is essential for real-time monitoring of the level of activity of AChE to guide the AD therapies.4 A number of traditional methods, e.g., colorimetric Ellmann method, and detection of hydrogen peroxide produced by oxidation of choline, have been employed for the quantitative and qualitative determination of AChE acitivity.5–8 However, low sensitivity, false-positive effects, and the techniques containing multi-steps organic reactions have limited the application of these methods. To improve the performance of the AChE activity determination method, some novel materials have been applied.4,9 Nevertheless, these methods also demand labeling and multi-step operation which are both labor-intensive and time-consuming.
Noble metal nanoclusters (NCs) typically consist of a few to several hundred metal atoms and their sizes are smaller than 2 nm. Compared to semiconductor quantum dots (QDs) and organic dyes, NCs are of special interest as their ultrafine size, non-toxicity, high fluorescence and good biocompatibility mean that they have been used in biological detections, e.g., bioimaging, biosensing, specific protein detection etc.10–14 For the determination of AChE activity, several highly sensitive NCs methods, e.g., fluorometric assay based on DNA-templated copper/silver nanoclusters15 and DNA-templated silver nanoclusters for fluorescence turn-on assay,16 in addition to continuous colorimetric assay with gold nanoparticles, have been proposed.4 Among noble metal NCs, Au clusters have been extensively studied because of their intrinsic characteristics such as ease of preparation and chemical stability.17
The synthesis of metal nanoclusters including AuNCs can be divided into two categories: the template-based synthesis such as using poly(amidoamine) dendrimers and DNA as a size-confining template, and monolayer-protected nanocluster synthesis such as protection by glutathione, tiopronin, meso-2,3-dimercaptosuccunic acid, phenylethylthiolate, dodecanethiol, and mercaptoundecanol.18 Compared with template-based synthesis NCs, monolayer-protected nanoclusters contain large Stokes's shifts, and even tunable fluorescence via the selection of appropriate length thiolated ligands.19 In 2009, a simple and green synthetic method of using native bovine serum albumin (BSA) as a stabilizing agent and a reductant to prepare AuNCs was proposed. The BSA-protected AuNCs exhibit photo-stable, non-toxic, and biocompatible features.20 Besides BSA, other proteins, e.g., trypsin, transferrin, insulin, ovalbumin, and papain, have also been used to prepare NCs.21–25
However, there are 34 cysteine residues in the formation of disulfide bonds and only 1 free cysteine in BSA. If the 35 cysteine residues could be completely liberated, they could act as polyvalent ligands for passivating the surface of metallic materials.26 Inspired by this thinking, denatured BSA (dBSA)-protected Ag NCs have been synthesized.17 The dBSA-protected Ag NCs exhibit higher stability vs. BSA-protected Ag NCs.
Here we synthesized water-soluble, fluorescent dBSA-protected AuNCs and used the AuNCs as a label-free fluorescent sensor for the sensitive and selective detection of AChE activity in human serum (Fig. 1 and Fig. 2). S-Acetylthiocholine iodide (ACTI) was used as the substrate. AChE hydrolyzed ACTI to give thiocholine whose sulfhydryl group can combine with the dBSA–AuNCs leading to fluorescence quenching of the NCs. The method is label-free, selective and sensitive.
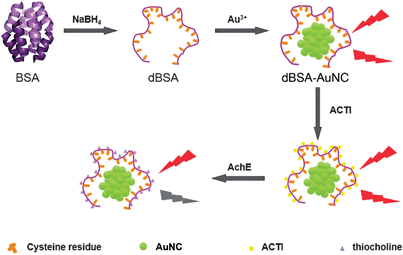 |
| Fig. 1 Schematic of the dBSA-directed synthesis of fluorescent Au clusters and the detection of AChE activity. | |
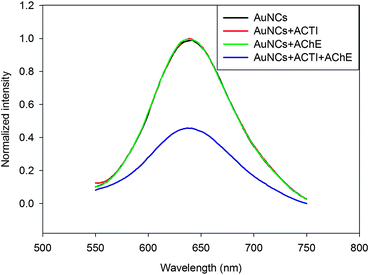 |
| Fig. 2 Fluorescence quenching of dBSA–AuNCs with different substrates: dBSA–AuNCs only (black), dBSA–AuNCs with 3 mM ACTI (red), dBSA–AuNCs with 0.4 U mL−1 AChE (green), dBSA–AuNCs with both 3 mM ACTI and 0.4 U mL−1 AChE (blue). | |
2. Experimental
2.1 Materials and reagents
Lyophilized bovine serum albumin (BSA), N-ethylmaleimide (NEM) acetylcholinesterase (AChE), and S-acetylthiocholine iodide (ACTI) were purchased from Sigma-Aldrich Chemical Co. (St. Louis, MO, USA). Sodium borohydride (NaBH4), tetrachloroauric acid tetrahydrate (HAuCl4·4H2O), and sodium hydroxide (NaOH) were purchased from Sinopharm Chemical Reagent Co., Ltd. (Beijing, China). Water (>18.2 MΩ) used for the experiments was purified using a Milli-Q System. Human serum samples derived from volunteers in Hunan normal university (all experiments were performed in compliance with the relevant laws and institutional guidelines).
2.2 Apparatus
Fluorescence emission spectra were recorded using an F-4500 fluorescence spectrometer (Hitachi, Japan). Absorption spectra were recorded using a UV 2450 UV-Vis spectrophotometer (Shimadzu, Japan). UV light excitation was at 365 nm. The morphology of the AuNCs was obtained by TEM.
2.3 Preparation of dBSA-templated gold nanoclusters
The dBSA was prepared according to the literature reports.27,28 The steps were as follows: 1 g of BSA was dissolved in 25 mL of deionized water, then 30 mg of sodium borohydride was added under stirring, the reaction was kept at room temperature for 1 h, and was then maintained at 70 °C until no more gas (H2) was generated. Whereafter, 9 mL of 24.3 mM HAuCl4 was added under stirring, and 2 min later, 4 mL of 1 M NaOH was added. The reaction was kept for 12 h at room temperature. After completing the reaction, the solution was separated by dialysis to remove the residual ions (i.e., Na+, Au3+, and OH−). The color of the solution changed from light yellow to deep brown. Finally, the solution was diluted with deionized water to 100 mL.
2.4 The linear curves of the fluorescence intensity of dBSA–AuNCs vs. the enzyme activity of AChE
The reaction solution contained 100 μL of diluted AuNCs and 100 μL of 3 mM ACTI, and was kept at 37 °C in a water bath for 10 min; then, 20 μL of various concentrations AChE were added to the reaction solution at 37 °C and reacting for 30 min. The change of fluorescence intensity of the solution was recorded using the F-4500 spectrometer.
2.5 The specificity of AChE detection by dBSA-protected gold nanoclusters
The reaction solution contained 100 μL of diluted AuNCs and 100 μL of 3 mM ACTI, and was kept at 37 °C in a water bath for 10 min; then, 20 μL of different enzymes, thiol-containing compounds and metal ions (AChE, GPT (glutamic-pyruvic transaminase), γ-GT (γ-glutamyltransferase), GOx, L-cysteine, homocysteine, GSH (glutathione), K+, Ca2+, and Na+) were added to the reaction solution at 37 °C and allowed to react for 30 min. The change of fluorescence intensity of reaction solution was recorded using the F-4500 spectrometer.
2.6 Detection of AChE activity in serum
The reaction solution contained 100 μL of diluted AuNCs and 100 μL of 3 mM ACTI, it was kept at 37 °C in a water bath for 10 min, and then 20 μL of human serum in 0.1 M PBS buffer (pH = 8.0) was added, and the reaction was kept at 37 °C for 30 min. The change of the fluorescence intensity reaction solution was recorded using the F-4500 spectrometer.
3. Results and discussion
3.1 Preparation and characterization of dBSA-coated gold nanoclusters
BSA has been widely used in the synthesis of gold nanoclusters. It consists of 35 thiol groups including 17 disulfide bonds and 1 free group. After reduction, 35 thiol groups could be free, which then could act as chelating groups for entrapping metal ions (e.g., Au3+) and as polyvalent groups for covalence-crosslinking with metallic nanomaterials (e.g., Au clusters). In this work, the denatured BSA was prepared by reducing the native BSA with sodium borohydride; then, the Tyr residues with phenolic groups in the BSA would reduce Au3+ to luminescent AuNCs at physiological temperature.29 The insert in Fig. 3 shows that the formed AuNC solutions were deep brown in color under visible light (left), whereas they emitted red fluorescence under UV light with 365 nm (right). Fluorescent AuNCs with red or near-infrared emissions that offered improved tissue penetration depth and reduced background fluorescence would be effective for cellular biosensing and bioimaging in further work. From the optical absorption and photoemission spectra of these as-prepared AuNCs (Fig. 3), it could be concluded that no apparent surface plasmon resonance absorption peak in the range between 400 and 600 nm could be observed to indicate the existence of small Au nanoparticles. While the emission band of dBSA-capped AuNCs was 640 nm with excitation at 520 nm, the fluorescence quantum yield of the Au clusters was ∼6.5%, calculated with Rhodamine B as a reference according to the method of Williams et al.30 To further confirm the formation of the dBSA-coated AuNCs, transmission electron microscopic images were obtained. As shown in Fig. S1,† the as-prepared fluorescent AuNCs were mostly spherical dots with high monodispersity. The size is uniform, averaging about 1.5 nm in diameter.
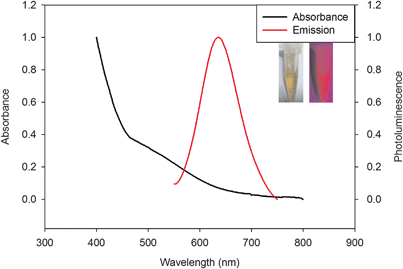 |
| Fig. 3 UV-Vis absorption (black line), fluorescence emission (red line) spectra of the as-prepared AuNCs. The excitation wavelength was 520 nm. Insert: the photographs of the aqueous solution of AuNCs under visible (left) and UV light (right) with excitation at 365 nm. | |
For application in biosensing and bioimaging, one of the primary requirements of NCs is water-solubility and stability under high ionic strength environments. Whereas protein-stabilized fluorescent metal clusters are sufficiently water-soluble, their stability in high ionic strength environments is still a challenge. The normalized fluorescent spectra of the dBSA-coated Au clusters in the absence and presence of various ionic strengths of PBS buffer are shown in Fig. S2D,† showing that the photoluminescence intensity was hardly changed. Under further investigation, such as variation of temperature, storage time, as well as pH, the PL intensity was not obviously changed (Fig. S2A–C†). The stability of the dBSA–AuNCs was enhanced by the polyvalent interactions between the ligand and the metal core.
3.2 Sensitivity of sensor
Under the optimum conditions (Fig. S3†), we used the fluorescent materials to detect the enzyme activity of AChE, which would catalyze the hydrolysis of ACTI and then produce thiocholine that could quench the fluorescence of AuNCs (Fig. 1 and 2). With the increase in enzyme activity of the AChE, the fluorescence of the AuNCs could decrease. As depicted in Fig. S4† and Fig. 4, the linear range of the sensor was 0.005–0.15 U mL−1 and the corresponding linear function was y = −2.9231x + 0.5729 with a correlation coefficient of R2 = 0.9276. The limit of detection (LOD) was 0.02 mU mL−1 (S/N = 3), which was lower than that of previous methods, and detailed comparison is shown in Table S1.† However, with higher enzyme activity, the quenching curve tended towards being flattened. This tendency is consistent with the kinetics of enzyme-catalyzed reactions.31,32
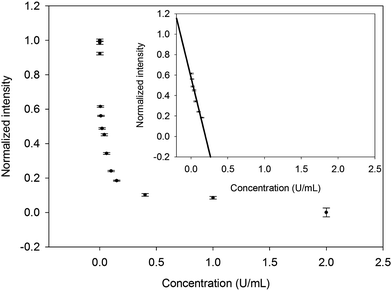 |
| Fig. 4 The fluorescence intensity of dBSA–AuNCs in the presence of various enzyme activities (0–2.0 U mL−1). Inset: the linear relationship of the quenching efficiency of dBSA–AuNCs vs. the enzyme activity of AChE (0.005–0.15 U mL−1). The error bars represent the standard deviation of three independent measurements. | |
3.3 Specificity of sensor
To evaluate the novel fluorescence sensor for the detection of AChE activity, a series of relevant enzymes, thiol-containing compounds and metal ions (AChE, GPT, γ-GT, GOx; L-cysteine, homocysteine, GSH; K+, Ca2+, and Na+) were studied as a control. As demonstrated in Fig. 5 (black bars), except for GPT, γ-GT, GOx, K+, Ca2+, and Na+, obvious fluorescence quenching was obtained with different sensitivity toward AChE and thiol-containing compounds. AChE was the most efficient in quenching the fluorescence intensity of the dBSA–Au clusters. However, in order to improve the accuracy of the fluorescent probe, interferences of thiol-containing compounds could be eliminated by using thiol-blocking reagents, such as N-ethylmaleimide (NEM). As shown in Fig. 5 (gray bars), thiol-containing compounds showed minimal interference by using NEM compared to the control without NEM. In brief, the fluorescence probe could be used for the selective and sensitive detection of AChE activity.
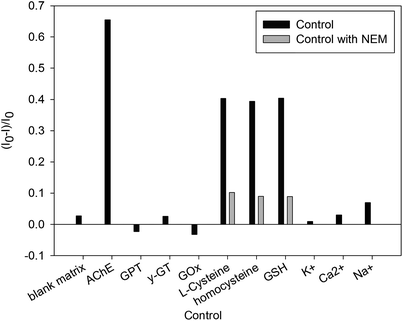 |
| Fig. 5 Fluorescence responses of dBSA–AuNCs to different enzymes, thiol-containing compounds and ions. AChE, GPT, γ-GT, and GOx were 0.4 U mL−1; L-cysteine, homocysteine, GSH, K+, Ca2+, and Na+ were 10 nM. The gray bars and black bars represent the control samples with and without NEM, respectively. All measurements were performed in PBS, pH = 8.0, thermostatted at 37 °C for 30 min. I0 and I are the PL intensities of the dBSA–AuNCs in the absence and presence of analytes, respectively. | |
3.4 Detection of AChE activity in human serum
The selective and sensitive sensor could be directly applied for detecting AChE activity in real samples. We further investigated the practicality of the development of the label-free sensor by detecting AChE activity in human serum samples. The enzyme activity of AChE in human serum samples detected by dBSA–AuNCs is shown in the Fig. 6. The results showed almost no difference between the quenched fluorescence intensity induced by the diluted serum samples with and without NEM, which indicated that any thiols in the diluted serum samples did not affect the detection of AChE activity. Therefore, no sample pretreatment of human serum was used except for appropriate dilution. So the specificity of the developed biosensor might be a most possible candidate to detect the enzyme activity of AChE in human serum without any pretreatment.
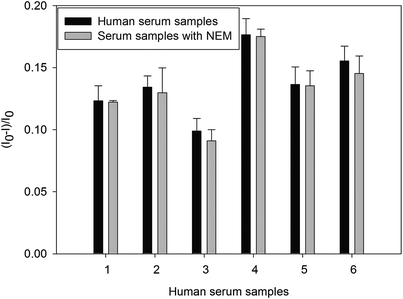 |
| Fig. 6 Quenched fluorescence intensity of the dBSA–AuNCs by dilute human serum samples before (black bars) and after (gray bars) pre-treatment with NEM. All measurement were performed in 100 mM PBS, pH = 8.0, thermostatted at 37 °C for 30 min. The error bars represent the standard deviation of three independent measurements. | |
4. Conclusion
In summary, we reported a simple, ‘green’ synthetic route to prepare dBSA-protected AuNCs. The as-prepared AuNCs were satisfactorily water-soluble and stable under high ionic strength environments. The dBSA with its 35 cysteine residues could act as a polyvalent ligand to stabilize the metal core and lead to the formation of stable AuNCs in aqueous solutions. The dBSA-coated Au clusters were further used as a label-free sensor for the sensitive and selective detection of AChE activity in human serum. The strategy could be described as their fluorescence being quenched by thiocholine that was produced from the acetylcholinesterase (AChE) hydrolysis of S-acetylthiocholine iodide (ACTI). Importantly, the method was simple to apply because it did not require complicated modifications and expensive instrumentation. Moreover, the as-prepared fluorescent AuNCs with red emission could improve the tissue penetration depth and reduce background fluorescence, which presage more opportunities for the study of biological systems in future applications.
Acknowledgements
This work was financially supported by the National Natural Science Foundation of China (21205037, 21275049, 21275052), the aid program for S&T innovation research team in higher education institutions and the construct program of the key disciplines of Hunan province. Project supported by the foundation for innovative research groups of the Hunan Natural Science Foundation of China.
References
- W. Xue and T. H. Cui, J. Nano Res., 2008, 1, 1–9 CAS.
- P. J. Whitehouse, D. L. Price, R. G. Struble, A. W. Clark, J. T. Coyle and M. R. Delon, Science, 1982, 215, 1237–1239 CAS.
- D. R. Liston, J. A. Nielsen, A. Villalobos, D. Chapin, S. B. Jones, S. T. Hubbard, I. A. Shalaby, A. Ramirez, D. Nason and W. F. White, Eur. J. Pharmacol., 2004, 486, 9–17 CrossRef CAS PubMed.
- M. Wang, X. Gu, G. Zhang, D. Zhang and D. Zhu, Langmuir, 2009, 25, 2504–2507 CrossRef CAS PubMed.
- G. L. Ellman, K. D. Courtney, V. Andres and R. M. Featherstone, Biochem. Pharmacol., 1961, 7, 88–90 CrossRef CAS.
- S. H. Hou, Z. M. Ou, Q. Chen and B. Y. Wu, Biosens. Bioelectron., 2012, 33, 44–49 CrossRef CAS PubMed.
- A. Riklin and I. Willner, Anal. Chem., 1995, 67, 4118–4126 CrossRef CAS.
- P. Valeri, Y. Xiao and W. Itamar, Nano Lett., 2005, 5, 649–653 CrossRef PubMed.
- S. Z. Liao, Y. N. Qiao, W. T. Han, Z. X. Xie, Z. Y. Wu, G. L. Shen and R. Q. Yu, Anal. Chem., 2012, 84, 45–49 CrossRef CAS PubMed.
- R. L. Whetten, J. T. Khoury, M. M. Alvarez, S. Murthy, I. Vezmar, Z. L. Wang, P. W. Stephens, C. L. Cleveland, W. D. Luedtke and U. Landman, Adv. Mater., 1996, 8, 428–433 CrossRef CAS.
- P. D. Jadzinsky, G. Calero, C. J. Ackerson, D. A. Bushnell and R. D. Kornberg, Science, 2007, 318, 430–433 CrossRef CAS PubMed.
- J. H. Yu, S. Choi and R. M. Dickson, Angew. Chem., Int. Ed., 2009, 48, 318–320 CrossRef CAS PubMed.
- W. W. Guo, J. P. Yuan, Q. Z. Dong and E. K. J. Wang, J. Am. Chem. Soc., 2010, 132, 932–934 CrossRef CAS PubMed.
- Y. Lei, F. Mehmood, S. Lee, J. Greeley, B. Lee, S. Seifert, R. E. Winans, J. W. Elam, R. J. Meyer, P. C. Redfern, D. Teschner, R. Schlogl, M. J. Pellin, L. A. Curtiss and S. Vajda, Science, 2010, 328, 224–228 CrossRef CAS PubMed.
- W. H. Li, W. Li, Y. F. Hu, Y. L. Xia, Q. P. Shen, Z. Nie, Y. Huang and S. Z. Yao, Biosens. Bioelectron., 2013, 47, 345–349 CrossRef CAS PubMed.
- Y. D. Zhang, Y. N. Cai, Z. L. Qi, L. Lu and Y. X. Qian, Anal. Chem., 2013, 85, 8455–8461 CrossRef CAS PubMed.
- C. L. Guo and J. Irudayaraj, Anal. Chem., 2011, 83, 2883–2889 CrossRef CAS PubMed.
- C. A. Lin, C. H. Lee, J. T. Hsieh, H. H. Wang, J. K. Li, J. L. Shen, W. H. Chan, H. I. Yeh and W. H. Chang, J. Med. Biol. Eng., 2009, 29, 276–283 Search PubMed.
- C. C. Huang, Z. Yang, K. H. Lee and H. T. Chang, Angew. Chem., Int. Ed., 2007, 46, 6824–6828 CrossRef CAS PubMed.
- J. P. Xie, Y. G. Zheng and J. Y. Ying, J. Am. Chem. Soc., 2009, 131, 888–889 CrossRef CAS PubMed.
- J. M. Liu, J. T. Chen and X. P. Yan, Anal. Chem., 2013, 85, 3238–3245 CrossRef CAS PubMed.
- Y. Wang, J. T. Chen and X. P. Yan, Anal. Chem., 2013, 85, 2529–2535 CrossRef CAS PubMed.
- C. L. Liu, H. T. Wu, Y. H. Hsiao, C. W. Lai, C. W. Shih, Y. K. Peng, K. C. Tang, H. W. Chang, Y. C. Chien, J. K. Hsiao, J. T. Cheng and P. T. Chou, Angew. Chem., Int. Ed., 2011, 50, 7056–7060 CrossRef CAS PubMed.
- J. Qiao, X. Y. Mu, L. Qi, J. J. Deng and L. Q. Mao, Chem. Commun., 2013, 49, 8030–8032 RSC.
- Y. Chen, Y. Wang, C. X. Wang, W. Y. Li, H. P. Zhou, H. P. Jiao, Q. Lin and C. Yu, J. Colloid Interface Sci., 2013, 396, 63–68 CrossRef CAS PubMed.
- K. Hirayama, S. Akashi, M. Furuya and K. Fukuhara, Biochem. Biophys. Res. Commun., 1990, 173, 639–646 CrossRef CAS.
- Q. Wang, Y. Kuo, Y. Wang, G. Shin, C. Ruengruglikit and Q. Huang, J. Phys. Chem. B, 2006, 110, 16860–16866 CrossRef CAS PubMed.
- J. H. Wang, H. Q. Wang, H. L. Zhang, X. Q. Li, X. F. Hua and Y. D. Zhao, Anal. Bioanal. Chem., 2007, 388, 969–974 CrossRef CAS PubMed.
- J. Xie, J. Y. Lee, D. I. C. Wang and Y. P. Ting, ACS Nano, 2007, 1, 429–439 CrossRef CAS PubMed.
- A. T. R. Williams, S. A. Winfield and J. N. Miller, Analyst, 1983, 108, 1067–1071 RSC.
- L. Cao, J. Ye, L. Tong and B. Tang, Chem.–Eur. J., 2008, 14, 9633–9640 CrossRef CAS PubMed.
-
A. G. Marangoni, Enzyme Kinetics: A Modern Approach, John Wiley & Sons, New Jersey, USA, 2003 Search PubMed.
Footnotes |
† Electronic supplementary information (ESI) available. See DOI: 10.1039/c3an01736b |
‡ Contributed equally to this work. |
|
This journal is © The Royal Society of Chemistry 2014 |
Click here to see how this site uses Cookies. View our privacy policy here.