DOI:
10.1039/C3GC41769G
(Paper)
Green Chem., 2014,
16, 342-350
Efficient bioconversion of crude glycerol from biodiesel to optically pure D-lactate by metabolically engineered Escherichia coli†
Received
28th August 2013
, Accepted 21st October 2013
First published on 6th November 2013
Abstract
Biodiesel has attracted considerable attention as one of the best choices among alternative and renewable fuels. Large quantities of crude glycerol are produced as a main co-product with increasing biodiesel production. Currently, the problem of waste glycerol utilization needs to be crucially addressed, not only for environmental protection but also for the economy of the biodiesel industry. In this paper, the use of crude glycerol for the production of D-lactate by engineered Escherichia coli was investigated. Engineered E. coli B0013-070 with a homolactic pathway for D-lactate synthesis by elimination of byproduct pathways (ethanol, succinate, formate and acetate) could convert 20 g L−1 of crude glycerol to 13.6 g L−1 of D-lactate with a yield of 0.67 g g−1 glycerol. Overexpression of D-lactate dehydrogenase by a low-copy vector in E. coli B0013-070 resulted in the increased production and yield of D-lactate, in which 14.5 g L−1 of D-lactate was produced with a yield of 0.72 g g−1 glycerol from crude glycerol. The effect of temperature on D-lactate fermentation by the engineered strain E. coli B0013-070-pTHldhA was also investigated, and 34 °C and 40 °C were found to be the optimal temperatures for cell growth and lactate production, respectively. The engineered strain B0013-070-pTHldhA produced 100.3 g L−1 of D-lactate with 99.97% optical purity from 531.5 g of crude glycerol with an overall productivity of 2.78 g L−1 h−1 and a yield of 75.4 g per 100 g glycerol (0.77 mol mol−1) using two phase fermentation combined with a temperature shifting strategy in a 7 L bioreactor. In summary, this paper shows that crude glycerol could be directly converted to D-lactate without any prior purification.
Introduction
Biodiesel (fatty acid methyl esters), one of the best choices among alternative fuels, has attracted considerable attention because it has the potential to completely displace its petroleum counterpart.1 It is produced from triacylglycerols by transesterification with short chain alcohols that employs methanol and alkali catalysis. This process would generate large quantities of glycerol into the market in the near future. It was projected that the world biodiesel market would reach 37 billion gallons by 2016, which implied that approximately 4 billion gallons of crude glycerol would be produced.2 The problem of waste glycerol utilization needs to be crucially addressed if biodiesel usage is to be enhanced.3 There are some chemical applications which utilize waste glycerol, but a purification process is needed to use glycerol as a chemical source.4 Therefore, development of sustainable biorefineries based on crude glycerol is imperative to favor the economy of the biodiesel industry by reducing the costs associated with the disposal of residues and increasing the production of value-added chemicals.5
Microbial conversion of glycerol to high value chemicals such as 1,3-propanediol,6 poly(3-hydroxypropionate),7 succinate8 and lactate9 is attractive in that the process is relatively simple and does not generate toxic byproducts. In most cases, refined glycerol was used to produce a value-added compound. However, the interest in low-grade glycerol as a substrate is high but there are only a few investigations examining the bioconversion of this substance into high value chemicals.10,11
Lactate and its derivatives have versatile applications in the food, pharmaceutical, textile, chemical and polymer industries.12 For example, D-lactate is a critical chiral intermediate and a monomer for the production of polylactate (PLA). The physical properties and the rate of biodegradation of PLA are due to the isomeric forms.13 Manufacturing polylactic acid needs large amounts of the monomer, D-lactic acid and/or L-lactic acid. Therefore, a greater demand for chirally and chemically pure D-lactate is impending with the increase in the global PLA market. However, the relatively high cost of raw materials and the requirement of optically pure monomers of lactate pose major limitations on the commercialization of polylactate. The two basic approaches to overcome this are as follows: (i) to produce D-lactate with higher optical purity using novel strains and (ii) to use cheaper raw materials for monomer production. The first approach is being addressed by overproducing D-lactate using engineered strains and recently a yield of up to 125 g L−1 of D-lactate with a chiral purity of more than 99.9% from glucose by engineered E. coli has been reported.13,14 Another strategy used is utilization of low-priced feedstock such as crude glycerol generated from the biodiesel production process. It was noted that wild-type E. coli exhibited a heterofermentative behavior using glycerol as the substrate under anaerobic and microaerobic conditions, which resulted in the synthesis of significant amounts of ethanol, acetate, succinate, and formate but only negligible production of D-lactate.15Fig. 1 shows that in addition to ATP, NADH is also formed in the glycerol to D-lactate pathway. Actually, this metabolic pathway is an unbalanced-redox reaction under the conditions of limited availability of external electron acceptors (e.g., anaerobic or microaerobic conditions). Therefore, it is important to engineer E. coli strains producing D-lactate from glycerol to eliminate byproduct formation and balance cell growth and product accumulation. Recently, refined glycerol has been successfully used for the production of D-lactate.9 It has also been reported that metabolically engineered E. coli is able to produce 50 g L−1 of L-lactate from 56 g L−1 of crude glycerol at a yield of 93% of the theoretical maximum.16
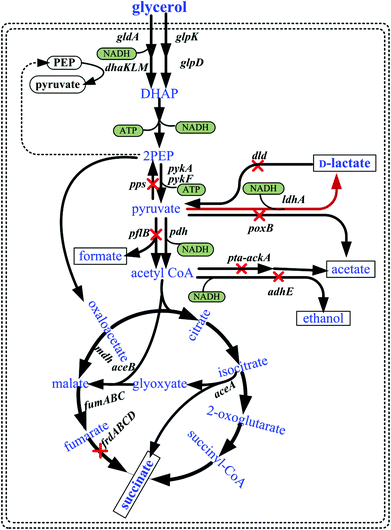 |
| Fig. 1 Metabolic pathways involved in the aerobic and/or microaerobic utilization of glycerol in E. coli and genetic modification strategies for producing D-lactate employed in the present study. Relevant reactions are represented by the names of the gene(s) coding for the enzymes. aceA: isocitrate lyase; aceB: malate synthase; ackA, acetate kinase gene; adhE, alcohol dehydrogenase gene; dhaKLM, dihydroxyacetone kinase; dld, respiratory D-lactate dehydrogenase gene; frdABCD, fumarate reductase gene; fumABC, three isoenzymes of fumarases; gldA, glycerol dehydrogenase; glpD, aerobic glycerol-3-phosphate dehydrogenase; glpK, glycerol kinase; ldhA, fermentative D-lactate dehydrogenase gene; mdh, malate dehydrogenase; pdh, pyruvate dehydrogenase complex; pflB, pyruvate formate lyase gene; poxB, pyruvate oxidase gene; pps, PEP synthase gene; pta, phosphotransacetylase gene; pykA/pykF, pyruvate kinase. Abbreviations: DHAP, dihydroxyacetone phosphate; PEP, phosphoenolpyruvate. Red lines denote overexpression of ldhA, and red cross bars denote the disruption of indicated genes. | |
Recently, we isolated a wild-type E. coli B0013 strain based on its ability to grow quickly, and engineered it for overproducing D-lactate from glucose via elimination of byproduct-forming pathways as indicated in Fig. 1. The resulting strain produced 125 g L−1D-lactate with a high chiral purity from glucose and reduced the yields of byproducts such as acetate, succinate, formate, and ethanol significantly.13 The current investigation is aimed at assessing the potential to bioconvert crude glycerol into optically pure D-lactate using a rationally engineered E. coli strain. Biochemical and kinetic considerations related to the growth of engineered strains and the efficiency in D-lactate production from glycerol were considered and discussed. To date, there have been few reports on the direct production of D-lactate using crude glycerol.
Materials and methods
Strain, media and culture conditions
E. coli B0013 is a wild type strain isolated from a natural sample from which all derivatives were created. E. coli B0013-070 (Δack-pta, Δpps, ΔpflB, Δdld, ΔpoxB, ΔadhE, ΔfrdA) was obtained from a previous study.13E. coli B0013-070-pTHldhA (Δack-pta, Δpps, ΔpflB, Δdld, ΔpoxB, ΔadhE, ΔfrdA, pTH-ldhA) and B0013-070-pUCldhA (Δack-pta, Δpps, ΔpflB, Δdld, ΔpoxB, ΔadhE, ΔfrdA, pUC-ldhA) were constructed in this study. Both strains were stored at the CICIM-CU (Culture and Information Center of Industrial Microorganism of China Universities at Jiangnan University, http://CICIM-CU.jiangnan.edu.cn).
All pre-cultures were grown in LB medium. Modified M9 medium containing glycerol was used as the fermentation medium for lactate production. Modified M9 medium14 contained (per liter): 15.11 g Na2HPO4·12H2O, 3 g KH2PO4, 1 g NH4Cl, 0.5 g NaCl to which a 0.1% MgSO4 solution (1 M) and a 0.1% trace metal stock solution were added. The trace metal stock solution contained (per liter): 2.4 g FeCl3·6H2O, 0.3 g CoCl2·6H2O, 0.15 g CuCl2·2H2O, 0.3 g ZnCl2, 0.3 g Na2MoO4·2H2O, 0.075 g H3BO3, 0.495 g MnCl2·4H2O. Both LB medium and modified M9 medium were sterilized at 121 °C for 20 min. The trace metal stock solution was filter-sterilized through a 0.22 μm pore-size filter.
Genetic manipulations
Standard molecular biology protocols were used for DNA manipulation unless otherwise specifically mentioned.24 The ldhA gene encoding the D-lactate dehydrogenase (LDH) was cloned from the genomic DNA of E. coli B0013 using PCR amplification and the primers 5′-CCCAAGCTTGGGCAGCCCGAGCGTCATCAG-3′ and 5′-CCCAAGCTTCTTGAATTCAAGCTTGCTGCCGGAAATCATCATTTTTT-3′. The resulting PCR fragment, which includes the promoter and coding frame of the ldhA gene, was inserted into the SmaI site of the high-copy vector pUC19 (TaKara, China) to generate the recombinant plasmid pUC-ldhA. The ldhA fragment was obtained by digesting pUC-ldhA using the restriction enzyme HindIII and was inserted into the corresponding site of the low-copy vector pTH18kr25 to create pTH-ldhA. The recombinant plasmids were transformed into competent E. coli B0013-070 cells by electroporation to generate the engineered strains E. coli B0013-070-pTHldhA and E. coli B0013-070-pUCldhA, respectively. The resulting strains were used to investigate the effect of an increased copy number of ldhA on microbial growth and fermentation properties.
Measurement of D-lactate dehydrogenase activity
Cells from microaerobic cultures were treated as previously reported.14 Cells were harvested by centrifugation (2 min, 10
000g), washed twice with 9 g L−1 NaCl, and stored as cell pellets at −20 °C. Cell pellets were resuspended in 0.1 M potassium phosphate buffer to obtain ∼3.4 g dry cell weight (DCW) L−1 and were permeabilized with chloroform. Crude cell extracts were prepared using the bacterial soluble total protein preparation kit (GENMED Scientifics Inc., USA). The extracts were assayed for LDH activity using a kit to colorimetrically determine the total bacterial LDH activity (GENMED Scientifics Inc., USA). One unit of the overall LDH activity was defined as the amount of enzyme required to transform 1 nmol of pyruvate per minute. The protein concentration in the crude extracts was determined by the Bradford method,26 and bovine serum albumin was used as the standard.
Shake-flask experiments
Cells were pre-cultured in 100 mL LB medium in a 250 mL flask. After 10 h of growth with shaking at 37 °C, cells were harvested by centrifugation and resuspended in modified M9 medium. This suspension was used to inoculate 50 mL fresh modified M9 medium containing glycerol at a concentration of 0.02 g L−1 DCW. A two-phase fermentation with an aerobic cell growth phase, followed by an oxygen limited lactate production phase was initiated. During the cell growth phase, all flasks were incubated at 37 °C and 200 rpm except where indicated. When the cell concentration reached 1.37 g L−1 DCW, 1.5 mL of 500 g L−1 glucose and 2 g CaCO3 were added to the broth, and the flasks were kept stationary at 37 °C to start the oxygen-limited phase. Fermentations ceased when glycerol was depleted. When the fed-batch process was conducted, an appropriate volume of autoclaved crude glycerol was added at the indicated time point.
Bioreactor experiments
A modified two-phase fed-batch process was used that was similar to that reported by Zhu et al.,27 except that the lactate production phase generated by nitrogen sparging was not applied. For each bioreactor experiment, cells were pre-cultured as described above and transferred to 150 mL fresh modified M9 medium containing 5 g L−1 glycerol (in a 500 mL flask) at a concentration of 0.1 g L−1 DCW. After incubation for 9 h, this seed culture was used to inoculate a 7 L bioreactor (New Brunswick Scientific Co., USA) containing 4 L modified M9 medium with 20 g L−1 glycerol at a concentration of 0.06 g L−1 DCW. Growth was initiated by sparging air into the bioreactor at 3 to 7 L min−1 and the dissolved oxygen concentration was maintained above 30% saturation by agitation at 200 to 1000 rpm. The pH was maintained at 7 by automatically feeding concentrated NH4OH and 10% (vol/vol) H2SO4. The microaerobic phase started when the cell concentration reached about 26 OD600 (∼10 g L−1 DCW). During the lactate formation phase, the dissolved oxygen tension (DOT) was controlled under 1% saturation by controlling the air flow at 3 L min−1 and agitation at 400 rpm and the pH was maintained at 7 using 25% (wt/vol) Ca(OH)2. Glycerol was added at an initial concentration of 30 g L−1 during the aerobic phase and diluted crude glycerol at a concentration of 600 g L−1 was automatically fed into the bioreactor to maintain the concentration above 10 g L−1 during the lactate formation phase. The two-phase fermentation stopped when glycerol was exhausted (unless otherwise stated), at which time the final product concentrations were measured. During the process, the temperatures of cell growth and D-lactate production were set at 34 °C and 40 °C (unless otherwise stated), respectively.
Source of crude glycerol
Crude glycerol was derived from alkali-catalyzed transesterification of oil-bearing biomass which was used as the substrate for lactate production. The composition of crude glycerol was assayed according to ref. 10 and 17. After dilution with water and filtration, glycerol and methanol concentrations were measured by HPLC according to the methods described below. The free fatty acid content was estimated by adjusting the pH of crude glycerol to 1.0 using HCl and centrifuged at 4000g for 20 min after which fatty acids were carefully removed from the top layer and weighed.10 Ash content was determined by heating the sample at 550 °C overnight in a furnace (Fenton, UK) before the ash weight was determined.17
Analytical methods
Optical density during cell growth was measured at 600 nm. A standard curve relating DCW to OD was constructed (1 OD600 = 0.38 g L−1 DCW). Samples were pretreated with H2SO4 (at 5% of the sample volume), to release organic acids precipitated with CaCO3 or Ca(OH)2 during fermentation. After centrifugation, the supernatant was stored at −20 °C for subsequent HPLC analysis. To quantify the concentrations of glycerol, lactate, acetate, formate, succinate, and ethanol, supernatants were analyzed by ion-exclusion HPLC using a Shimadzu Prominence SIL 20 system (Shimadzu Scientific Instruments, Inc., Columbia, MD) equipped with UV (210 nm) and refractive index detectors, using an Agilent Hi-Plex H, 7.7 × 300 mm, 8 μm (p/n PL1170-6830) with 0.01 M H2SO4 as an eluent (0.6 mL min−1; 50 °C). A RI detector and a UV detector (210 nm) were used for the detection of glycerol and organic acids, respectively. Lactate enantiomeric excess (isomeric purity) was measured by HPLC using a chiral column (CLC-L; Advanced Separation Technologies Inc., Whippany, NJ, USA), at room temperature, equilibrated with 1 mL min−1 of 5 mM CuSO4 as the mobile phase and detected at 254 nm with a UV detector.
Results and discussion
Analysis of the composition of crude glycerol
Previous studies have indicated that the chemical composition of crude glycerol samples varied extensively based on the composition of the oil feedstock, the type of catalyst used to produce biodiesel, the transesterification efficiency, the recovery efficiency of the biodiesel, and the biodiesel production processes.2,10 Thus, in this study the composition of crude glycerol derived from oil-bearing biomass was characterized. After being sterilized by autoclaving, the treated crude glycerol sample contained 63.5% (w/w) glycerol, 24.2% water, 1.9% fatty acids and 2.8% ash (Table S1†). The results show a low glycerol concentration and a high water content, which may favor cell growth and downstream treatment. Methanol was undetectable after autoclaving, as has been reported previously.17
Effect of crude glycerol on the growth of engineered E. coli strains
Previously we constructed an overproducing D-lactate engineered strain of E. coli B0013-070 by deletion of byproduct-forming pathways including genes coding for acetate kinase (ackA), phosphotransacetylase (pta), phosphoenolpyruvate synthase (pps), pyruvate formate lyase (pflB), FAD-binding D-lactate dehydrogenase (dld), pyruvate oxidase (poxB), alcohol dehydrogenase (adhE) and fumarate reductase (frdA), as indicated in Fig. 1. When glucose was used as the substrate, the resulting strain produced 125 g L−1D-lactate with an increased oxygen limiting lactate productivity of 0.61 g g−1 h−1 (2.1-fold greater than E. coli B0013) and significantly reduced yields of byproducts such as acetate, succinate, formate, and ethanol.13 Therefore, it stands to reason that this engineered strain be used to evaluate its feasibility to synthesize D-lactate from crude glycerol.
It has previously been reported that various kinds of impurities in crude glycerol samples have a negative impact on bacterial growth and the bioconversion.11 In order to assess the effect of crude glycerol on the growth performance of E. coli B0013 and B0013-070 strains, a series of minimal media containing various concentrations of crude glycerol was used to culture E. coli in shake flasks, with pure glycerol being the control carbon source for cell growth. There was no discernible difference in the growth of wild type and engineered strains when cultured at concentrations below 40 g L−1 of either crude (40 g L−1 of crude glycerol indicates the final glycerol concentration in the medium containing crude glycerol which has 63.5% glycerol, the same as below) or pure glycerol (Fig. S1†). However, when the crude glycerol concentration increased to 60 g L−1 and 80 g L−1, cell growth was inhibited significantly and biomass content decreased to 3.2 g L−1 and 1.3 g L−1, respectively (Fig. S1a†). Similarly, a high concentration of pure glycerol exhibited little suppressive impact on both the growth and the biomass content of both strains (Fig. S1b†). Moreover, the glycerol consumption rate declined significantly when cultured in high concentrations of glycerol (data not shown). Fig. S1† elucidated that both wild type and engineered strains can efficiently utilize glycerol for cell growth in the crude samples; however, the biomass productivity of strains was inferior to that achieved with pure glycerol under higher substrate concentration. Presumably, the high quantities of salt in crude glycerol had a negative impact on the bacterial growth. Generally, noticeable salt concentrations can inhibit bacteria growth, whereas high salt concentrations can impose cell contraction and eventually osmotic plasmolysis, causing the microorganisms to shrink and die.18,19
Evaluation of the engineered strain B0013-070 for the capability to produce D-lactate from crude glycerol
Higher cell density cultures are directly related to higher volumetric productivity, which is a major objective of any E. coli-based process.20 On the other hand, efficient D-lactate synthesis needs microaerobic or anaerobic conditions during the cultivation of E. coli. Thus, we firstly addressed this issue by manipulation of the initial glycerol concentration and fermentation conditions in a shake flask. Considering that higher concentrations of glycerol suppressed cell growth and substrate consumption, batch fermentations and fed-batch processes with different strains were carried out to investigate the efficiency of lactate production from glycerol. Fig. 2 and Table 1 present a comparison of the fermentation performances of wild type and engineered strains in defined media containing crude or pure glycerol, respectively. In a batch fermentation containing 20 g L−1 of crude glycerol, the wild type strain B0013 consumed glycerol producing D-lactate, acetic acid, succinic acid and ethanol as the main primary products. Comparatively, the engineered strain B0013-070 converted crude glycerol into significantly more D-lactate and all byproducts were observed at low concentrations (Fig. 2 and Table 1). Using 20.1 g L−1 of crude glycerol, B0013-070 produced 13.6 g L−1D-lactate (0.38 g L−1 h−1) at a yield of 0.67 g D-lactate per g glycerol (Fig. 2 and Table 1). However, a higher concentration of D-lactate (14.4 g L−1) with a yield of 0.76 g D-lactate per g glycerol was obtained when pure glycerol was used as the substrate (Fig. 2). Furthermore, a slight increase in cell mass and glycerol consumption was also observed in batch fermentations using pure glycerol as the substrate compared to crude glycerol (Fig. 2), which indicated that some unknown compounds in crude glycerol had a trivial effect on the fermentation process.
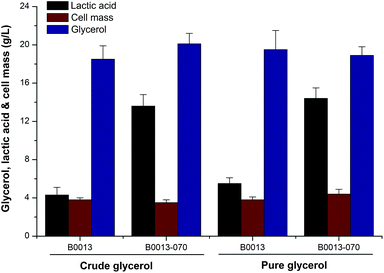 |
| Fig. 2
D-Lactate production, cell growth, and glycerol utilization in 36 hour shake flask cultures of wild-type E. coli B0013 and the engineered strain E. coli B0013-070 in crude glycerol-medium and/or pure glycerol-medium. Error bars represent standard deviations for triplicate measurements. | |
Table 1 Glycerol consumption, product synthesis, and yield of cell mass and product during the two phases of aerobic–microaerobic fermentation by wild-type and engineered strains in the defined medium containing crude glycerola
Strain |
Glycerol consumed (g L−1) |
DCW (g L−1) |
Lactate (g L−1) |
Succinic acid (g L−1) |
Acetic acid (g L−1) |
Ethanol (g L−1) |
Lactate yield (g g−1 glycerol) |
Data represent the averages of three samples (mean ± standard deviations) taken from 36 hour shake flask cultures grown on minimal medium supplemented with 20 g L−1 of crude glycerol, unless otherwise specified.
Cultures to which 40 g L−1 of crude glycerol was used and samples were taken at 60 hours (99% glycerol was consumed).
Culture to which 60 g L−1 of crude glycerol (40 g L−1 initially present and 20 g L−1 added at 48 hours) was used and samples were taken at 80 hours (∼59 g L−1 of glycerol was consumed).
|
Wild-type B0013 |
19.6 ± 1.0 |
3.8 ± 0.3 |
5.3 ± 0.4 |
2.7 ± 0.1 |
3.1 ± 0.3 |
1.2 ± 0.1 |
0.27 ± 0.06 |
B0013-070 |
20.1 ± 1.2 |
3.5 ± 0.1 |
13.6 ± 1.3 |
0.6 ± 0.1 |
1.1 ± 0.2 |
0.5 ± 0.2 |
0.68 ± 0.14 |
B0013-070b |
39.6 ± 0.7 |
3.2 ± 0.1 |
27.4 ± 0.9 |
0.4 ± 0.0 |
0.6 ± 0.2 |
0.6 ± 0.1 |
0.69 ± 0.1 |
B0013-070c |
58.6 ± 2.7 |
2.9 ± 0.2 |
41.2 ± 0.9 |
0.3 ± 0.0 |
1.1 ± 0.1 |
0.5 ± 0.0 |
0.70 ± 0.14 |
Given these results, we further examined the production of D-lactate by the B0013-070 strain in the presence of a higher concentration of glycerol. The strain B0013-070 produced about 27 g L−1 and 41.2 g L−1 of D-lactate from 39 and 59 g L−1 of crude glycerol in about 60 h and 80 h, respectively (Table 1). However, it was noted that the fed-batch process had an impact on cell activity and viability and decreased the productivity of D-lactate because of a long fermentation period in a shake flask. Results indicated that the engineered strain E. coli B0013-070 could convert glycerol into D-lactate with a low concentration of byproducts; however, the conversion efficiency was lower than that when glucose was used as the substrate.13 It may need further engineering to improve the strain's performance.
Effect of overexpressing D-lactate dehydrogenase on the strain's fermentation performance
D-Lactate dehydrogenase (LDH, encoded by ldhA gene) catalyzes pyruvate to D-lactate with an irreversible reaction. It is likely that overexpressing ldhA improved lactate synthesis in E. coli.9 However, high activity of D-lactate dehydrogenase leads to lactate accumulation and inhibits cell growth during the earlier phase of cell growth.21,22 Therefore, we constructed various recombinant plasmids containing ldhA gene of pTH18kr-ldhA (a low-copy vector) and pUC-ldhA (a high-copy vector) (Fig. S2†), which were transformed into E. coli B0013-070 to generate strains E. coli B0013-070-pTHldhA and E. coli B0013-070-pUCldhA, respectively. The resulting strains were evaluated for the capability to produce D-lactate from glycerol. Fig. 3 showed the impact of amplifying the LDH pathway on cell growth and product synthesis. Results showed that overexpressing the ldhA gene using a low-copy vector led to an increase of the concentration and yield of D-lactate, in which 14.5 g L−1 of lactate with a yield of 0.72 g g−1 crude glycerol was obtained (Fig. 3b). However, the growth properties and final cell concentration were similar to that of E. coli B0013-070 (Fig. 3a). Increasing the expression of the ldhA gene in E. coli B0013-070-pUCldhA significantly decreased the cell growth rate with a lower final cell mass (Fig. 3a). Although the final concentration of D-lactate increased (14.7 g L−1 of lactate was produced after a 50 h fermentation period, data not shown), the overall productivity was impeded significantly due to prolonging of the fermentation period (Fig. 3b) and slower glycerol consumption was observed (data not shown). Furthermore, we found that E. coli B0013-070-pUCldhA produced a higher amount of lactate within the earlier phase of growth, which would not only compete for more substrate for cell growth but also depress the growth performance because of the lower pH of the medium. We found that both engineered strains harboring recombinant plasmids produced small amounts of acetate and succinic acid as byproducts in the culture medium (data not shown), similar to E. coli B0013-070 (Table 1). Moreover, LDH activity was assayed and results showed that a 6.5 and 15.1-fold increase in the activity of LDH was obtained from E. coli B0013-070-pTHldhA and E. coli B0013-070-pUCldhA, respectively (Fig. S3†). The observed increases in D-lactate yield and concentration were arguably caused by an increase in LDH activity. Results however indicated that a moderate increase in LDH activity benefits D-lactate production and overexpressing the ldhA gene with a high-copy vector would lead to a negative impact on the fermentation performance of the engineered strain.
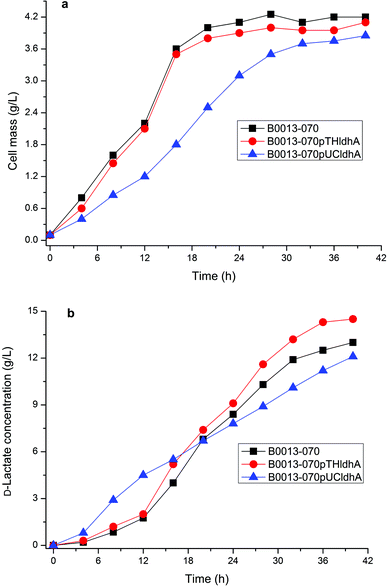 |
| Fig. 3 Evaluation of overexpression of D-lactate dehydrogenase on cell growth and D-lactate synthesis in engineered strains. (a) Growth curves of strains B0013-070, B0013-070-pUCldhA and B0013-070-pTHldhA. (b) Production of D-lactate over time by strains B0013-070, B0013-070-pUCldhA and B0013-070-pTHldhA. Three experiments were carried out and the average values are presented. | |
In addition, previous studies have indicated that overexpression of the enzymes involved in the conversion of glycerol to glycolytic intermediates of DHAP (GlpK-GlpD and GldA-DHAK pathways) can improve the activity of two primary glycerol transport routes and cause a modest increase in D-lactate concentration and yield.9 It can be observed that it is possible to further improve the lactate fermentation performance by engineering the glycerol assimilation pathway in E. coli.
Effect of temperature on growth and D-lactate accumulation by engineered strains
It has been reported that the formation of the desired product during the cell growth phase competes for substrates with biomass formation or inhibits cell growth directly, which results in a decrease in production efficiency.14 Bunch et al.23 found that overexpressing fermentative D-lactate dehydrogenase (LDH, encoded by the ldhA gene) to overproduce D-lactate in E. coli resulted in very poor growth because converting the larger pyruvate pool to lactate by overexpression of LDH resulted in a shortage of 3-carbon metabolic intermediates. Previously, we have developed a two-phase fermentation process to attain a high cell density and intensified production of D-lactate when glucose was used as the substrate.13 However, a certain amount of lactate was still produced during the aerobic growth phase and suppressed the high cell density. A similar pattern of cell growth and D-lactate accumulation in the aerobic phase was observed when using crude glycerol as the substrate (data not shown). Therefore the effect of temperature in relation to cell growth and D-lactate production of the strain B0013-070-pTHldhA was investigated.
As indicated in Fig. 4, the engineered E. coli strain had different growth rates and accumulated different amounts of lactate during different fermentation temperatures in the cell growth phase. At temperatures of 34 °C and 37 °C a similar growth pattern with a higher growth rate and final cell mass was observed (Fig. 4a). However, at higher temperatures (42 °C and/or 45 °C) there was an obvious inhibition of cell growth (Fig. 4a). On the other hand, the production of D-lactate exhibited a different trend as compared to the growth pattern (Fig. 4b). A yield of 0.42 g g−1 glycerol was reached at a temperature of 40 °C, which was higher when compared to other temperatures. Increases in temperature decreased the yield, and 0.16 g g−1 glycerol and 0.11 g g−1 glycerol of yields were obtained at 42 °C and 45 °C, respectively. The effect of temperature on D-lactate production in the micro-aerobic phase was evaluated and the results are shown in Fig. 5. After the cultures reached a high cell density in the aerobic phase at 34 °C following 12 h of incubation, the temperature for D-lactate production was optimized. Fig. 5(b) showed that temperatures of 40 °C and 42 °C led to a higher D-lactate concentration, and 22.5 g L−1 and 22.1 g L−1D-lactate from 40 g L−1 glycerol were obtained under shake flask batch fermentations, respectively; however, the cell mass decreased slightly at temperatures above 42 °C compared to temperatures below 40 °C (Fig. 5a). Higher temperature impaired both the cell mass and the accumulation of the desired product (Fig. 5a and b). Therefore, there is reason to speculate that the two-phase process combined with a temperature-shifting strategy can improve D-lactate production significantly under bioreactor conditions.
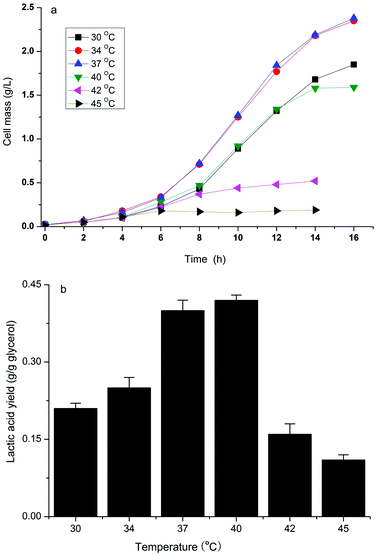 |
| Fig. 4 Cell growth pattern (a) and D-lactate accumulation (b) of the strain B0013-070-pTHldhA during the aerobic growth phase on shake flask incubation with different temperatures. Values are reported as averages of triplicate assays (a) and error bars represent standard deviations for triplicate measurements (b). | |
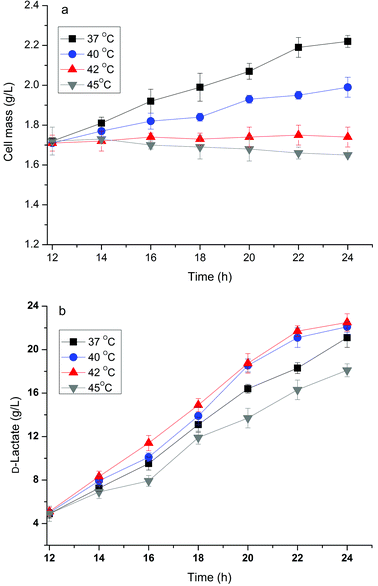 |
| Fig. 5 Cell mass accumulation (a) and D-lactate production (b) of the B0013-070-pTHldhA strain during the microaerobic fermentation phase under different temperatures. Error bars represent standard deviations for triplicate measurements. | |
Enhanced production of D-lactate from crude glycerol in a bioreactor
The above results indicated that the engineered strain B0013-070-pTHldhA performed well when crude glycerol was used as a carbon source, better than the wild type and B0013-070 strains. Experimentally, it is difficult to accurately control the fermentation parameters such as dissolved oxygen and pH in a shake flask. Therefore, a scale-up fermentation using the B0013-070-pTHldhA strain with a 7 L bioreactor was employed to better assess the potential for D-lactate production of this engineered strain. The two-phase fermentation combined with a temperature shifting process was carried out under controlled conditions. Cell mass reached a maximum at ∼10 h but lactate accumulation was very low which indicated that oxygen supply in the aerobic phase was sufficient in the bioreactor compared to the shake-flask fermentation (Fig. 6). Furthermore, the optimal growth temperature also significantly reduced the lag period of the engineered strain (Fig. 6). When the lactate formation phase was shifted by controlling the agitation and aeration rates, at which point the temperature was raised from 34 °C to 40 °C, lactate production increased at a high specific rate and the final concentration reached 100.3 g L−1 with an optical purity of 99.97% (Fig. 6). After a 36 h fermentation period, 531.5 g of the total crude glycerol was consumed with an overall lactate productivity of 2.78 g L−1 h−1 and an overall yield of 75.4 g per 100 g glycerol (Table 2), which indicated a significant increase in lactate productivity and concentration in the bioreactor experiment. When a constant temperature of 37 °C was maintained during the overall process, a lower yield of 69.4 g per 100 g glycerol and a lower productivity of 2.62 g−1 L−1 h−1 were observed for D-lactate production compared to the temperature-shifting process (Table 2), with the final concentration of D-lactate reaching only 93.3 g L−1. Pure glycerol was also used to compare the potential and efficiency of lactate production from biodiesel-based crude glycerol. Using similar conditions to the temperature-shifting strategy, the engineered strain showed an increase in the concentration, yield and productivity of D-lactate, which reached 111.7 g L−1, 3.10 g L−1 h−1 and 80.4 g per 100 g glycerol, respectively. It can be deduced that crude glycerol is suitable to be used as a cheap substrate for D-lactate production using a metabolically engineered strain of E. coli; however, unknown impurities in the sample did have minor effects on the efficiency of bioconversion to D-lactate.
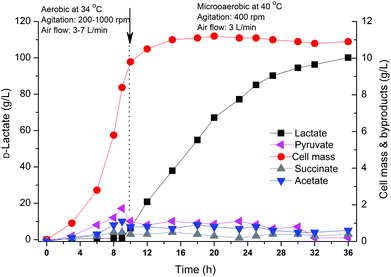 |
| Fig. 6 Representative kinetics of D-lactate production by the engineered strain B0013-070-pTHldhA in a bioreactor with the aerobic and microaerobic fermentation processes combined with the temperature shifting strategy. The arrow indicates the time point at which the culture was switched from aerobic cultivation at 34 °C to the microaerobic production phase at 40 °C. An initial concentration of 20 g L−1 of crude glycerol was used and a high concentration of the substrate was automatically fed into the bioreactor to maintain the concentration above 10 g L−1 during the microaerobic phase. Coefficients of variation (i.e., standard deviations/average × 100) were below 5% in all cases. | |
Table 2 Comparison of fermentation data of B0013-070-pTHldhA (mean ± range of duplicate experiments) in bioreactor experiments from pure glycerol and crude glycerol after a period of 36 h
Fermentation processa |
Glycerol consumedb (g) |
Lactate concentration (g L−1) |
Lactate volumetric productivityc (g L−1 h−1) |
Yield of the overall fermentation process (g per 100 g glycerol) |
Cell mass |
Lactate |
Acetate |
Succinate |
Pyruvate |
37 °C indicated that the overall fermentation temperature was constantly set at 37 °C, 34 °C/40 °C indicated that the aerobic incubation temperature was set at 34 °C and the microaerobic phase was set at 40 °C, and crude glycerol or pure glycerol was used as a carbon source.
Indicated total glycerol consumed during the overall fermentation process.
Average volumetric productivity for lactate during the overall fermentation process.
|
34 °C/40 °C and crude glycerol |
531.5 ± 4.1 |
100.3 ± 4.1 |
2.78 ± 0.3 |
8.4 ± 0.5 |
75.4 ± 4.4 |
0.5 ± 0.1 |
0.3 ± 0.1 |
0.2 ± 0.0 |
37 °C and crude glycerol |
538.2 ± 15.7 |
93.3 ± 5.7 |
2.62 ± 0.1 |
8.9 ± 0.4 |
69.4 ± 4.1 |
0.6 ± 0.2 |
0.5 ± 0.2 |
0.0 ± 0.0 |
34 °C/40 °C and pure glycerol |
555.6 ± 16.5 |
111.7 ± 9.6 |
3.10 ± 0.2 |
9.0 ± 0.3 |
80.4 ± 5.5 |
0.4 ± 0.0 |
0.1 ± 0.0 |
0.1 ± 0.0 |
37 °C and pure glycerol |
568.1 ± 15.2 |
106.9 ± 6.0 |
2.97 ± 0.2 |
9.3 ± 0.6 |
75.3 ± 4.8 |
0.5 ± 0.1 |
0.4 ± 0.1 |
0.2 ± 0.0 |
It should be noted that the primary costs associated with lactate production from crude glycerol depends on not only D-lactate yield and productivity but also the downstream D-lactate recovery and purification processes. Conventional purification processes such as crystallizing, filtering, dissolving, and subsequently acidifying were performed for the isolation of lactic acid from the fermentation broth. However, huge quantities of calcium sulfate cake were generated as waste.28 Recently, some novel purification processes such as adsorption, electrodialysis, reactive extraction, and reactive distillation were also developed.29 Especially, the electrodialysis process is advantageous in its capability for separation, purification and concentration of salts, and in the conversion of these salts into acids and bases without generating waste effluents containing high concentrations of salts.30 This process might be suitable for broth using crude glycerol as the substrate, which contains high quantities of salt and some unknown impurities. In the future, developing a robust biocatalyst which can directly convert crude glycerol into lactate without prior autoclave could also decrease the price of lactic acid.
Conclusions
It can be concluded that a metabolically engineered strain of E. coli could be efficiently used to produce D-lactate using crude glycerol as a sole carbon source. Impurities in the sample had a minor impact on the fermentation performance. A combination of a two-phase fermentation process and a temperature-shifting strategy using crude glycerol improved D-lactate production significantly, achieving 100.3 g L−1D-lactate with an overall lactate productivity of 2.78 g L−1 h−1 and a yield of 0.75 g g−1 glycerol (0.77 mol mol−1) from the crude sample. In summary, our study shows that the crude glycerol could be directly converted to D-lactate with high efficiency.
Acknowledgements
This work was financially supported by the National Natural Science Foundation of China (no. 21006039), the Jiangsu province science and technology support program (BE2012618), the 111 Project (no. 111-2-06) and the Jiangsu province “Collaborative Innovation Center for Advanced Industrial Fermentation” industry development program.
References
- P. J. Westfall and T. S. Gardner, Curr. Opin. Biotechnol., 2011, 22, 344–350 CrossRef CAS PubMed.
- F. Yang, M. A. Hanna and R. Sun, Biotechnol. Biofuels, 2012, 5, 13 CrossRef CAS PubMed.
- J. A. Posada, L. E. Rincon and C. A. Cardona, Bioresour. Technol., 2012, 111, 282–293 CAS.
- M. L. Barbelli, G. F. Santori and N. N. Nichio, Bioresour. Technol., 2012, 111, 500–503 CAS.
- A. A. Koutinas, R. H. Wang and C. Webb, Biofuels Bioprod. Bior., 2007, 1, 24–38 CrossRef CAS.
- X. Tang, Y. Tan, H. Zhu, K. Zhao and W. Shen, Appl. Environ. Microbiol., 2009, 75, 1628–1634 CrossRef CAS PubMed.
- B. Andreessen, A. B. Lange, H. Robenek and A. Steinbuchel, Appl. Environ. Microbiol., 2010, 76, 622–626 CrossRef CAS PubMed.
- M. D. Blankschien, J. M. Clomburg and R. Gonzalez, Metab. Eng., 2010, 12, 409–419 CrossRef CAS PubMed.
- S. Mazumdar, J. M. Clomburg and R. Gonzalez, Appl. Environ. Microbiol., 2010, 76, 4327–4336 CrossRef CAS PubMed.
- Y. Liu, C. M. Koh and L. Ji, Bioresour. Technol., 2011, 102, 3927–3933 CrossRef CAS PubMed.
- A. Chatzifragkou, D. Dietz, M. Komaitis, A. P. Zeng and S. Papanikolaou, Biotechnol. Bioeng., 2010, 107, 76–84 CrossRef CAS PubMed.
- C. Gao, C. Ma and P. Xu, Biotechnol. Adv., 2011, 29, 930–939 CrossRef CAS PubMed.
- L. Zhou, Z. R. Zuo, X. Z. Chen, D. D. Niu, K. M. Tian, B. A. Prior, W. Shen, G. Y. Shi, S. Singh and Z. X. Wang, Curr. Microbiol., 2011, 62, 981–989 CrossRef CAS PubMed.
- L. Zhou, D. D. Niu, K. M. Tian, X. Z. Chen, B. A. Prior, W. Shen, G. Y. Shi, S. Singh and Z. X. Wang, Metab. Eng., 2012, 14, 560–568 CrossRef CAS PubMed.
- A. Murarka, Y. Dharmadi, S. S. Yazdani and R. Gonzalez, Appl. Environ. Microbiol., 2008, 74, 1124–1135 CrossRef CAS PubMed.
- S. Mazumdar, M. D. Blankschien, J. M. Clomburg and R. Gonzalez, Microb. Cell. Fact., 2013, 12, 7 CrossRef CAS PubMed.
- D. J. Pyle, R. A. Garcia and Z. Y. Wen, J. Agric. Food Chem., 2008, 56, 3933–3939 CrossRef CAS PubMed.
- A. L. Koch, J. Bacteriol., 1984, 159, 919–924 CAS.
- R. E. Marquis, J. Bacteriol., 1968, 95, 775–781 CAS.
- J. Shiloach and R. Fass, Biotechnol. Adv., 2005, 23, 345–357 CrossRef CAS PubMed.
- L. Zhou, K. M. Tian, D. D. Niu, W. Shen, G. Y. Shi, S. Singh and Z. X. Wang, Biotechnol. Lett., 2012, 34, 1123–1130 CrossRef CAS PubMed.
- L. Zhou, W. Shen, D. D. Niu, K. M. Tian, B. A. Prior, G. Y. Shi, S. Singh and Z. X. Wang, J. Ind. Microbiol. Biotechnol., 2012, 39, 1209–1217 CrossRef CAS PubMed.
- P. K. Bunch, F. Mat-Jan, N. Lee and D. P. Clark, Microbiology, 1997, 143(Pt 1), 187–195 CrossRef CAS PubMed.
-
J. Sambrook, E. F. Fritsch and T. Maniatis, Molecular Cloning. A Laboratory Manual, Cold Spring Harbor Laboratory Press, Cold Spring Harbor, NY, 1989 Search PubMed.
- T. Hashimoto-Gotoh, M. Yamaguchi, K. Yasojima, A. Tsujimura, Y. Wakabayashi and Y. Watanabe, Gene, 2000, 241, 185–191 CrossRef CAS.
- M. M. Bradford, Anal. Biochem., 1976, 72, 248–254 CrossRef CAS.
- Y. Zhu, M. Eiteman, K. DeWitt and E. Altman, Appl. Environ. Microbiol., 2007, 73, 456–464 CrossRef CAS PubMed.
- H. G. Joglekar, I. Rahman, S. Babu, B. D. Kulkarni and A. Joshi, Sep. Purif. Technol., 2006, 52, 1–17 CrossRef CAS PubMed.
- J. Vijayakumar, R. Aravindan and T. Viruthagiri, Chem. Biochem. Eng. Q., 2008, 22, 245–264 CAS.
- M. A. Abdel-Rahman, Y. Tashiro and K. Sonomoto, Biotechnol. Adv., 2013, 31, 877–902 CrossRef CAS PubMed.
Footnotes |
† Electronic supplementary information (ESI) available. See DOI: 10.1039/c3gc41769g |
‡ These authors contributed equally to this work. |
|
This journal is © The Royal Society of Chemistry 2014 |
Click here to see how this site uses Cookies. View our privacy policy here.