DOI:
10.1039/C2SC21300A
(Edge Article)
Chem. Sci., 2013,
4, 965-969
Tuning the catalytic activity of L-proline functionalized hydrophobic nanogel particles in water†
Received 17th August 2012, Accepted 2nd November 2012
First published on 20th November 2012
Abstract
L-Proline functionalized PMMA nanogels with a range of catalyst functionalization (0.5–15 wt%) and cross-linking densities (0–50 wt%) were prepared via emulsion polymerization. The catalyst efficiency in water was investigated using a model asymmetric aldol reaction and an unprecedented reduction in catalyst loading, whilst maintaining high catalytic activity, is reported. In these reactions, a marked effect on selectivity was observed and determined to be dependent on the hydrophobicity of the nanogel particles. Furthermore, the effect of increasing cross-linking density on the catalytic efficiency of these particles (and their core–shell analogues) was explored and a significant reduction in activity was observed.
Introduction
It is well known that L-proline may be used to effectively catalyze a range of asymmetric organic reactions, yielding products with high stereoselectivity and enantiomeric excess.1–5 However, although its efficiency in organic solvents has been well documented, there have only been a few examples of its use in water due to the inability of water to dissolve the organic reagents, which are often hydrophobic.6–8 In cases where the catalyst was modified so as to be more hydrophobic, a concentrated organic microphase was formed around the catalytic site by the reagents, resulting in an observed acceleration in rate of reaction. This was proposed to be driven by the hydrophobic effect where the surrounding water drives the reagents towards the formed organic phase to lower the interfacial energy of the system.9,10Subsequently, nanoreactor systems based on synthetic polymers have been reported to successfully create a favourable nanoenvironment able to concentrate the reagent phase for efficient catalysis.11 For example, Fréchet and Hawker showed the ability of dendrimeric star polymers to attract reagents based on polarity, calling this the ‘concentrator effect’.12 Similarly, diblock copolymers that consist of a hydrophobic and a hydrophilic block have also been extensively studied for their use in catalytic systems due to their ability to self-assemble in water, providing a protected hydrophobic environment for the supported catalyst.13 Previous studies have incorporated the catalyst in the hydrophobic compartment of a micellar assembly by copolymerizing the monomeric derivative with a hydrophobic co-monomer. Recently our group reported the use of such polymeric nanoreactor systems containing 4-dimethylaminopyridine14 or L-proline15 that efficiently carried out organic reactions in water. The observed increase in reaction rate was proposed to be due to the ability of the hydrophobic core to effectively sequester the organic reagents from the surrounding water. Although such systems have proven to be highly efficient, the multi-step synthesis of the micelles significantly reduces their potential for use in industrial scale applications.
Related to these systems, microgel particles are excellent candidates for use as catalytic systems because they are unimolecular polymeric nano-sized entities that retain their form under a range of conditions (i.e. changes in temperature, solvent or concentration).16 In addition, their synthesis is scalable and similar procedures are already being performed in industry for the mass production of paints etc.17–19 Previous reports have also shown that microgel particles can be used as catalyst supports by loading metal nanoparticles within the polymeric matrix.20,21 Other applications involving sub-micron sized cross-linked polymer particles can be found in fields such as drug delivery,22,23 biotechnology,24 membranes25 and cosmetics,26 as well as biomedical diagnostics (theranostics).27
Although the incorporation of L-proline within micron-sized polymer beads (∼100 μm) has been reported by Hansen et al.28 the effect of parameters such as the degree of catalyst functionalization within the particles or particle cross-linking density on the catalysis activity have not yet been investigated in detail. We aim to synthesize nano-sized cross-linked particles with a confined hydrophobic core and fully explore their potential as nanoreactors. Minimization of the particle size should enhance the reaction rate due to the increased surface area that enables faster diffusion of the reagents into the nanoparticle core. By tethering the catalyst within the hydrophobic core it ultimately brings the catalyst and reagents into close proximity with one another, concentrating both moieties within the same reaction nano-space. Fréchet et al. reported the synthesis of poly(styrene) nanogels where increasing the cross-linking density (CLD) caused a marked decrease in access to an azide-decorated core for large alkyne functionalized PEG units due to steric hindrance: small molecules were successfully incorporated into the nanogel core regardless of the CLD.29 We aim to exploit the hydrophobic–hydrophilic balance of the nanogels by tailoring the CLD and thus controlling the access of small molecules into the catalyst enriched core. Furthermore, by varying the degree of catalyst functionalization (DoF, where DoF refers to the weight% of L-proline methacrylate incorporated in the nanogel), we aim to highlight the importance of the local environment within the nanoreactor core and how this can enable the catalytic activity and selectivity to be tuned.
Results and discussion
Nanogel synthesis
A range of functional cross-linked poly(methyl methacrylate) (PMMA) nanogel particles (Fig. 1) were prepared with different CLDs (0.5–10 wt%) and DoFs (2–15 wt%). A schematic representation of this process is presented in Scheme S1.† Particles were readily synthesized in the 20–50 nm size range, with low size polydispersity (Table 1 and Fig. S2†). The small size of the nanogels was attributed to the intended high concentration of surfactant (sodium dodecyl sulfate (SDS), 25 wt% with respect to the monomer) in the emulsion system. The size was determined by dynamic light scattering (DLS) (Fig. S2 and S3†) and confirmed by dry state transmission electron microscopy (TEM, Fig. 1). In order to better understand the structure of the nanogel particles, especially the positioning of the proline units, the emulsion polymerization of ProMA and MMA in water was studied in the absence of a cross-linker (Fig. S1†). Despite ProMA polymerizing slightly faster than MMA, the difference was not thought to be significant enough to promote formation of a core–shell structure, with L-proline moieties almost exclusively in the shell, rather that ProMA can be considered to be evenly distributed within the hydrophobic nanogel core.30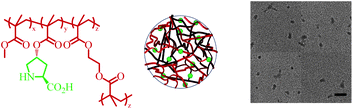 |
| Fig. 1 Structural representation of L-proline functionalized cross-linked PMMA nanogel and unstained TEM micrograph (10 wt% CLD, 2 wt% DoF), and Dav = 25 ± 4 nm (scale bar = 100 nm). | |
Table 1 Table showing the particle size (in nm, with PD in parenthesis) with a range of DoFs (wt%) and CLDs (wt%), as determined by DLS.
DoF (%)/CLD (%) | 0.5 | 2 | 5 | 10 |
---|
2 | 23 (0.192) | 32 (0.080) | 38 (0.093) | 27 (0.095) |
5 | 28 (0.232) | 35 (0.142) | 36 (0.162) | 21 (0.111) |
9 | 42 (0.128) | 36 (0.109) | 33 (0.148) | 41 (0.081) |
15 | 49 (0.252) | 48 (0.101) | 42 (0.167) | 38 (0.250) |
Catalysis
The aldol reaction of 4-nitrobenzaldehyde and cyclohexanone was used as a model reaction, as it has been widely used in the literature for a range of supported L-proline catalyst systems.31 The reaction was catalyzed by each of the functionalized PMMA nanogels at 1 mol% catalyst loading. As the nanogels are functionalized with the catalyst to varying degrees, catalysis with different amounts of nanogel particles (i.e. different number of hydrophobic nanoreactors), diluted to the same volume was required, keeping the reagent concentration and ratio to L-proline constant (Table S1†). It was found that, after 24 hours the reaction catalyzed by nanogels with high DoF is less efficient than low DoF (Table 2), which can be attributed to the lower number of hydrophobic nanoreactors present in these reactions.
Table 2 Table showing conversion (%) in the aldol reaction after 24 hours catalyzed by the functionalized nanogels, at 1 mol% catalyst loading and room temperature in water.

|
---|
DoF (%)/CLD (%) | 0.5 | 2 | 5 | 10 |
---|
2 | 73 | 69 | 75 | 49 |
5 | 32 | 25 | 33 | 33 |
9 | 28 | 28 | 31 | 44 |
15 | 18 | 40 | 51 | 37 |
Effect of DoF
At 0.5 wt% CLD, a general trend in catalytic efficiency was observed: nanogels with low DoF were found to be more efficient than those with high DoF (Fig. 2A). This trend was observed throughout the range of CLDs (Fig. S4†), though less prominent at high CLDs.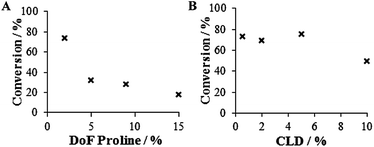 |
| Fig. 2 Aldol reaction catalyzed by L-proline functionalized PMMA nanogels at 1 mol% catalyst loading and room temperature, in water, (A) CLD 0.5 wt% and DoF 2–15 wt%, (B) DoF 2 wt% and CLDs 0.5–10 wt%. | |
The trends observed in catalytic activity may be due to differences in the number of distinct catalytic nanoreactors or hydrophobic pockets present in each reaction, as more nanogels with low DoF are required to make up the desired catalyst loading (1 mol%) (Table S1†). Although the reactions do not reach completion within 24 hours, selectivity comparable to unsupported L-proline in organic solvents is achieved. However, in terms of activity, the nanogel system reached conversions comparable to unsupported catalysis in organic solvents using 5 to 10 times less catalyst (Tables 2 and 3), highlighting the efficiency of our hydrophobic L-proline functionalized system in water.31 The smaller particle size does not seem to show the anticipated effect as comparable yields and selectivity were shown in Hansen et al.'s work with a larger and different (micron-sized) proline-bearing polymeric system with 2% CLD.28
Table 3 Aldol reaction catalyzed by PMMA nanogels with 0.5 wt% CLD and DoFs of 2–15 wt%.
DoF/% | Conv.a/% | Anti/syn ratioa | eeb/% |
---|
Determined by 1H NMR spectroscopy, in CDCl3. Determined by HPLC, Chiralpak IA, hexane/IPA (90/10), 1 mL min−1. |
---|
0.5 | 28 | 98/2 | 97 |
2 | 73 | 97/3 | 99 |
5 | 32 | 98/2 | 89 |
9 | 28 | 99/1 | 86 |
15 | 18 | 95/5 | 81 |
Effect of CLD
Changes to the CLD in the 0.5–10 wt% range were found to have less of an effect on the catalyst activity than changes to the DoF (see Fig. 2B and S5†). The selectivity was also unaffected by changes to the CLD in this range (Table 4) and furthermore, leaving the reaction for an additional 2 days resulted in near completion for all systems. In order to investigate the CLD limit, nanogel particles with 25 wt% CLD were synthesized (DoF 2 wt%, 22 nm, PD 0.234). However, the catalyst activity was not completely shut down and the reaction at 1 mol% still reached 31% conversion after 24 hours. Further increasing the CLD to 50 wt% (DoF 2 wt%, 20 nm, PD 0.108) did cause the expected and dramatic drop in conversion to 14% after 24 hours. We hypothesized that, due to the somewhat hydrophilic nature of the catalyst, the particles remain active even at high CLD due to the presence of L-proline moieties close to the water interface of the nanogels. To test this rationale, a PtBuMA cross-linked shell was grown using the PMMA nanogels as seeds for the emulsion polymerization (CLD 50 wt%, DoF 2 wt%, 23 nm, PD 0.119, DLS in Fig. S6†), the idea being to render any surface catalyst moieties difficult for the reagents to access. As anticipated, the activity of the double-hydrophobic nanogel was almost eliminated and less than 5% conversion after 24 hours was observed. The influence of CLD was further emphasized when particles without cross-linking (CLD 0 wt%, DoF 2 wt%, 24 nm, PD 0.152) showed low catalytic efficiency (41% conversion) deviating from the observed trend. Although slightly difficult to rationalize, one possible explanation for this observation is that in absence of a cross-linker, the individual polymer chains are unable to form confined hydrophobic pockets that efficiently concentrate reagents and cannot allow such easy diffusion of reagents in and out from the catalytic moieties.
Table 4 Activity and selectivity of the aldol reaction catalyzed by 1 mol% PMMA nanogels with 2 wt% DoF and a range of CLDs.
CLD/% | Conv.a/% | Anti/syn ratioa | eeb/% |
---|
Determined by 1H NMR spectroscopy, in CDCl3. Determined by HPLC, Chiralpak IA, hexane/IPA (90/10), 1 mL min−1. PMMA nanogel with PtBuMA shell. |
---|
0 | 41 | 98/2 | 94 |
0.5 | 73 | 97/3 | 99 |
2 | 69 | 95/5 | 98 |
5 | 75 | 97/3 | 86 |
10 | 49 | 96/4 | 95 |
25 | 31 | 97/3 | 95 |
50 | 14 | 95/5 | 95 |
50c | <5 | — | — |
Effect of catalyst concentration/loading
To examine a series in which the number of reactors in the catalysis reactions remained constant (previously carried out at 1 mol% catalyst, leading to variations in the amount of polymeric material present), the same volume of nanogels (i.e. same amount of polymeric material, variable amounts of catalyst: 1–8.5 mol%) was used to catalyze the reaction (Table 5). The catalyst efficiency in this case is represented by turnover numbers (TON)32 and once again results suggest that particles with low DoF catalyze the reaction more efficiently (2 wt% DoF is almost 4 times more efficient than 5 wt% DoF with same CLD, Fig. S7†). The importance of a confined space where the reagents are able to efficiently interact with the chiral catalyst in a chiral space has been studied by Raja et al.33 using inorganic supports. We rationalize that in our case, having a greater number of isolated catalytic sites within the particles allows for efficient formation of the undisrupted transition states responsible for the high activity and enantioselectivity observed in L-proline catalyzed reactions.34,35 Further lowering the catalyst DoF to 0.5 wt% (CLD 0.5 wt%, 25 nm, PD 0.091), and thus reducing the catalyst loading in the reaction to 0.15 mol% resulted in a great increase in TON to 187 (whilst maintaining excellent selectivity, anti/syn 98/2, ee 97%). This highlights the potential for effectively reducing the catalyst loading and maximizing product output in these nanogel systems. In addition, control experiments with unfunctionalized PMMA nanogels, which provided no conversion whatsoever, further highlighting the importance of having the catalyst tethered within the particles, bringing reaction components with different solubilities together within the same reaction sphere (Table S2†).
Table 5 Table showing the efficiency of nanogel particles with the same amount of reagents catalyzed by same number of particles (different catalyst loading) with 0.5 wt% CLD, particles with PMMA core.
DoF/% | mol% | Conv.a/% | Anti/syna | eeb/% | TON |
---|
Determined by 1H NMR spectroscopy, in CDCl3. Determined by HPLC, ChiralPak IA, hexane/IPA (90/10), 1 mL min−1. |
---|
2 | 1 | 73 | 97/3 | 99 | 73 |
5 | 3 | 51 | 97/3 | 88 | 17 |
9 | 5 | 57 | 97/3 | 81 | 11 |
15 | 8.5 | 53 | 98/2 | 67 | 6 |
Effect of nanogel hydrophobicity
The results presented so far are interesting, as they show a dramatic decrease in enantioselectivity with increasing L-proline DoF (Table 5). We hypothesize that this effect is related to the reduced hydrophobicity of the core as well as the formation of less isolated catalytic sites.To probe reaction dependence on nanogel core hydrophobicity, nanogels based on four more hydrophobic monomers were synthesized: ethyl, n-butyl, tert-butyl and lauryl methacrylate. Particle size of the new nanogels was found to be in a similar range to those previously reported for the PMMA nanogels (DLS, Fig. S8 and TEM, Fig. S9†) and analogous aldol reactions were carried out using the same number of nanogel particles (resulting in different catalyst loadings vide supra). The same trend in TON was observed for each, confirming the high efficiency of nanogel particles with lower catalyst DoF (Fig. S10†). However, a dramatic decrease in enantioselectivity with increasing DoF was not observed for any of these, more hydrophobic, nanogel systems (Table 6). We propose that the high enantioselectivity observed for more hydrophobic nanogels is a direct result of their ability to more efficiently sequester the reagents. The difference in core hydrophobicity was investigated by relative uptake of the hydrophobic dye Nile Red (Fig. S11†) and the efficient uptake of the reaction reagents was also demonstrated by an increase in particle size upon re-dispersing the nanogels in cyclohexanone after removal of water (Fig. S12†). The considerably lower catalytic activity achieved by the lauryl methacrylate-based nanogels is most likely a result of greater steric hindrance. On the other hand, the greater catalytic efficiency observed for the ethyl methacrylate based system compared to both the nbutyl and tbutyl methacrylate systems is attributed to the hydrophobic–hydrophilic balance within the nanogel core (Fig. S13†). Although at low DoF the activity and selectivity seem unaffected by the slight difference in steric bulk introduced by tbutyl compared to nbutyl side groups, at higher DoF a greater difference in activity is observed. These results suggest that the overall core hydrophobicity is an important factor in achieving high stereoselectivity in L-proline catalyzed aldol reactions.
Table 6 Table showing the catalytic efficiency of nanogel particles with 0.5 wt% CLD, a range of DoF and different cores, catalysis carried out using the same number of particles.
Core | DoF/% | mol% | Conv.a/% | Anti/syna | eeb/% | TON |
---|
Determined by 1H NMR spectroscopy, in CDCl3. Determined by HPLC, ChiralPak IA, hexane/IPA (90/10), 1 mL min−1. |
---|
EMA | 2 | 1 | 99 | 99/1 | 99 | 99 |
5 | 3 | 97 | 98/2 | 99 | 32 |
9 | 5 | 95 | 98/2 | 99 | 19 |
15 | 8.5 | 95 | 98/2 | 97 | 11 |
nBuMA | 2 | 1 | 78 | 99/1 | 99 | 78 |
5 | 3 | 63 | 99/1 | 99 | 21 |
9 | 5 | 79 | 97/3 | 99 | 16 |
15 | 8.5 | 78 | 98/2 | 99 | 9 |
tBuMA | 2 | 1 | 79 | 97/3 | 93 | 79 |
5 | 3 | 48 | 99/1 | 94 | 16 |
9 | 5 | 44 | 99/1 | 95 | 9 |
15 | 8.5 | 53 | 99/1 | 92 | 6 |
LMA | 2 | 1 | 16 | 95/5 | 99 | 16 |
5 | 3 | 19 | 96/4 | 98 | 6 |
9 | 5 | 13 | 95/5 | 97 | 3 |
15 | 8.5 | 14 | 98/2 | 89 | 2 |
Conclusions
In summary, the synthesis of a range of hydrophobic nanogels with embedded L-proline catalytic functionality via emulsion polymerization is reported. The catalytic efficiency with respect to modifications to cross-linking density and degree of catalyst functionalization is demonstrated. The importance of local core environment design is also highlighted by the enantioselectivity dependence on degree of functionalization and core hydrophobicity as shown by the use of different monomers. PMMA, being the least hydrophobic in the range, showed a decrease in enantioselectivity with increasing L-proline content and hence a proposed increase in water content within the core. However, the same effect was not observed when using more hydrophobic nanogels. The ability to completely shut down the catalytic activity via the preparation of double-hydrophobic core–shell nanogels was also demonstrated. Successful organocatalytic reactions were reported at loadings as low as 0.15 mol%, highlighting the efficiency of the functionalized nanogel system to catalyze organic reactions in water.Acknowledgements
Financial support from the EPSRC, Homerton College and the Universities of Warwick and Cambridge are gratefully acknowledged. Some of the equipment used in this research was obtained through Birmingham Science City with support from Advantage West Midlands and part funded by the European Regional Development Fund.Notes and references
- B. List, Tetrahedron, 2002, 58, 5573 CrossRef CAS.
- K. Sakthivel, W. Notz, T. Bui and C. F. Barbas III, J. Am. Chem. Soc., 2001, 123, 5260 CrossRef CAS.
- A. B. Northrup and D. W. C. MacMillan, J. Am. Chem. Soc., 2002, 124, 6798 CrossRef CAS.
- Z. Tang, F. Jiang, L.-T. Yu, X. Cui, L.-Z. Gong, A.-Q. Mi, Y.-Z. Jiang and Y.-D. Wu, J. Am. Chem. Soc., 2003, 125, 5262 CrossRef CAS.
- W. Notz, F. Tanaka and C. F. Barbas III, Acc. Chem. Res., 2004, 37, 580 CrossRef CAS.
- P. M. Pihko, K. M. Laurikainen, A. Usano, A. I. Nyberg and J. A. Kaavi, Tetrahedron, 2006, 62, 317 CrossRef CAS.
- H. Torii, M. Nakadai, K. Ishihara, S. Saito and H. Yamamoto, Angew. Chem., Int. Ed., 2004, 43, 1983 CrossRef CAS.
- S. Aratake, T. Itoh, T. Okano, N. Nagae, T. Sumiya, M. Shoji and Y. Hayashi, Chem.–Eur. J., 2007, 13, 10246 CrossRef CAS.
- M. Gruttadauria, F. Giacalone, A. Mossuto Marculescu, P. Lo Meo, S. Riela and R. Noto, Eur. J. Org. Chem., 2007, 4688 CrossRef CAS.
- N. Mase, Y. Nakai, N. Ohara, H. Yoda, K. Takabe, F. Tanaka and C. F. Barbas III, J. Am. Chem. Soc., 2005, 128, 734 CrossRef.
- Z. Ge, D. Xie, D. Chen, X. Jiang, Y. Zhang, H. Liu and S. Liu, Macromolecules, 2007, 40, 3538 CrossRef CAS.
- B. Helms, C. O. Liang, C. J. Hawker and J. M. J. Fréchet, Macromolecules, 2005, 38, 5411 CrossRef CAS.
- R. K. O'Reilly, C. J. Hawker and K. L. Wooley, Chem. Soc. Rev., 2006, 35, 1068 RSC.
- P. Cotanda, A. Lu, J. P. Patterson, N. Petzetakis and R. K. O'Reilly, Macromolecules, 2012, 45, 2377 CrossRef CAS.
- A. Lu, P. Cotanda, J. P. Patterson, D. A. Longbottom and R. K. O'Reilly, Chem. Commun., 2012, 48, 9699 RSC.
- B. R. Saunders, H. M. Crowther and B. Vincent, Macromolecules, 1997, 30, 482 CrossRef CAS.
- B. Szabo, in Polymer Dispersions and Their Industrial Applications, Wiley-VCH Verlag GmbH & Co. KGaA, 2003, p. 103 Search PubMed.
- B. Richey and M. Burch, in Polymer Dispersions and Their Industrial Applications, Wiley-VCH Verlag GmbH & Co. KGaA, 2003, p. 123 Search PubMed.
- S. Grandhee, in Polymer Dispersions and Their Industrial Applications, Wiley-VCH Verlag GmbH & Co. KGaA, 2003, p. 163 Search PubMed.
- A. Pich, S. Bhattacharya, Y. Lu, V. Boyko and H.-J. P. Adler, Langmuir, 2004, 20, 10706 CrossRef CAS.
- E. Pavlopoulou, G. Portale, K. E. Christodoulakis, M. Vamvakaki, W. Bras and S. H. Anastasiadis, Macromolecules, 2010, 43, 9828 CrossRef CAS.
- G. M. Eichenbaum, P. F. Kiser, A. V. Dobrynin, S. A. Simon and D. Needham, Macromolecules, 1999, 32, 4867 CrossRef CAS.
- X. Qiu, S. Leporatti, E. Donath and H. Möhwald, Langmuir, 2001, 17, 5375 CrossRef CAS.
- K. Ogawa, B. Wang and E. Kokufuta, Langmuir, 2001, 17, 4704 CrossRef CAS.
- H. Siddique, L. G. Peeva, K. Stoikos, G. Pasparakis, M. Vamvakaki and A. G. Livingston, Ind. Eng. Chem. Res., 2012 DOI:10.1021/ie202999b.
- J.-Y. Kim, J.-Y. Song, E.-J. Lee and S.-K. Park, Colloid Polym. Sci., 2003, 281, 614 CAS.
- D. C. González-Toro, J.-H. Ryu, R. T. Chacko, J. Zhuang and S. Thayumanavan, J. Am. Chem. Soc., 2012, 134, 6964 CrossRef.
- T. E. Kristensen, K. Vestli, K. A. Fredriksen, F. K. Hansen and T. Hansen, Org. Lett., 2009, 11, 2968 CrossRef CAS.
- V. Rodionov, H. Gao, S. Scroggins, D. A. Unruh, A.-J. Avestro and J. M. J. Fréchet, J. Am. Chem. Soc., 2010, 132, 2570 CrossRef CAS.
- Although ProMA is a water soluble monomer it becomes water insoluble upon polymerization. In the system presented here, the ProMA undergoes precipitation polymerization and the water-insoluble oligomers then participate in the emulsion polymerization similarly to PMMA.
- A. Lu, T. P. Smart, T. H. Epps III, D. A. Longbottom and R. K. O'Reilly, Macromolecules, 2011, 44, 7233 CrossRef CAS.
- Turnover number (TON) refers to the number of moles of substrates that one mole of catalyst can convert to product in a given time. In this case, it was determined for each nanogel system after 24 hours.
- J. Dzierzak, E. Bottinelli, G. Berlier, E. Gianotti, E. Stulz, R. M. Kowalczyk and R. Raja, Chem. Commun., 2010, 46, 2805 RSC.
- E. R. Jarvo and S. J. Miller, Tetrahedron, 2002, 58, 2481 CrossRef CAS.
- B. List, L. Hoang and H. J. Martin, Proc. Natl. Acad. Sci. U. S. A., 2004, 101, 5839 CrossRef CAS.
Footnotes |
† Electronic supplementary information (ESI) available: See DOI: 10.1039/c2sc21300a |
‡ These authors contributed equally. |
|
This journal is © The Royal Society of Chemistry 2013 |