DOI:
10.1039/C2MB25345C
(Paper)
Mol. BioSyst., 2013,
9, 143-153
Binding of isoquinoline alkaloids berberine, palmatine and coralyne to hemoglobin: structural and thermodynamic characterization studies†
Received
27th August 2012
, Accepted 8th October 2012
First published on 19th October 2012
Abstract
Berberine, palmatine and coralyne, the isoquinoline alkaloids distributed in many botanical families, are extensively investigated due to their potential therapeutic actions and clinical utilities. In this work, their binding characteristics to hemoglobin (Hb) were studied by UV-vis absorption spectroscopy, fluorescence spectroscopy, circular dichroism spectroscopy, isothermal calorimetric titration and differential scanning calorimetric techniques. The results indicated that all the three alkaloids caused strong fluorescence quenching of Hb by the static quenching mechanism, but with differing quenching efficiencies. There was a single binding site on Hb for these alkaloids. According to the theory of Förster resonance energy transfer, the binding distances between β-Trp37 of Hb and berberine, palmatine and coralyne were evaluated to be 2.78 nm, 2.64 nm and 3.29 nm, respectively. The result of synchronous fluorescence, circular dichroism and 3D fluorescence revealed that the polarity around Trp residues experienced a significant increase in the presence of alkaloids. The binding was favoured by enthalpy and entropy changes. Results of circular dichroism, 3D and synchronous fluorescence studies confirmed that the binding of the alkaloids significantly changed the secondary structure of Hb. The studies revealed that berberine and palmatine bound to a site near to the α1β2 interface on Hb different than coralyne but the affinity of coralyne was one order higher than that of berberine and palmatine.
Introduction
Alkaloids, natural products of plant origin, are gaining increasing importance for their potential therapeutic utility with high potency and low systemic toxicity.1,2 Isoquinolines, the largest group of alkaloids, are one such group useful in a number of therapeutic applications for their anticancer, antimalarial, antitumor, antiviral, antileukemic, anticholesterol and anti-inflammatory effects.3–12 The most important representatives of this group, berberine (BER), the closely related palmatine (PAL) and the synthetic coralyne (COR) (Fig. 1), have been studied by a large number of investigators. These alkaloids have been reported to exhibit antiproliferative activity in vitro and induce apoptosis/necrosis in several cell lines tested.6,13–15 A number of investigations on the anticancer properties of these alkaloids against a variety of different cell lines operating through various mechanisms are reported.16–21 All these data and the emerging new information continue to build up data that are exploitable for potential clinical applications. The anticancer activity of these alkaloids may be deriving from their ability to form strong complexes with nucleic acids, effect telomerase inhibition, induce topoisomerase poisoning and suppress the DNA transcription process. A number of studies on these aspects have been reported.22–31 More recently studies on their binding to serum proteins have also been reported that may explain their distribution in the blood stream.32–36
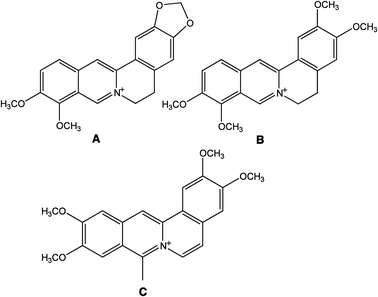 |
| Fig. 1 Chemical structures of (A) berberine, (B) palmatine and (C) coralyne. | |
Human hemoglobin (Hb) is the most abundant blood protein that carries oxygen from lungs to various respiring tissues. Structurally this molecule consists of two α and two β subunits, non-covalently associated with erythrocytes as a tetramer; each α-chain contains 141 amino acid residues and each β-chain contains 146 amino acid residues.37 Hb has the ability to reversibly bind a number of endogenous and exogenous molecules and thus act as a drug carrier for effective delivery to the required physiological site for drug therapy for the treatment of diseases.38–41 Thus a knowledge of the binding characteristics of these therapeutically important alkaloids with Hb is critical for understanding their possible delivery and consequent availability at the required tissues.
Here we studied the interaction of two natural and one synthetic isoquinoline alkaloid with human hemoglobin using spectroscopic and calorimetric techniques. The strong binding of these alkaloids to the protein was evident from spectroscopic results and the structural data have been complemented by the energetic aspects.
Results and discussion
Absorbance spectral studies
The absorption spectrum of Hb has two major peaks in the 190–500 nm region with maximum at 195 nm and 405 nm, respectively; the former is mainly due to the π→π* transitions of the carbonyl (C
O) groups of amino acid residues and the latter being the soret band. In the presence of increasing concentration of the alkaloids, a decrease in the intensity of both bands was observed. Typical absorbance spectral changes in the Hb spectrum in the presence of BER and COR are presented in Fig. 2A and B. In the inset the changes in the 195 and 405 nm bands are highlighted. It can be observed that there is a small blue shift of the wavelength maximum of the 195 nm band in the presence of BER. A similar change was observed for palmatine also (not shown). But for coralyne there is only a hypochromic effect in the 195 nm band. The behaviour of the soret band was similar in all the cases and showed only a decrease in the absorbance. This result suggests the formation of a complex of the alkaloids with Hb. The absorbance data were analyzed by the Benesi–Hildebrand equation in each case, | 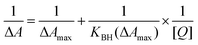 | (1) |
where KBH is the Benesi–Hildebrand binding constant and [Q] is the concentration of alkaloids. This equation gave linear plots (Fig. S1, ESI†) for each case with binding affinity values (KBH) as 6.51 × 104, 8.80 × 104 and 2.14 × 105 M−1, respectively, for BER, PAL and COR. This result suggests that COR has the highest affinity for Hb followed by PAL and BER.
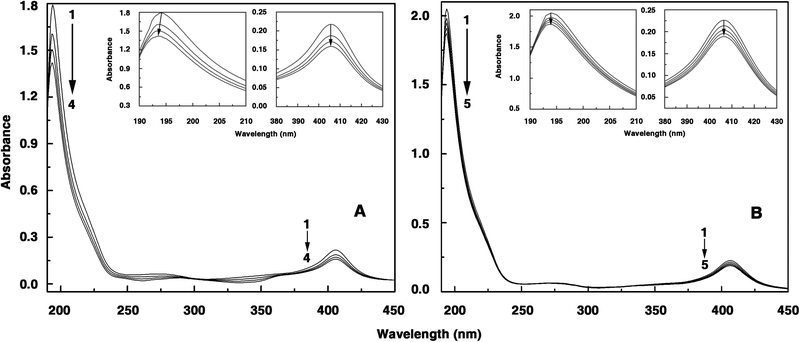 |
| Fig. 2 Representative absorption spectra of hemoglobin in the presence and absence of alkaloids. (A) Hemoglobin (1.21 μM) treated with 0, 6.11, 12.20, 18.26 μM (curves 1–4) of berberine. (B) Hemoglobin (1.30 μM) treated with 0, 0.21, 0.42, 0.63, 0.84 μM (curves 1–5) of coralyne. Inset: 195 nm and 405 nm bands are highlighted in each case. | |
Fluorescence studies have been widely used to understand the interaction of small molecules to proteins.42–46 A number of experiments like steady state quenching, synchronous fluorescence, competitive binding etc. could be performed to understand the various aspects of protein binding phenomena. Changes in the intrinsic fluorescence of Hb may provide information about the nature and mode of interaction. Temperature dependent quenching may provide information about the nature of the quenching. Fluorescence quenching may result from a variety of molecular interactions like ground state complex formation, the excited state interaction, energy transfer, collision etc. Hb contains three intrinsic fluorophores as tryptophan, tyrosine and phenylalanine. Among the six tryptophan residues, two αβ dimers have three Trp residues each as α-Trp14, β-Trp15 and β-Trp37.47 Moreover, there are also five tyrosine residues in each αβ dimer as α-Tyr24, α-Tyr42, α-Tyr140, β-Tyr34 and β-Tyr144.48 The primary fluorescence emission of the Hb molecule has been identified to be from the β-Trp37 residue located at the α1β2 interface.49 Hence, a study of the change in intrinsic fluorescence of Hb in the presence of the alkaloids may give information about the local environment of the Trp moiety on interaction. Hb exhibits a strong fluorescence emission with a peak at 329 nm on excitation at 295 nm. The fluorescence spectral changes of Hb in the presence of BER, PAL and COR are illustrated in Fig. 3. In the presence of increasing concentrations of the alkaloids, quenching of the fluorescence of the Hb was observed, finally reaching saturation in each case. In the case of BER and PAL a blue shift of the peak maximum by 8 and 9 nm, respectively, was observed while a red shift of the peak maximum by 12 nm was observed with coralyne. The result suggests that the alkaloids interact with Hb and the fluorescence quenching was due to specific complex formation.
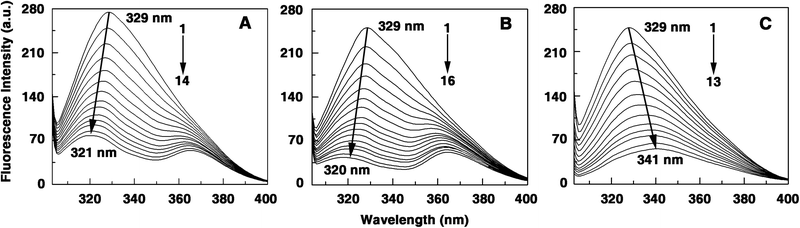 |
| Fig. 3 Steady state fluorescence emission spectra of hemoglobin (10 μM) treated with various concentrations of (A) berberine, (B) palmatine and (C) coralyne in 10 mM CP buffer, pH = 7.2. In panel (A) curves (1–14) denote 0, 3.1, 6.1, 9.1, 12.2, 15.2, 18.3, 21.3, 24.3, 27.3, 30.3, 33.3, 36.3 and 39.2 μM of berberine, (B) curves (1–16) denote 0, 2.7, 5.5, 8.2, 10.9, 13.6, 16.3, 19.1, 21.7, 24.4, 27.1, 29.8, 32.4, 35.2, 37.8 and 40.5 μM of palmatine and (C) curves (1–13) denote 0, 0.9, 1.8, 2.7, 4.5, 6.3, 8.1, 9.9, 11.7, 13.4, 14.3, 16.9 and 18.7 μM of coralyne, respectively. | |
In order to determine the fluorescence quenching mechanism, quenching experiments were performed at different temperatures viz., 15, 25 and 35 °C and the data were analyzed by the classical Stern–Volmer equation50
| 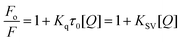 | (2) |
where
Fo and
F are the fluorescence intensities in the absence and presence of the quencher,
Kq is the quenching rate constant,
Ksv is the dynamic quenching constant,
τ0 is the average life time of the
protein in the absence of quencher which is of the order of 10
−8 s
51 and [
Q] is the concentration of the free quencher, respectively. Fig. S2 (ESI
†) shows the Stern–Volmer plots of
Fo/
F versus [
Q] at the three temperatures and the calculated
Ksv and
Kq values are summarized in
Table 1. The results showed that the values of
Ksv and
Kq decreased with increasing temperature suggesting that the quenching mechanism was due to complex formation rather than dynamic collision.
50 In other words, the quenching of the fluorescence of Hb by all the three
alkaloids was due to specific complex formation and dynamic collision could be negligible.
Table 1 Binding data derived for alkaloids binding to hemoglobin from spectrofluorimetric studies at different temperatures
Alkaloids |
Temp./°C |
Stern–Volmer quenching constant (KSV) M−1 |
Quenching rate constant (Kq) M−1 s−1 |
Apparent binding constant (KA) M−1 |
Correlation coefficient for KA |
Lineweaver–Burk constant (KLB) M−1 |
(τ0 = ∼10−8 s). |
Berberine |
15 |
7.84 × 104 |
7.84 × 1012 |
8.13 × 104 |
0.9723 |
7.15 × 104 |
25 |
6.37 × 104 |
6.37 × 1012 |
6.25 × 104 |
1.0491 |
6.03 × 104 |
35 |
5.38 × 104 |
5.38 × 1012 |
5.27 × 104 |
1.0801 |
4.87 × 104 |
Palmatine |
15 |
1.39 × 105 |
1.39 × 1013 |
1.40 × 105 |
0.8550 |
1.19 × 105 |
25 |
0.87 × 105 |
0.87 × 1013 |
0.83 × 105 |
0.9382 |
0.79 × 105 |
35 |
0.72 × 105 |
0.72 × 1013 |
0.69 × 105 |
0.9943 |
0.62 × 105 |
Coralyne |
15 |
2.08 × 105 |
2.08 × 1013 |
2.06 × 105 |
0.9305 |
1.61 × 105 |
25 |
1.15 × 105 |
1.15 × 1013 |
1.16 × 105 |
1.1284 |
1.18 × 105 |
35 |
0.58 × 105 |
0.58 × 1013 |
0.65 × 105 |
1.2266 |
0.80 × 105 |
For the static quenching mechanism, if it is assumed that there are independent binding sites to a set of equivalent sites on the protein, the apparent binding constant and the number of binding sites can be determined from the following equation52,53
| 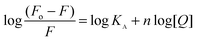 | (3) |
where
KA is the binding constant to a site and
n is the number of binding sites per
protein. From the linear plots of log (
F0−
F/
F)
versus log[
Q] (not shown) for BER, PAL and COR the binding affinity values to Hb obtained at the three temperatures are presented in
Table 1. The higher binding affinity of COR (
KA = 1.16 × 10
5 M
−1) compared to BER (
KA = 6.25 × 10
4 M
−1) and PAL (
KA = 8.3 × 10
4 M
−1) was also apparent from these data. The magnitude of
KA suggests that there is a strong interaction of the
alkaloids with Hb and even very small concentrations in the body can interact with Hb easily. The
n values were found to be around one, suggesting one binding site for these
alkaloids around the Trp residue of the
protein. The 1
![[thin space (1/6-em)]](https://www.rsc.org/images/entities/char_2009.gif)
:
![[thin space (1/6-em)]](https://www.rsc.org/images/entities/char_2009.gif)
1 binding of these
alkaloids on Hb was further confirmed from Job's plot analysis in fluorescences by varying the
alkaloid![[thin space (1/6-em)]](https://www.rsc.org/images/entities/char_2009.gif)
:
protein molar ratio while keeping the total molar concentration constant. The difference in fluorescence at 329 nm
versus mole fraction of
alkaloids (
X) crossed at 0.48, 0.48 and 0.49, respectively, for BER, PAL and COR (figures not shown), yielding the number of each of these molecules binding on the
protein to be around 1.0.
The quenching data were further analyzed by the Lineweaver–Burk equation54,55
| 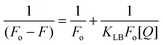 | (4) |
where the static quenching constants (
KLB) were obtained from the ratio of the intercept to the slope of the Lineweaver–Burk plot, describing the efficiency of quenching at the ground state. The plots of
hemoglobin–
alkaloid interactions at three temperatures are presented in Fig. S3, ESI.
† The data depicted in
Table 1 revealed that the value is higher for COR followed by PAL and then BER. The decreasing trend in
KLB with increasing temperature for all the
alkaloids was in accordance with the temperature dependence of
Ksv values and is consistent with the static quenching mechanism.
Energy transfer to the alkaloids and the binding distance measurement
The fluorescence resonance energy transfer (FRET) is a good technique to determine the distance of separation between the donor (fluorophore) and the acceptor and hence useful for realizing structural and conformational distribution of donor–acceptor complexes.56 The FRET efficiency depends on the extent of spectral overlap of the donor emission and acceptor absorption, orientation of the transition dipole of the donor, and the distance between the donor and acceptor which must be within the Förster distance of 2–8 nm.57 The overlap of the absorbance spectra of the alkaloids with the emission spectrum of Hb is presented in Fig. S4, ESI.† In a proteinous environment, the proximity of the ligand molecule to the tryptophan moiety is often determined through the FRET study. The efficiency (E) of the FRET process depends on the inverse sixth power of the distance between the donor and acceptor (r) and of the critical energy transfer distance or Förster radius (Ro). When the efficiency of transfer is 50%, a condition of 1
:
1 situation of donor to acceptor concentration prevails and E is expressed by the equation | 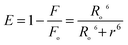 | (5) |
Ro can be calculated using the relation | Ro6 = 8.8 × 10−25k2n−4φJ | (6) |
where k2 is the spatial factor of orientation, n is the refractive index of the medium, φ is the fluorescence quantum yield of the donor and J is the overlap integral of the fluorescence emission spectrum for the donor and the absorption spectrum of the acceptor. J may be calculated from the equation, | 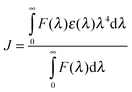 | (7) |
where F(λ) and ε(λ) represent the fluorescence intensity of the donor and the molar absorption coefficient of the acceptor, respectively, at the wavelength λ. Using the values of k2 = 2/3, n = 1.36 and φ = 0.062 for Hb,58 the values of E, J, Ro and r have been calculated to be 0.29, 1.769 × 10−14cm3 L mol−1, 2.39 nm and 2.79 nm for BER–Hb, 0.37, 1.91 × 10−14cm3 L mol−1, 2.41 nm and 2.64 nm for PAL-Hb and 0.05, 0.64 × 10−14 cm3 L mol−1, 2.02 nm and 3.29 nm for COR-Hb interaction. The distances between the ligands and the Trp residues are far lower than the 7 nm value indicating a high probability of energy transfer from the Trp residues of the protein to the alkaloids.59 Furthermore, the fact that r was bigger than Ro also implied that BER, PAL and COR could accept energy from β-Trp37 effectively60 and PAL was closer to β-Trp37 than BER. This is also in accordance with the static quenching mechanism. The results indicated that the conditions of Förster energy transfer for the binding are obeyed and the binding site spans a distance of 2.79 nm, 2.64 nm and 3.29 nm from β-Trp37 which is in the α1β2 interface for BER, PAL and COR.
BER and PAL are weakly fluorescent molecules with emission maxima around 445 nm when excited at 345 nm.24,31 COR, on the other hand, is a strong fluorophore with a maximum at 470 nm when excited at 420 nm.25 In the presence of increasing concentrations of Hb, only a marginal change of the weak fluorescence of BER and PAL was observed. But the fluorescence intensity of coralyne was rapidly quenched in the presence of Hb (Fig. S5, ESI†), indicating the formation of a stronger Hb–coralyne complex compared to BER and PAL-Hb complexes.
The interaction of the three alkaloids with Hb was investigated from isothermal titration calorimetry (ITC) studies. ITC results may provide key insights into the molecular forces that drive the complex formation.61 Thermodynamic parameters like Gibbs energy change (ΔG°), enthalpy of binding (ΔH°), the entropy contribution (TΔS°) and also the affinity (Kb) and stoichiometry (N) can be obtained, which may be correlated to the results of other experiments. In Fig. 4 the representative calorimetric profiles of the titration of BER, PAL and COR with Hb are presented. The binding is an exothermic process and has a single binding event in all the cases (curves in the upper panel). The heat liberated in each injection was corrected for the heat of dilution (curves in the upper panel offset for clarity) that was determined in a separate but identical experiment injecting protein into buffer alone. The resulting values were plotted against the molar ratio of protein/alkaloid and fitted to a one-site model by the nonlinear least square method (curves in the lower panel). The equilibrium constant, binding stoichiometry, enthalpy change, entropy contribution, and Gibbs energy change obtained from the calorimetric data are summarized in Table 2. The binding constants of BER, PAL and COR to Hb at 25 °C were evaluated to be (6.49 ± 0.24) × 104 M−1, (8.70 ± 0.40) × 104 M−1 and (1.15 ± 0.08) × 105 M−1, respectively. The stronger binding of COR to Hb and the variation of the affinity as COR > PAL > BER is thus evident from the calorimetric data and confirms the spectroscopic results.
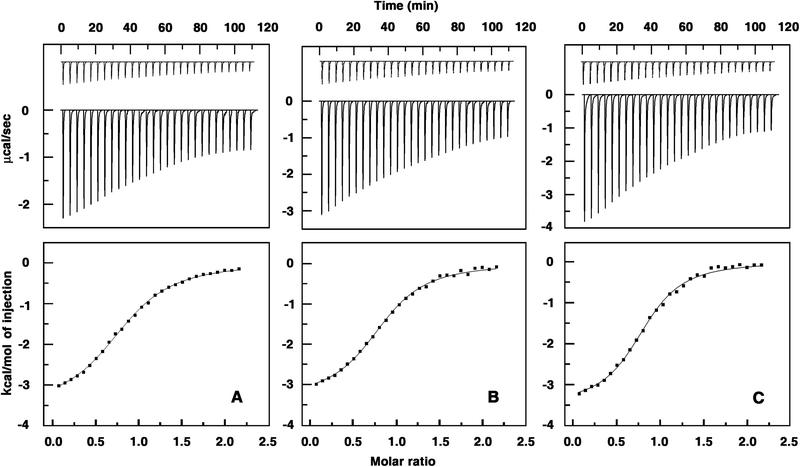 |
| Fig. 4 ITC profiles for the binding of alkaloids to Hb. Top panels present raw results for the sequential injection of hemoglobin (1.0 mM) into solutions of (A) berberine (0.1 mM), (B) palmatine (0.1 mM) and (C) coralyne (0.1 mM) in CP buffer, pH = 7.2 at 25 °C and dilution of Hb into buffer (not in scale). The bottom panels show the integrated heat results after correction of heat of dilution against the mole ratio of Hb/alkaloids. The data points were fitted to the one site model and the solid lines represent the best-fit data. | |
Alkaloids |
Temp./°C |
Binding constant (Kb) |
(N) |
(ΔH°)/kcal mol−1 |
(TΔS°)/kcal mol−1 |
(ΔG°)/kcal mol−1 |
(ΔCop)/cal mol K−1 |
All the data in this table are derived from ITC experiments conducted in CP buffer of 10 mM [Na+], pH 7.2, and are averages of four determinations. Kb and ΔH° values were determined from ITC profiles fitting to Origin 7 software as described in the text.
|
Berberine |
15 |
(7.90 ± 0.28) × 104 |
1.16 |
−1.27 ± 0.02 |
5.21 |
−6.49 ± 0.02 |
|
25 |
(6.49 ± 0.24) × 104 |
1.16 |
−3.04 ± 0.02 |
3.61 |
−6.65 ± 0.03 |
−177.50 |
35 |
(5.41 ± 0.19) × 104 |
1.21 |
−4.82 ± 0.03 |
1.52 |
−6.34 ± 0.03 |
|
Palmatine |
15 |
(1.42 ± 0.19) × 105 |
1.17 |
−1.24 ± 0.02 |
5.62 |
−6.86 ± 0.02 |
|
25 |
(0.87 ± 0.04) × 105 |
1.17 |
−2.86 ± 0.02 |
3.88 |
−6.73 ± 0.02 |
−174.00 |
35 |
(0.72 ± 0.04) × 105 |
1.21 |
−4.72 ± 0.04 |
1.80 |
−6.52 ± 0.04 |
|
Coralyne |
15 |
(2.15 ± 0.17) × 105 |
1.17 |
−1.53 ± 0.02 |
5.56 |
−7.08 ± 0.02 |
|
25 |
(1.15 ± 0.08) × 105 |
1.21 |
−2.89 ± 0.03 |
4.11 |
−7.00 ± 0.03 |
−153.00 |
35 |
(0.53 ± 0.01) × 105 |
1.21 |
−4.59 ± 0.03 |
1.76 |
−6.34 ± 0.03 |
|
The number of binding sites for BER, PAL and COR to Hb was around 1.0 for 1
:
1 binding supporting the results from Job's plot analysis and Lineweaver–Burk analysis. The Gibbs energy change for COR was slightly higher by about 0.27 kcal mol−1 than PAL and larger than 0.35 kcal mol−1 compared to BER. The energetics of the interaction are similar in all the cases except for small changes; the binding of all the three alkaloids was favored by enthalpy and entropic changes, the enthalpy being a dominant contribution to the Gibbs energy. The forces driving the interaction between the alkaloids and the protein were then examined as a function of temperature (15–35 °C). The binding isotherms at different temperatures were found to be sigmoidal (not shown). Overall, with increasing temperature, the affinity values decreased, and the binding enthalpies became negative with increasing magnitudes. The negative enthalpy of binding at all the temperatures indicated favorable exothermic binding of the alkaloids with Hb. In all the cases, the entropy contributions decreased with increasing temperature but remained a favorable factor to the binding even at 35 °C. This suggests that the binding is driven by a dominant entropy contribution at lower temperatures though the enthalpy of binding becomes the dominant force at higher temperatures. Based on the variation of the enthalpy with temperature, ITC can provide information on the heat capacity changes (ΔCop) accompanying the binding.62 The observed enthalpy values varied linearly with the experimental temperature in the range of 15–35 °C (Fig. 5) for the binding of the three alkaloids, proving that there is no measurable shift in the pre-existing equilibrium between the conformational states of the proteins in the temperature range studied. Strong enthalpy–entropy compensation was however observed making Gibbs energy of the binding nearly independent of the temperature. To determine the change in heat capacity, the first derivative of temperature dependence of enthalpy change, the data were plotted as ΔH° versus temperature to yield ΔCop values of −178, −174 and −153 cal mol−1 K−1, respectively, for the binding of BER, PAL and COR to Hb (Table 2). The negative values of ΔCop in all the three cases indicate that the binding is specific and accompanied by burial of the non-polar surface area.63,64 In addition, the negative heat capacity also causes the shift from an entropy dominated binding to an enthalpy dominated one as the temperature rises. The difference in the values between BER and PAL, and COR indicates the different extent of hydrophobic interaction in these systems.
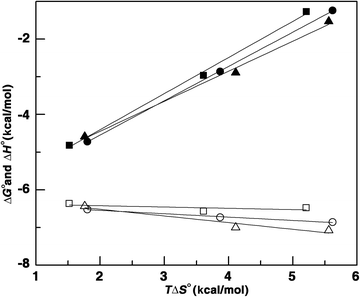 |
| Fig. 5 Plot of variation of thermodynamic parameters for alkaloids–Hb complex formation. ΔG° (filled symbol) and ΔH° (open symbol) versus TΔS° for the binding of berberine (■), palmatine (▲) and coralyne (●) with Hb. | |
We studied the melting of the protein by differential scanning calorimetry and found that a single transition occurred with a Tm value of 59.23 °C (Table 3). The ratio between the calorimetric enthalpy (ΔHcal) and the van't Hoff enthalpy (ΔHv) (Table 3) obtained for the thermal unfolding is not unity indicating that the melting is not exhibiting a simple two state unfolding behavior. In the presence of BER and PAL, there was stabilization of about 5 °C while the melting temperature was reduced by 4 °C in the presence of COR indicating that the binding of the alkaloids effected differential effects on the melting transition of Hb. BER and PAL stabilized the temperature dependent transition, while COR destabilized the protein (Table 3). A similar effect was also observed from absorbance melting experiments (Fig. S6, ESI†). Some ligands have been shown to induce strong conformational changes in serum proteins at the same time stabilizing the protein thermally.65
Table 3 Optical melting and DSC data for hemoglobin–alkaloids complexesa
System |
T
m/°C (optical melting) |
T
m/°C (DSC) |
ΔHcal/kcal mol−1 |
ΔHV/kcal mol−1 |
Melting stabilization of hemoglobin in the presence of saturating concentrations of the alkaloids in CP buffer of 10 mM [Na+], pH 7.2. ΔHcal and ΔHV were obtained from the DSC data.
|
Hemoglobin |
59.23 |
58.83 ± 0.09 |
142.6 ± 2.12 |
61.69 ± 1.13 |
Hemoglobin–berberine complex |
64.78 |
63.39 ± 0.04 |
94.62 ± 1.06 |
100.60 ± 1.40 |
Hemoglobin–palmatine complex |
64.57 |
64.13 ± 0.03 |
115.6 ± 0.86 |
82.78 ± 0.76 |
Hemoglobin–coralyne complex |
55.06 |
55.70 ± 0.05 |
83.42 ± 0.99 |
89.35 ± 1.31 |
Probing the site of binding of the alkaloids on hemoglobin
No specific sites of binding for small molecules have been assigned on hemoglobin as per the present literature. Recently the small molecule docetaxel and the cationic ligand doxorubicin have been suggested to bind to the central cavity of Hb.66,67 We carried out competitive fluorescence experiments with 1,8-anilinonaphthalene sulfonic (ANS) acid, known to bind to the hydrophobic region of the central cavity of hemoglobin.68 The alkaloids were added to a Hb solution and a mixture of ANS–Hb in 1
:
1 and 5
:
1 ratios. The relative fluorescence intensity (F/Fo), where F and Fo are the fluorescence intensity of hemoglobin in the presence and absence of quencher versus concentration of quencher i.e. alkaloids were plotted (Fig. S7, ESI†). The results revealed that the binding of the alkaloids to Hb was only marginally affected by ANS–Hb complex formation. These observations suggest that the alkaloids do not bind to the site of binding of ANS viz. the central cavity.
Conformational studies from synchronous fluorescence
Conformational changes occurring in the protein on binding of the alkaloids were evaluated using synchronous fluorescence.69 When the scanning interval between the excitation and emissions wavelength is stabilized at 15 and 60 nm, respectively, synchronous fluorescence of the protein is characteristic of Tyr and Trp residues; fluorescence quenching caused by the ligand then implies alteration of the polarity around these residues.70 The effect of alkaloids on the synchronous fluorescence of Hb with Δλ = 60 nm revealed that the fluorescence intensity diminished systematically with a large red shift of the emission maxima by 19 and 17 nm for BER and PAL but a small red shift of about 5 nm for COR binding to Hb (Fig. 6A–C). A large red shift is indicative of change from a polar environment to a more hydrophilic environment. This also indicates that in the presence of BER and PAL, Trp residues which were in a polar environment is more exposed to the solvent compared to that on the binding of COR. Comparatively, there is almost no shift in the maximum emission wavelength (not shown) using Δλ = 15 nm, showing that little transformation takes place in the microenvironment around tyrosines. Therefore alkaloids changed the polarity around β-Trp37, while that around Tyr was not changed, in agreement with the results from quenching and FRET experiments implicating unequivocally the involvement of Trp residues in the binding process.
![Synchronous fluorescence (Δλ = 60 nm) spectra of Hb in the presence of different concentrations of (A) berberine, (B) palmatine and (C) coralyne. [Hb] = 10 μM. In panel (A) curves (1–16) denote 0, 3, 6, 9, 12, 15, 18, 21, 24, 27, 30, 33, 36, 39, 42 and 45 μM of berberine, panel (B) curves (1–16) denote 0, 2.7, 5.4, 8.2, 10.9, 13.6, 16.3, 19.1, 21.7, 24.4, 27.2, 29.8, 32.5, 35.2, 37.8 and 40.5 μM of palmatine and panel (C) curves (1–16) denote 0, 0.9, 2.7, 4.5, 6.3, 8.2, 9.9, 11.7, 13.4, 15.2, 16.9, 18.7, 20.6, 22.2, 23.9 and 25.6 μM of coralyne.](/image/article/2013/MB/c2mb25345c/c2mb25345c-f6.gif) |
| Fig. 6 Synchronous fluorescence (Δλ = 60 nm) spectra of Hb in the presence of different concentrations of (A) berberine, (B) palmatine and (C) coralyne. [Hb] = 10 μM. In panel (A) curves (1–16) denote 0, 3, 6, 9, 12, 15, 18, 21, 24, 27, 30, 33, 36, 39, 42 and 45 μM of berberine, panel (B) curves (1–16) denote 0, 2.7, 5.4, 8.2, 10.9, 13.6, 16.3, 19.1, 21.7, 24.4, 27.2, 29.8, 32.5, 35.2, 37.8 and 40.5 μM of palmatine and panel (C) curves (1–16) denote 0, 0.9, 2.7, 4.5, 6.3, 8.2, 9.9, 11.7, 13.4, 15.2, 16.9, 18.7, 20.6, 22.2, 23.9 and 25.6 μM of coralyne. | |
Circular dichroism spectroscopy
To understand the effect of the binding of alkaloids on the secondary structure of the protein, CD measurements were performed. The far ultraviolet CD spectrum of native Hb contained two negative minima at 208 nm and 222 nm, which are characteristic of the α-helical structure. The 208 nm band corresponds to π–π* transition of the α-helix and the 222 nm band is due to n–π* transition for both the α-helix and random coil. In the presence of BER, PAL and COR, the CD signal of the protein decreased, suggesting that the binding of the alkaloids induced secondary structural changes in the conformation of Hb. The alkaloids are optically inactive and hence do not have any CD spectra in the region of the study. The helical content of the free and alkaloid bound Hb molecules were calculated in terms of the mean residue ellipticity (MRE) (deg cm2 dmol−1) as reported71,72 | 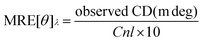 | (8) |
where C is the molar protein concentration, n is the number of amino acid residues and l is the path length of the cuvette.
The alpha helical content of the free and alkaloid bound Hb was calculated from the MRE value at 222 nm using the following equation
| 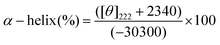 | (9) |
The secondary structure of Hb was found to contain ∼38.0% α-helix. This is very close to the reported 40% α-helicity at pH 7.0.73 Upon titration with increasing concentrations of the alkaloids, the CD spectrum of Hb decreased in intensity without any shift in the peaks, indicating a decrease in the helical structure (Fig. 7). At saturation, corresponding to 14, 19 and 47 μM, respectively, of BER, PAL and COR the helical content was reduced to 2, 3 and 4%, respectively. Thus, the unfolding and loss of a large part of the helical stability has been observed on binding inducing strong secondary structural changes in the protein. It appears that interaction with the alkaloids leads to an unfolded conformation of Hb with extended polypeptide chains exposing the hydrophobic cavities with concomitant exposure of the aromatic amino acid residues. However, soret band CD spectra of Hb at 413 nm decreased on binding of BER and PAL but in the presence of COR no significant change was seen suggesting that the COR binding region of Hb is different from that of BER and PAL. It is worth mentioning here that the alkaloids did not acquire any induced optical activity on binding to Hb molecules.
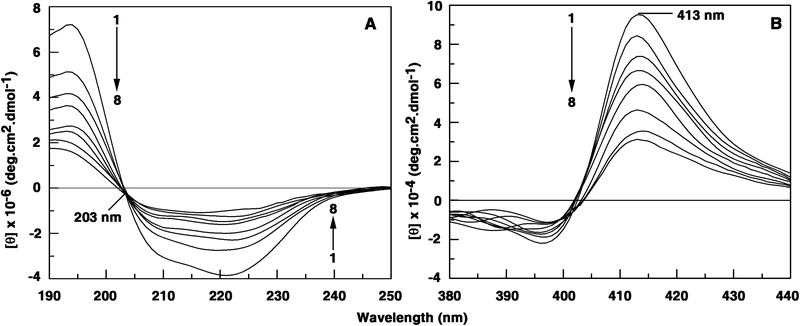 |
| Fig. 7 (panel A) Intrinsic circular dichroism (far UV CD) spectral changes of Hb (1 μM) on interaction with 0, 2.23, 4.46, 6.66, 8.86, 11.05, 13.23 and 15.39 μM of palmatine. (panel B) Soret band CD spectral changes of Hb (5 μM) on interaction with 0, 2.67, 5.35, 8.02, 10.67, 13.33, 15.98 and 18.63 μM of palmatine. | |
This is a relatively new technique that is useful to understand the conformational changes in a protein on interaction of a ligand on changing excitation and emission wavelength simultaneously. A comparative study of the 3D fluorescence spectra can provide conformational and micro environmental changes in the protein. The representative 3D fluorescence spectra and the contour maps of Hb in the absence and presence of BER and PAL are presented in Fig. 8 and corresponding parameters are listed in Table 4. In Fig. 8A, peak a and peak b represent first order Rayleigh scattering (λex = λem) and second order Rayleigh scattering peak (λex = 2λem), respectively. Peak 1 (λex = 280 nm, λem = 340 nm) is the characteristic intrinsic fluorescence spectral behavior of Trp and Try residues.74 On binding of the alkaloids to Hb it was observed that the fluorescence intensity decreased and Stokes (Δλ) shift increased. The increase of Stokes shift proved that the conformation of Hb was changed, the polarity around the Trp residues increased and the hydrophobicity decreased, which is consistent with the synchronous fluorescence data. Moreover, the Stokes shift value of the Hb–PAL complex was higher than that of Hb–BER and Hb–COR indicating that the energy transfer from Trp to alkaloids is higher in the case of PAL. Peak 2 (λex = 230 nm, λem = 338 nm) in Hb is mainly caused by the characteristic transition of n → π* of the polypeptide backbone. In the Hb–alkaloid complex, the fluorescence intensity of both the peaks decreased but to different extents. So the interaction of these alkaloids with Hb induced unfolding of the polypeptide chain resulting in conformational changes that increases the exposure of some hydrophobic regions from a non-polar to a polar environment. Peak 3 in the Hb–BER and Hb–PAL complex may be due to energy transfer from Hb to alkaloids. Peak 3 in Hb–COR system is absent (not shown). This may be due to weak energy transfer from Hb to COR. This was supported by a small overlap of the absorption and fluorescence spectra and the small energy transfer efficiency (vide supra).
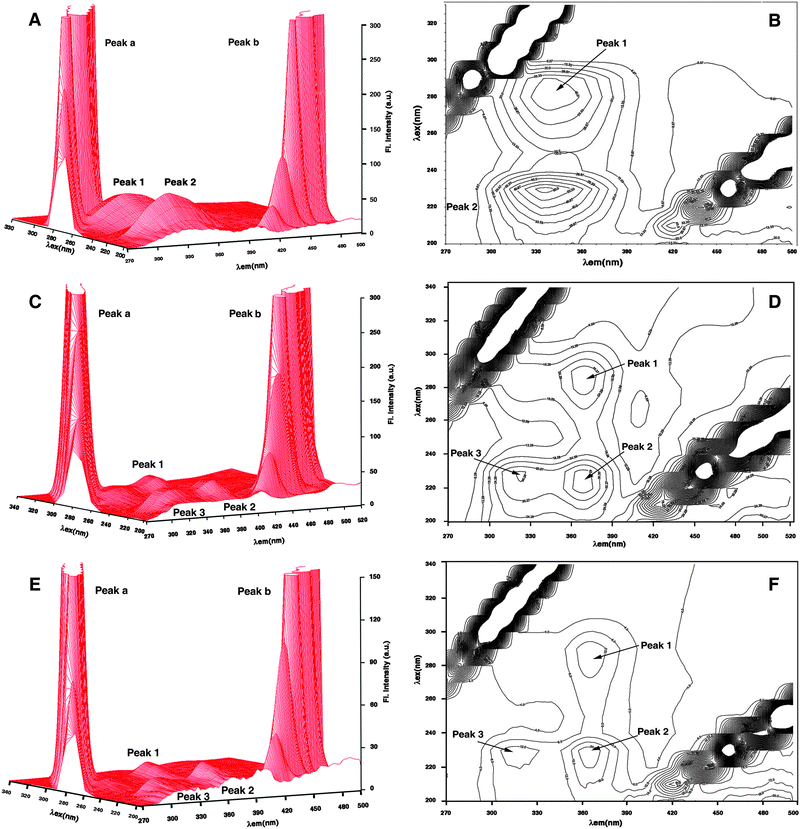 |
| Fig. 8 Three-dimensional fluorescence and contour spectra of Hb (A, B), Hb–berberine (C, D) and Hb–palmatine (E, F) complexes. | |
System |
Fluorescence peak 1 |
Fluorescence peak 2 |
Fluorescence peak 3 |
Peak position (λex/λem/intensity) (nm/nm/F) |
Stokes shift Δλ/nm |
Peak position (λex/λem/intensity) (nm/nm/F) |
Stokes shift Δλ/nm |
Peak position (λex/λem/intensity) (nm/nm/F) |
Stokes shift Δλ/nm |
Hemoglobin |
280/340/45.87 |
60 |
230/338/62.99 |
108 |
|
|
Hemoglobin–berberine |
280/365/28.33 |
85 |
230/368/44.62 |
138 |
285/326/23.50 |
41 |
Hemoglobin–palmatine |
280/368/18.79 |
88 |
230/370/22.75 |
140 |
285/320/7.20 |
35 |
Hemoglobin–coralyne |
280/353/38.04 |
73 |
230/353/23.42 |
123 |
|
|
Conclusions
Natural alkaloids are emerging as potential therapeutic drugs and hence an understanding of their distribution in the plasma may be useful for employing them as drugs. In this study the structural aspects and thermodynamics of the binding of three alkaloids with hemoglobin have been probed using a variety of biophysical techniques. The results presented reveal that the natural isoquinoline alkaloids berberine and palmatine and their synthetic analogue coralyne bind strongly to hemoglobin. Strong interaction with Hb has been confirmed from absorbance spectra and fluorescence quenching studies. The interaction involves close contact of the alkaloids with the β-Trp37 which is at the α1β2 interface. The binding involves static quenching due to ground state complexation with affinity of the order of 104 M−1 for BER and PAL and one order higher at 105 M−1 for COR. Fluorescence techniques have been effectively utilized to understand the proximity of these alkaloids to the protein residues. FRET studies have confirmed that the alkaloids are bound close to β-Trp37 of Hb; BER and PAL are more closer to this residue than COR. Thus the binding of the alkaloids is in the vicinity of β-Trp37 in the α1β2 interface. Thermodynamics of the interaction revealed that the binding involves a strong hydrophobic interaction along with electrostatic interactions from the charged alkaloids. The binding of the alkaloids induced strong secondary structural changes in the protein structure, and this was confirmed from synchronous fluorescence and 3D fluorescence. Again, the results suggested a significant difference between the behaviour of BER and PAL, and COR indicating that COR is bound at a site different from BER and PAL. The presence of bound 1,8-anilinonaphthalene sulfonic acid known to bind to the central cavity of Hb has not affected the binding of any of these alkaloids suggesting a binding site different from the central cavity for these alkaloids on Hb. Although the precise location of the binding sites of these alkaloids on Hb is not revealed, these results suggest the differential binding ability of hemoglobin to deliver these therapeutic alkaloids to physiological targets.
Experimental section
Materials
Berberine chloride, palmatine chloride, coralyne chloride and human methemoglobin were obtained from Sigma-Aldrich Corporation (St. Louis, MO, USA). The concentration of hemoglobin was determined by the molar extinction coefficient (ε) value of 179
000 M−1 cm−1 at 405 nm.75 The alkaloids were used as received. They were fairly soluble in aqueous buffers, and hence their solutions were freshly prepared each day and kept protected in the dark until use. The concentration of alkaloids were determined by an ε value of 22
500 M−1 cm−1 at 345 nm for BER, 25
000 M−1 cm−1 at 344.5 nm for PAL and 14
500 M−1 cm−1 at 420 nm for COR. No deviation from Beer's law was observed in the concentration range used in this study. All experiments were conducted using citrate-phosphate (CP) buffer (10 mM Na+) of pH 7.2, containing 5 mM Na2HPO4. The pH was adjusted using citric acid. pH measurements were made with a Sartorius PB-11 high precision bench pH meter (Sartorius GmBH, Germany) with an accuracy of >±0.01. All other chemicals used were of analytical grade and obtained from Sigma. Glass distilled deionized water and analytical grade reagents were used throughout. All buffer solutions were filtered through Millipore filters (Millipore India Pvt. Ltd, Bangalore, India) of 0.45 μm.
Apparatus and measurements
Electronic absorption spectroscopy.
Absorbance spectral studies were performed with a Jasco V660 double beam double monochromator spectrophotometer (Japan International Co., Hachioji, Japan) at 25 ± 0.5 °C. For titration, matched quartz cuvettes (Hellma, Germany) of 1 cm path length were used. Briefly, a known concentration of the protein solution was kept in the sample cuvette and small aliquots of a known concentration of the alkaloid solution were titrated into the sample and a reference cuvette. After each addition, the solution was thoroughly mixed and allowed to re-equilibrate for at least 10 min before noting the absorbance at the desired wavelength maxima.
Fluorescence measurements.
Steady state fluorescence was performed on either a Shimadzu RF-5301 PC (Shimadzu Corporation, Kyoto, Japan) or a Hitachi F4010 (Hitachi Ltd., Tokyo, Japan) fluorescence spectrometer in fluorescence free quartz cells of 1 cm path length. All measurements were performed keeping excitation and emission band passes of 5 nm. The sample temperature was maintained at 25 ± 1.0 °C using an Eyela UniCool U55 water bath (Tokyo Rikakikai Co. Ltd., Tokyo, Japan). No deviation from Beer's law was observed in the concentration range employed in this study.
Intrinsic fluorescence of the proteins was measured by exciting at 295 nm. BER and PAL were excited at 345 nm while coralyne was excited at 420 nm. Temperature dependent fluorescence spectral studies were performed on the Hitachi unit equipped with a circulating water bath. For measurement of synchronous fluorescence the excitation range was 220–340 nm and Δλ was set at 15 and 60 nm.
Isothermal titration calorimetry.
Isothermal titration calorimetry (ITC) experiments were performed with a VP-ITC unit (MicroCal, Inc., Northampton, MA, USA). Hb and alkaloid solutions were degassed on the MicroCal's Thermovac unit before loading to avoid the formation of bubbles in the calorimeter cell. The instrument control, titration and data analysis were performed through the dedicated Origin 7.0 software provided with the unit. The experiments were carried out as follows. The calorimeter syringe was filled with a solution of Hb (1.0 mM). Successive injections of 10 μL aliquots of this protein solution into 0.1 mM solution of the alkaloid contained in the calorimeter cell (volume = 1.4235 mL) was effected from the rotating syringe (611 rpm). The data were corrected for the heats of dilution of Hb, which were determined in a separate set of experiments under identical buffer conditions and temperature. The resulting corrected injection heats were plotted as a function of molar ratio (alkaloid/Hb) and fitted with a model of one set of binding sites and analyzed using the Origin software to provide the binding affinity (Kb), the binding stoichiometry (N), and the enthalpy of complex formation (ΔH°). The binding Gibbs energy (ΔG°) values and the entropy contribution to the binding (TΔS°) were calculated according to the standard relationsandwhere T is the absolute temperature in Kelvin and R is the gas constant (1.987 cal mol−1 K−1). The calorimeter was periodically calibrated electrically and verified with water–water dilution experiments as per the criteria of the manufacturer that the mean energy per injection was <1.30 μcal and standard deviation was <0.015 μcal. In temperature dependent ITC experiments no variation in the buffer pH was observed in the temperature range studied here.
Differential scanning calorimetry.
To investigate the temperature dependent transitions in the protein, excess heat capacities as a function of temperature were measured on a MicroCal VP-differential scanning calorimeter (DSC) (MicroCal, Inc., Northampton, MA, USA) as reported elsewhere.76 In a series of DSC scans, both reference and sample cells were loaded with the buffer solution, equilibrated at 30 °C for 15 min and scanned from 30 °C to 110 °C at a scan rate of 60 °C h−1. The buffer scans were repeated till the base line was reproducible (noise specification < 0.501 μcal °C−1 and repeatability specification < 1.3 μcal °C−1). Typically about 10 to 12 scans were required to obtain a stable instrument base line. On the cooling cycle, the sample cell was rinsed and loaded with a Hb solution and then with the Hb–alkaloid complexes (Hb
:
alkaloid molar ratio = 1
:
2) and scanned in the same range. Each experiment was repeated twice with separate fillings. The DSC thermograms of excess heat capacity versus temperature were analyzed using the Origin 7.0 software.
Circular dichroism (CD) spectroscopy.
The conformational changes in the protein secondary and tertiary structure on binding of the alkaloids were measured in the wavelength region 500 to 190 nm with a Jasco J815 spectropolarimeter at 25 °C equipped with a Peltier controlled cell holder and temperature controller PFD 425 L/15. The protein concentration and path length of the cell used were 1.0 μM and 0.1 cm for far UV CD and 5.0 μM and 1 cm for soret band CD determination. The instrument parameters for CD measurements were a scan speed of 50 nm min−1, band width of 1.0 nm and sensitivity of 100 milli degrees. Five scans were performed, averaged to improve the signal-to-noise ratio, and smoothed within permissible limits. The molar ellipticity values in the region of intrinsic CD were expressed in terms of the mean residue molar ellipticity [θ], in units of deg cm2 dmol−1.
Three-dimensional spectroscopy.
Three-dimensional (3D) fluorescence spectroscopy experiments were performed at 25 °C on a PerkinElmer LS55 fluorescence spectrometer (PerkinElmer, Inc., USA). The fluorescence emission spectra of Hb were measured in the range of 270–500 nm. With an increment of 10 nm, the initial excitation wavelength was set at 200 nm and continued up to 340 nm, i.e. the number of scans was 15. The concentration of Hb was 7.5 μM and the Hb–alkaloid complex ratio was 1
:
5.
Acknowledgements
S. Hazra is a Junior Research Fellow of the Council of Scientific and Industrial Research (CSIR) awarded through the national eligibility test (NET). M. Hossain was a Research Associate of CSIR. The authors thank all the colleagues of the Biophysical Chemistry Laboratory for the help and cooperation throughout the course of this work. The critical and judicious comments of the three anonymous reviewers that enabled us to improve the manuscript are highly appreciated.
References
-
A. Rahman, Studies in Natural Products Chemistry, Bioactive natural products (part B)Elsevier Science BV, Amsterdam, The Netherlands, 2000, vol. 21 Search PubMed.
-
A. E. Osbourn and V. Lanzotti, Plant Derived Natural Products Synthesis, Function, and Applications, Springer, 2009 Search PubMed.
- W. D. Wilson, A. N. Gough, J. J. Doyle and M. W. Davidson, J. Med. Chem., 1976, 19, 1261–1263 CrossRef CAS.
- K. Iwasa, M. Kamigauchi, M. Ueki and M. Taniguchi, Eur. J. Med. Chem., 1996, 31, 469–478 CrossRef CAS.
- T. Schmeler, B. Latz-Brüning and M. Wink, Phytochemistry, 1997, 44, 257–266 CrossRef.
- C. L. Kuo, C. W. Chi and T. Y. Liu, Cancer Lett., 2004, 203, 127–137 CrossRef CAS.
- W. Kong, J. Wei, P. Abidi, M. Lin, S. Inaba, C. Li, Y. Wang, Z. Wang, S. Si, H. Pan, S. Wang, J. Wu, Y. Wang, Z. Li, J. Liu and J.-D. Jiang, Nat. Med., 2004, 10, 1344–1351 CrossRef CAS.
- M. Imanshahidi and H. Hosseinzadeh, Phytother. Res., 2008, 22, 999–1012 CrossRef CAS.
- Y. Sun, K. Xun, Y. Wang and X. Chen, Anticancer Drugs, 2009, 20, 757–769 CrossRef CAS.
- A. Singh, S. Duggal, N. Kaur and J. Singh, J. Nat. Prod., 2010, 3, 64–75 CAS.
- H.-F. Ji and L. Shen, Molecules, 2011, 16, 6732–6740 CrossRef CAS.
- M. Maiti and G. Suresh Kumar, J. Nucleic Acids, 2010 Search PubMed , Article ID 593408, 23 pages.
- I. W. Yang, C. C. Chou and B. Y. M. Yung, Naunyn-Schmiedeberg's Arch. Pharmacol., 1996, 354, 102–108 CrossRef CAS.
- S. Letasiova, S. Jantova, L. Cipak and M. Muckova, Cancer Lett., 2006, 239, 254–262 CrossRef CAS.
- M. S. Choi, J. H. Oh, S. M. Kim, H. Y. Jung, H. S. Yoo, Y. M. Lee, D. C. Moon, S. B. Han and J. T. Hong, Int. J. Oncol., 2009, 34, 1221–1230 CrossRef CAS.
- S. K. Mantena, S. D. Sharma and S. K. Katyar, Mol. Cancer Ther., 2006, 5, 296–308 CrossRef CAS.
- J.-P. Lin, J.-S. Yang, J.-H. Lee, W.-T. Hsieh and J.-G. Chung, World J. Gastroenterol., 2006, 12, 21–28 CAS.
- P. L. Peng, Y. S. Hsiesh, C. J. Wang, J. L. Hsu and F. P. Chou, Toxicol. Appl. Pharmacol., 2006, 214, 8–15 CrossRef CAS.
- J. B. Kim, K. M. Lee, E. Ko, W. Han, J. E. Lee, I. Shin, J. Y. Bae, S. Kim and D. Y. Noh, Planta Med., 2008, 74, 39–42 CrossRef CAS.
- Y. T. Ho, J. S. Yang, C. C. Lu, J. H. Chiang, T. C. Li, J. J. Lin, K. C. Lai, C. L. Liao, J. G. Lin and J. G. Chung, Phytomedicine, 2009, 16, 887–890 CrossRef CAS.
- S. Mahata, A. C. Bharti, S. Shukla, A. Tyagi, S. A. Husain and B. C. Das, Mol. Cancer, 2011, 10, 39–52 CrossRef CAS.
- D. S. Pilch, C. Yu, D. Makhey, E. J. LaVoie, A. R. Srinivasan, W. K. Olson, R. R. Sauers, K. J. Breslauer, N. E. Geacintov and L. F. Liu, Biochemistry, 1997, 36, 12542–12553 CrossRef CAS.
- T.-K. Li, E. Bathory, E. J. LaVoie, A. R. Srinivasan, W. K. Olson, R. R. Sauers, L. F. Liu and D. S. Pilch, Biochemistry, 2000, 39, 7107–7116 CrossRef CAS.
- M. Maiti and G. Suresh Kumar, Med. Res. Rev., 2007, 27, 649–695 CrossRef CAS.
- P. Giri and G. Suresh Kumar, Mol. BioSyst., 2008, 4, 341–348 RSC.
- R. Sinha and G. Suresh Kumar, J. Phys. Chem. B, 2009, 113, 13410–13420 CrossRef CAS.
- M. M. Islam, S. R. Chowdhury and G. Suresh Kumar, J. Phys. Chem. B, 2009, 113, 1210–1224 CrossRef CAS.
- P. Giri and G. Suresh Kumar, Mol. BioSyst., 2010, 6, 81–88 RSC.
- B. S. Patro, B. Maity and S. Chattopadhyay, Antioxid. Redox Signaling, 2010, 12, 945–960 CrossRef CAS.
- K. Bhadra and G. Suresh Kumar, Biochim. Biophys. Acta, 2011, 1810, 485–496 CrossRef CAS.
- K. Bhadra and G. Suresh Kumar, Med. Res. Rev., 2011, 31, 821–862 CrossRef CAS.
- Y. Wang, M. M. Kheir, Y. Chai, J. Hu, D. Xing, F. Lei and L. Du, PLoS One, 2011, 6, e23495 CAS.
- Y.-J. Hu, Y. Liu and X.-H. Xiao, Biomacromolecules, 2009, 10, 517–521 CrossRef CAS.
- Y.-J. Hu, Y. O-Yang, C. M. Dai, Y. Liu and X.-H. Xiao, Biomacromolecules, 2010, 11, 106–112 CrossRef CAS.
- A. Y. Khan, M. Hossain and G. Suresh Kumar, Chemosphere, 2012, 87, 775–781 CrossRef CAS.
- A. Y. Khan, M. Hossain and G. Suresh Kumar, Mol. Biol. Rep. DOI:10.1007/s11033-012-2092-z.
-
http://www.rscb.org/pdb/explore/explore.do?structureId=30dq
.
- R. Mandal, R. Kalke and X. F. Li, Chem. Res. Toxicol., 2004, 17, 1391–1397 CrossRef CAS.
- L. Messori, C. Gabbianii, A. Casini, M. Siragusa, F. F. Vincieri and A. R. Bilia, Bioorg. Med. Chem., 2006, 14, 2972–2977 CrossRef CAS.
- J. C. Jang, H. Liu, W. Chen and G. L. Zou, J. Mol. Struct., 2009, 928, 72–77 CrossRef CAS.
- Y. Q. Wang, H. M. Zhang and Q. H. Zhou, Eur. J. Med. Chem., 2009, 44, 2100–2105 CrossRef CAS.
- S. De and A. Girigoswami, J. Colloid Interface Sci., 2006, 296, 324–331 CrossRef CAS.
- F. Ding, G. Zhao, S. Chen, F. Liu, Y. Suna and L. Zhang, J. Mol. Struct., 2009, 929, 159–166 CrossRef CAS.
- I. Saha, J. Bhattacharyya and G. Suresh Kumar, J. Chem. Thermodyn., 2013, 56, 114–122 CrossRef CAS.
- P. Qin, B. Su and R. Liu, Mol. BioSyst., 2012, 8, 1222–1229 RSC.
- S. Tabassum, W. M. Al-Asbahy, M. Afzal, F. Arjmand and R. H. Khan, Mol. BioSyst., 2012, 8, 2424–2433 RSC.
- S. Venkateshrao and P. T. Manoharan, Spectrochim. Acta, Part A, 2004, 60, 2523–2526 CrossRef CAS.
- T. C. Mueser, P. H. Rogers and A. Arnone, Biochemistry, 2000, 39, 15353–15364 CrossRef CAS.
- B. Alpert, D. M. Jameson and G. Weber, J. Photochem. Photobiol., 1980, 31, 1–4 CrossRef CAS.
-
J. R. Lakowicz, Principles of Fluorescence Spectroscopy, Plenum Press, New York, 1999 Search PubMed.
- J. R. Lakowicz and G. Weber, Biochemistry, 1973, 12, 4161–4170 CrossRef CAS.
- O. K. Abou-Zied and O. L. K. Al-Shihi, J. Am. Chem. Soc., 2008, 130, 10790–10801 CrossRef.
- G. W. Zhang, Q. M. Que, J. H. Pan and J. B. Guo, J. Mol. Struct., 2008, 81, 132–138 CrossRef.
- F.-L. Cui, J. Fan, J.-P. Li and Z. Hu, Bioorg. Med. Chem., 2004, 12, 151–157 CrossRef CAS.
- H.-X. Zhang, X. Huang, P. Mei, K.-H. Li and C.-N. Yan, J. Fluoresc., 2006, 16, 287–294 CrossRef CAS.
- A. Mallick, B. Haldar and N. Chattopadhyay, J. Phys. Chem. B, 2005, 109, 14683–14690 CrossRef CAS.
-
B. Valeur and J. C. Brochon, New Trends in Fluorescence Spectroscopy, Springer-Verlag, Berlin, 1999 Search PubMed.
- A. Haouz, S. E. Mohsni, C. Zentz, F. Merola and B. Alpert, Eur. J. Biochem., 1999, 264, 250–257 CrossRef CAS.
-
B. Valeur, Molecular Fluorescence: Principles and Applications, Wiley-VCH Verlag GmbH, 2001 Search PubMed.
- Y. Q. Yang, H. M. Zhang, G. C. Zhang, S. X. Lui, Q. H. Zhou, Z. H. Fei and Z. T. Liu, Int. J. Biol. Macromol., 2007, 41, 243–250 CrossRef.
- M. W. Freyer and E. A. Lewis, Methods Cell Biol., 2008, 84, 79–113 CrossRef CAS.
- J. B. Chaires, Annu. Rev. Biophys., 2008, 37, 135–151 CrossRef CAS.
- R. S. Spolar and M. T. Record Jr., Science, 1994, 263, 777–784 CAS.
- R. O'Brien, B. DeDecker, K. G. Fleming, P. B. Sigler and J. E. Ladbury, J. Mol. Biol., 1998, 279, 117–125 CrossRef CAS.
- M. Sánchez, F. J. Aranda, M. J. Espuny, A. Marqués, J. A. Teruel, Á. Manresa and A. Ortiz, Langmuir, 2008, 24, 6487–6495 CrossRef.
- H. Cheng, H. Liu, W. Bao and G. Zou, J. Photochem. Photobiol. B, 2011, 105, 126–132 CrossRef CAS.
- S. N. Khan, B. Islam, R. Yennamalli, Q. Zia, N. Subbarao and A. U. Khan, J. Pharm. Biomed. Anal., 2008, 48, 1096–1104 CrossRef CAS.
- V. E. Syakhovich, D. A. Parul, E. Y. Ruta, B. A. Bushuk and S. B. Bokut, Biochem. Biophys. Res. Commun., 2004, 317, 761–767 CrossRef CAS.
- J. B. F. Lloyd, Nat. Phys. Sci., 1971, 231, 64–65 CAS.
- J. N. Miller, Proc. Anal. Div. Chem. Soc., 1979, 16, 203–208 CAS.
- Y. H. Chen, J. T. Yang and H. M. Martinez, Biochemistry, 1972, 11, 4120–4131 CrossRef CAS.
-
Z. X. Lu, T. Cui and Q. L. Shi, Molecular Biology: Applications of Circular Dichroism and Optical Rotatory Dispersion, Science Press, Beijing, 1st edn, 1987 Search PubMed.
- P. Mandal, M. Bardhan and T. Ganguly, J. Photochem. Photobiol. B, 2010, 99, 78–86 CrossRef CAS.
- J. Kang, Y. Liu, M.-X. Xie, S. Li, M. Jiang and Y.-D. Wang, Biochim. Biophys. Acta, 2004, 1674, 205–214 CrossRef CAS.
-
E. Antonini and M. Brunori, Hemoglobin and Myoglobin in their Reactions with Ligands, North-Holland publishing Co., Amsterdam, The Netherlands, 1971 Search PubMed.
- P. Giri and G. Suresh Kumar, Arch. Biochem. Biophys. Acta, 2008, 474, 183–192 CrossRef CAS.
Footnotes |
† Electronic supplementary information (ESI) available: Fig. S1–S7. See DOI: 10.1039/c2mb25345c |
‡ Current address: Department of Chemistry, Vidyasagar University, West Midnapore, West Bengal 721 102, India. |
|
This journal is © The Royal Society of Chemistry 2013 |