DOI:
10.1039/C2FO30216K
(Review Article)
Food Funct., 2013,
4, 40-51
Mechanisms and prospects of food protein hydrolysates and peptide-induced hypolipidaemia
Received
23rd August 2012
, Accepted 19th October 2012
First published on 22nd October 2012
Abstract
Hyperlipidaemia is an important risk factor for developing cardiovascular disease, a leading global health issue. While pharmaceutical interventions have proved efficacious in acute conditions, many hypolipidaemic drugs are known to induce adverse side effects. Due to a strong positive link between functional food components and human health, emerging research has explored the application of natural food-based strategies in disease management. One of such strategies involves the use of food proteins as precursors of peptides with a wide variety of beneficial health functions. Some plant, animal and marine-derived protein hydrolysates and peptides have shown promising hypolipidaemic properties when evaluated in vitro, in cultured mammalian cells and animal models. The products exert their functions via bile acid-binding and disruption of cholesterol micelles in the gastrointestinal tract, and by altering hepatic and adipocytic enzyme activity and gene expression of lipogenic proteins, which can modulate aberrant physiological lipid profiles. The activity of the protein hydrolysates and peptides depends on their physicochemical properties including hydrophobicity of amino acid residues but there is knowledge gap on detailed structure–function relationships and efficacy in hyperlipidaemic human subjects. Based on the prospects, commercial functional food products containing hypolipidaemic peptides have been developed for enhancement of cardiovascular health.
1. Introduction
Cardiovascular disease (CVD) is a leading cause of morbidity and mortality in the world, and elevated blood lipids have been strongly associated with increased incidence of CVD.1 For instance, thoracic and coronary atherosclerosis is associated with unbalanced ratio and concentrations of lipoproteins whereas abdominal atherosclerosis is linked to decreased high-density lipoprotein (HDL) levels.2 Health care expenditures for individuals with dyslipidaemia, as of 2010, were estimated to be $5140 per person per annum compared to $2156 for healthy individuals in the United States.3 Pharmaceutical interventions for lowering endogenous lipids during dyslipidaemia have been effective but a variety of adverse side effects have been associated with hypolipidaemic drugs. For example, the use of statins, commonly prescribed cholesterol-lowering drugs, is associated with various myopathies that may persist even after cessation of treatment.4,5 Moreover, niacin has been associated with skin flushing and impaired glycaemic control.6 Due of these negative effects, there has been an increased emphasis on the discovery of alternative management and treatment approaches devoid of toxicity. Functional foods and nutraceuticals are of particular interest as the connection between food quality and the incidence of chronic diseases has been well established. Particularly, bioactive peptides or biopeptides derived from food proteins have shown promising future for use in the treatment and management of several human health conditions. Many of the peptides exhibit notable ameliorating effects during aberrant physiological conditions, and are generally considered safe for human consumption since they are derived from natural food sources.7 Biopeptides display a wide range of activities including antihypertensive,8 antioxidant,9 and antimicrobial.10 These food-derived peptides are often produced from a variety of proteins derived from plants,11 meats12 and marine organisms.13 This review was aimed at discussing the state of knowledge from the recent past regarding the mechanisms and potential application of food protein hydrolysates and peptides in ameliorating hyperlipidaemia and associated health complications.
2. Lipid lowering bioactive protein hydrolysates and peptides
Important indicators of the potential to develop CVD include elevated and decreased levels of endogenous lipids. Blood lipids can be represented in various forms including lipoproteins (HDL, low-density [LDL] and very low-density [VLDL]), triglycerides (TG), total cholesterol (TC), and free fatty acids (FFA). The aberrations in cardiovascular health are often associated with improper ratios of these lipids. Thus, CVD intervention is often focused on increasing the physiological levels of desirable lipids (e.g. HDL cholesterol) and reducing the others with atherogenic functions (e.g. LDL cholesterol, TG) in order to achieve an optimal lipid balance and positive cardiovascular health. Plasma lipid levels are controlled by the balance between dietary consumption and rates of endogenous synthesis and excretion of the lipids. Bioactive peptides and hydrolysates derived from food protein sources have shown promising functions in modulating endogenous lipid profiles during dyslipidaemia. Based on recent literature, a few hypolipidaemic peptide sequences have been identified from enzymatic food protein hydrolysates of plant, marine and animal origin14–22 with the majority of known sequences reported within the last decade (Table 1).
Table 1 Sequence, sources and mechanisms of action of purified hypolipidaemic peptides derived from food proteins
Protein |
Peptide |
Mechanism of hypolipidaemic function |
Reference |
Soybean glycinin |
VAWWMY (soystatin) |
Displayed bile acid binding, inhibited micellar solubility of cholesterol and inhibited cholesterol absorption and distribution in intestine, serum and liver of rats |
21 and 22
|
Soy β-conglycinin |
FVVNATSN |
Activated transcription of low-density lipoprotein (LDL) receptor (LDL-R) in cultured human hepatic (HepT9A4) cells by over 2 folds compared to control |
15
|
Soybean glycinin |
FKTNDRPSIGN |
Activated transcription of LDL-R in cultured HepT9A4 cells |
15
|
Soybean glycinin |
SSPDIYNPQAGS |
Activated transcription of LDL-R in cultured HepT9A4 cells |
15
|
Soybean glycinin |
DTPMIGT |
Activated transcription of LDL-R in cultured HepT9A4 cells |
15
|
Soybean protein |
WGAPSL |
Potently inhibited micellar cholesterol solubility due to its hydrophobicity |
20
|
Soybean globulin |
IAVPGEVA |
Displayed high bile acid binding activity for cholic and deoxycholic acids |
17
|
Soybean glycinin |
LPYP and LPYPR |
Displayed hypocholesterolemic activity by the inhibition of lipogenic 3-hydroxy-3-methyl-glutaryl-CoA reductase activity |
16
|
Bovine β-lactoglobulin |
IIAEK (lactostatin) |
Reduced cholesterol uptake in cultured Caco-2 cells possibly due to inhibition of cholesterol micellar solubility; binds bile acids; activates 7α-hydroxylase (CYP7A1) via Ca2+-induced signal transduction pathway in HepG2 cells |
18 and 64
|
Bovine β-lactoglobulin |
GLDIQK |
Reduced cholesterol uptake in cultured Caco-2 cells |
18
|
Bovine β-lactoglobulin |
ALPMH |
Reduced cholesterol uptake in cultured Caco-2 cells |
18
|
Bovine β-lactoglobulin |
VYVEELKPTPEGDLEILLQK |
Reduced cholesterol uptake in cultured Caco-2 cells |
18
|
Soybean globulin |
LRVPAGTTFYVVNPDNDENLRMIA |
Increased cellular uptake and degradation of LDL cholesterol mediated by cell surface LDL-R in cultured HepG2 cells |
14
|
Bovine haemoglobin |
VVYP |
Inhibited triglyceride (TG) synthesis by reducing lipid absorption in the enterocytes of mice |
19
|
Soybean β-conglycinin, glycinin, lipoxygenase |
SY |
Inhibited apolipoprotein B secretion and TG synthesis in HepG2 cells |
63
|
Soy glycinin, lipoxygenase, trypsin inhibitor |
VK |
Inhibited TG synthesis in HepG2 cells |
63
|
Soy β-conglycinin, glycinin, lipoxygenase, trypsin inhibitor |
KA |
Inhibited TG synthesis in HepG2 cells |
63
|
Soybean β-conglycinin |
YPFVV (soymorphin-5) |
Reduced plasma and liver TG; increased plasma adiponectin, liver adiponectin receptor expression and PPARα activation with upregulation of target genes involved in fatty acid oxidation and energy expenditure |
69
|
Bovine β-lactoglobulin |
HIRL (β-lactotensin) |
Reduced serum total cholesterol and LDL cholesterol; activity mediated by neurotensin NT2 receptor and possibly bile acid secretion |
72
|
2.1. Hypolipidaemic animal and marine food protein hydrolysates
Major sources of hypolipidaemic peptides include animal and marine food proteins such as chicken collagen, egg white, bovine and fish proteins. Several studies have reported evidence that enzymatic hydrolysates of these proteins can reduce key lipid markers of cardiovascular disease including elevated plasma levels of TC and TG in animal models of hyperlipidaemia.23–29 Some of the effects have often been associated with reduced plasma levels of lipoproteins LDL + VLDL,25,28,29 responsible for delivering cholesterol and TG into cells through associated receptors, as well as elevated amounts of HDL,25,26,28 which transports cholesterol from circulation to the hepatocytes for processing and excretion. Therefore, the above induced lipoprotein pattern is indicative of the potential benefits of animal and marine food protein hydrolysates in cardiovascular disease intervention. Summaries of recent animal and cell culture studies with hypolipidaemic food protein hydrolysates are presented in Tables 2 and 3. Although there are demonstrated hypolipidaemic functions even with long-term consumption,24 reliable comparison of the efficacy of the interventions seems difficult due to marked differences in the reported experimental conditions. For instance, the physiological diversity of the genetic and diet-induced animal models of hyperlipidaemia in Table 2 may impact baseline plasma and hepatic lipid profiles, and subsequent response of the animals to the protein hydrolysate treatments. Other sources of variation include the different dietary doses of the hydrolysates and duration of treatments.
Table 2 Animal studies with hypolipidaemic food protein hydrolysatesa
Hydrolysate |
Animal model |
Treatment, dose and duration |
Study outcome on lipid profiles |
Ref. |
Abbreviations: TC, total cholesterol; TG, triglyceride; HDL, high-density lipoprotein; LDL, low-density lipoprotein; VLDL, very low-density lipoprotein.
|
Animal sources
|
Chicken collagen |
C57BL/6.KOR-ApoEsh1 mice Atherosclerosis |
Diet containing 10% hydrolysate was fed to the animals for 12 weeks |
Plasma TC was reduced by 14% but no effect was observed on HDL or LDL; hepatic TC and TG were reduced by 24% and 42%, respectively |
23
|
Egg white |
Spontaneously hypertensive rats |
0.5 or 1 g hydrolysates per kg body weight per day administered via drinking water over a 20-week period |
Long-term consumption led to decreased plasma TG and TC (−28% and −13%, respectively) and improved oxidative status; no effect was observed on HDL; lower dose of 0.5 g kg−1 did not induce hypolipidaemia in the rats |
24
|
Whole freshwater clam |
Sprague-Dawley rats |
High-cholesterol diet containing 16% hydrolysate was fed to the rats over a 4-week period |
Plasma TG and TC were decreased by 65% and 26%, respectively; HDL was increased by 66% with corresponding 60% reduction of LDL + VLDL; hepatic TG and TC were also decreased with increased faecal excretion of lipids and bile acids; hypolipidaemic effects were better than those of soluble fibre |
25
|
Jellyfish |
Wistar rats |
0.2, 0.4 or 0.8 g hydrolysates per kg body weight per day for 30 days with a high-fat diet |
Dose-dependent hypolipidaemic effects; lowest dose enhanced HDL level (23%); higher doses decreased TG (up to 34%) and the highest dose decreased TC (14%) |
26
|
Sardinelle |
Wistar rats |
High-cholesterol diets containing 5% of three hydrolysates were administered for 7 weeks |
Decreased serum TC, TG and LDL by up to 21%, 46% & 40%, respectively; increased HDL by 22% and improved atherogenic index which indicates hypolipidaemic function |
28
|
Silk |
C57BL/KsJ-db/db mice Obesity and type 2 diabetes model |
0.1 or 0.2 g hydrolysates per kg body weight was administered per day for 4 weeks |
TC and LDL were decreased by 11% and 27%, respectively; no effect was observed on HDL although atherogenic index was reduced; hypolipidaemic function was statistically independent of the amount of administered hydrolysates |
29
|
Bovine heart |
Wistar rats |
High-cholesterol diet containing 11.6% hydrolysates was fed to the rats for 1 week |
Decreased serum TC and LDL + VLDL by 15% and 18%, respectively compared to a casein diet; lowered the animals' atherogenic index by 17% and increased fecal excretion of cholesterol and total steroids; no observed effect on HDL |
52
|
Pork liver |
OLETF rats Obese and type 2 diabetes model |
Diet containing 20% hydrolysates was fed for 14 weeks |
Decreased plasma TG, TC and FFA; no effect on hepatic lipids; hydrolysates also increased fecal excretion of total lipids |
62
|
Plant sources
|
Oats β-glucan/protein |
Wistar rats |
High-fat diet containing 11.6% hydrolysates (with 13% protein component) administered over a 4-week period |
Induced a decrease in serum LDL (31%) and VLDL (23%) and TG; increased HDL (62%) with corresponding improvement of hepatic lipid profile; increased fecal bile acid, cholesterol and TG excretion compared to native protein |
33
|
Whole soybean milk |
C57BL/6N mice |
High-fat and cholesterol diet containing 20% hydrolysates or native protein was fed for 5 weeks |
Decreased plasma and hepatic TG, TC, and plasma FFA; also reduced hepatic lipid droplets and adipocyte size indicating possible anti-obesity effects; hypolipidaemic functions superior to the effects of native proteins |
34
|
Brown/white rice and soy |
Syrian Golden hamster |
High-fat diet containing 20% hydrolysates or native protein were administered for 3 weeks |
Brown rice (BRPH) and soy protein hydrolysates induced a decrease in TC by 8% and 19%, respectively; all hydrolysates decreased VLDL by 23% to 63%; BRPH also induced a decrease in hepatic TC, weight gain, liver weight, and increase in the removal of removal of lipids and bile acids in faeces |
36
|
Soy |
OLETF rats |
Diet containing 19.1% crude hydrolysates or 11.8% hydrophilic fraction fed to rats for 2 and 4 weeks, respectively |
Decreased hepatic and serum TG accumulation; similar activities observed for crude hydrolysates and hydrophilic fraction with lower dose of the latter indicating concentration of bioactive principles after fractionation |
63
|
Table 3 Hypolipidaemic properties of food protein hydrolysates in mammalian cell culturesa
Protein |
Protease |
Cell culture and treatment |
Outcome/mechanism |
Ref. |
Abbreviations: LDL-R, low-density lipoprotein receptor; TG, triglyceride; VLDL, very low-density lipoprotein; ApoB, apolipoprotein B; FAS, fatty acid synthase.
|
Soybean |
Microbial neutrase and alkaline proteases |
Human hepatocytes HepT9A4 treated with up to 1 mg mL−1 hydrolysates for 24 h |
Stimulated the transcription of LDL-R from 126% to 238% compared to control (100%); no observed cytotoxicity due to treatment but cholesterol synthesis was increased via unknown mechanisms |
15 and 65
|
Bovine heart |
Alcalase® |
Human intestinal Caco-2 cells incubated with 5 mg mL−1 hydrolysates |
Enzymatic hydrolysate treatment induced substantial decrease of 14C-cholesterol uptake in the cultured enterocytes compared to casein; not compared with intact bovine heart protein |
52
|
Soybean |
Microbial endo-type protease |
Human hepatocytes HepG2 incubated with 1-10 mg mL−1 crude hydrolysates or hydrophilic fraction for 24 h |
Treatments decreased cellular TG synthesis (14C-acetate incorporation into TG fraction); hydrophilic fraction also induced a decrease in the secretion of ApoB100 (protein component of VLDL) |
63
|
Soybean |
Thermoase, bioprase and sumizyme |
Human hepatocytes HepG2 incubated with 3 and 6 mg mL−1 hydrolysates for 24 h |
Also decreased ApoB100 secretion and TG synthesis (14C-acetate and 3H-glycerol incorporation into TG); decreased cholesteryl ester synthesis and intracellular cholesterol level, but increased cholesterol synthesis in the cells; no cytotoxicity associated with treatment |
68
|
Soybean (different varieties) |
Alcalase® |
Human (pre)adipocytes treated with different concentration of enzymatic hydrolysates |
The different hydrolysates inhibited cellular FAS activity and lipid accumulation and adipogenesis; effects were dependent on the soybean genotype due to variable amounts of protein precursors |
70
|
Furthermore, a number of studies have demonstrated lack of effects of the protein hydrolysate treatments on the lipoproteins,23,24,29 and these observations can be attributed to the amount of active principles and the heterogeneous nature of the peptide constituents, which may have acted via different mechanisms. Protein hydrolysates contain several peptides mostly of uncharacterized structures and unknown relative amounts, and these peptides may interact with physiological disease targets inducing scores of positive effects on aberrant endogenous lipid profile. In order to delineate the functional units and for better understanding of structure–function relationships, there is a need to characterize the active peptides in the complex mixtures; some examples are shown in Table 1. The hypolipidaemic functions can be dependent on the dose of the protein hydrolysates administered to the animals,26 perhaps due to increased amounts of particular active principles, although contradictory observation has been reported.29 The lack of dose–response can be due to saturation of the active peptides within the hydrolysates, which may be resolved by further evaluation with lower amounts. Moreover, some doses (e.g. 0.8 and 1 g per kg body weight) of protein hydrolysates that induced hypolipidaemic effects in the animals24,26 would be considered high if directly translated to humans considering the dietary reference intakes of proteins. In addition, a combination of peptides in protein hydrolysates with other compounds can induce hypolipidaemic functions. This has been demonstrated in Wistar rats that consumed a high-fat diet with fish protein hydrolysate and fish oil resulting in significantly reduced plasma levels of TC and cholesteryl esters, but also reduced HDL level.30 These effects are attributable to interaction of the individual fish-derived components, which lacked substantial hypolipidaemic effects when consumed alone.
In order to validate the potency of food protein hydrolysates and peptides in cardiovascular health promotion, human clinical trials involving the consumption of the products need to be conducted. There is limited literature information on the effects of the protein hydrolysates and peptides on endogenous atherogenic lipid profiles in hyperlipidaemic human subjects. In one study, marine collagen peptides was found to reduce plasma levels of TG, TC, LDL and FFA, and also increased plasma HDL level in human subjects with type 2 diabetes over a 3-month period.31 These observed effects will be considered particularly promising due to the roles of hyperlipidaemia and diabetes in the aetiology and progression of cardiovascular disease. Furthermore, a spread containing dairy antihypertensive lactotripeptides (IPP/VPP) and plant sterols was reported to significantly reduce plasma levels of TC and LDL.32 Although plant sterols possess hypolipidaemic properties, the multifunctional nature of the milk peptide/sterol combination can lead to enhanced cardiovascular health promotion.
2.2. Hypolipidaemic plant protein hydrolysates
In addition to animal and marine proteins, plant proteins have also been reported as sources of hypolipidaemic peptides. The choice of protein source of the functional peptides is often influenced by the need to add value to underutilized proteins, or based on the preponderance of particular structural features that will contribute to bioactivity.7 Plant protein hydrolysate products have been found to induce favourable lipid profiles in hyperlipidaemic animal models with up to 66% increase in serum HDL level.33–36 Although unhydrolyzed plant proteins may exhibit hypolipidaemic functions,33,34 there is evidence of marked difference between the effects of native plant proteins and their enzymatic hydrolysates on endogenous lipid profiles.33 Moreover, endogenous proteases act on ingested proteins to produce peptides that may have exerted the observed hypolipidemic effects. There is also considerable evidence that the modulatory effects of plant protein hydrolysates on hepatic and plasma lipid profiles are associated with anti-obesity activity characterized by decreased fat deposition in adipose tissue of mice.34 Furthermore, a number of studies have reported the production of lipid-lowering peptides and protein hydrolysates from rice.35–37 Recently, an attempt was made to enhance the function of rice protein as a source of bioactive peptides using biotechnological approach. A highly functional hypolipidaemic peptide, lactostatin (IIAEK, originally derived from bovine β-lactoglobulin), was inserted in the C-terminal region of rice glutelin acidic subunit as six tandem repeats of polypeptide with trypsin cleavage sites between the pentapeptides.35 The expressed and isolated lactostatin–glutelin protein fraction was reported to induce substantial decreases in LDL cholesterol, atherogenic index, serum TC and hepatic lipids, and to increase HDL cholesterol in Wistar rats compared to only glutelin, which also showed considerable hypolipidaemic effects. Thus, the overall effects observed in the study could be attributed to the combined activities of lactostatin and rice glutelin. Furthermore, a water-soluble rice bran extract that contained peptides and lipids was found to reduce plasma TC although the observed activity can be partly due to the phytosterol content and not the peptides alone.37 Based on available evidence, the hypolipidaemic activity patterns of plant protein hydrolysates are not particularly distinct from those of their animal and marine source-derived counterparts, even with the presence of other plant bioactives such as fibre, isoflavones and sterols.33,34,37 Therefore, the hypolipidaemic properties of these products seem to depend on the nature of the constituent peptides and not the protein sources. The differential effects exerted by the protein hydrolysates indicate possible different mechanisms of action of the constituent peptides.
3. Proposed mechanisms of hypolipidaemia
The proposed mechanisms of the hypolipidaemic functions of food protein hydrolysates and peptides based on in vitro, cell culture and animal studies are summarized in Fig. 1.
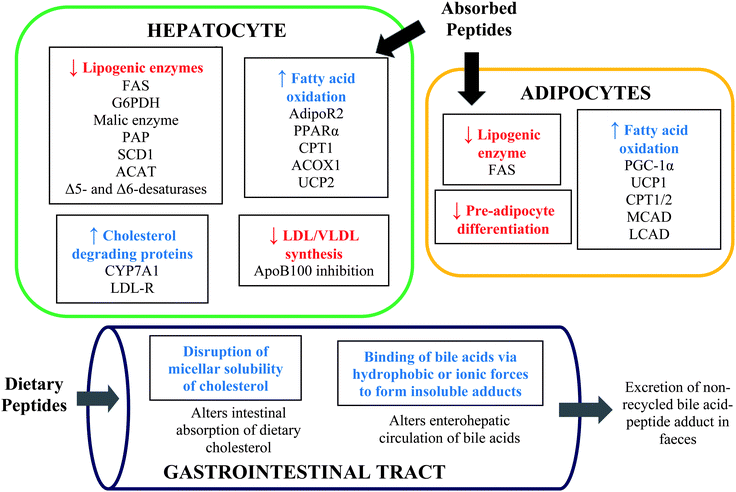 |
| Fig. 1 Proposed mechanisms of the hypolipidaemic functions of food protein hydrolysates and peptides in hepatocytes, adipocytes and intestinal tract. FAS, fatty acid synthase; G6PDH, glucose 6-phospahte dehydrogenase; PAP, phosphatidate phosphohydrolase; SCD, stearoyl-CoA desaturase; ACAT, acyl-CoA cholesterol acyl transferase; AdipoR2, adiponectin receptor; PPAR, peroxisome proliferator-activated receptor; CPT, carnitine palmitoyltransferase; ACOX1, acyl-coenzyme A oxidase; UCP, uncoupling proteins; CYP7A1, 7α-hydroxylase; LDL-R, low density lipoprotein receptor; ApoB, apolipoprotein B; PGC-1α, PPAR-γ coactivator-1α; MCAD, medium chain acyl-CoA dehydrogenase; LCAD, long-chain acyl-CoA dehydrogenase. | |
Bile acids are biosynthesized from endogenous metabolism of cholesterol via a pathway whose rate-limiting step is catalyzed by 7α-hydroxylase or cytochrome P450 7A1 (CYP7A1). The binding of bile acids by a sequestrant within the intestinal lumen can disrupt their enterohepatic circulation. This will lead to cholesterol catabolism and can effectively lower endogenous cholesterol, as the insoluble bile acid–sequestrant complex are not reabsorbed in the intestine and are eventually excreted in the faeces. Bile acid sequestrant drugs are synthetic ion-exchange polymers used as effective means of reducing bile acid re-absorption and endogenous cholesterol levels. The sequestrants are prescribed in conjunction with statins in hypercholesterolemic patients, if statins are not very effective or the patients are unable to tolerate statins at high doses.38 However, the use of sequestrants, such as cholestyramine, can result in unpleasant gastrointestinal side effects.39 Although some newly developed bile acid sequestrants have reduced incidences of gastrointestinal adverse effects, their use can interfere with the absorption of other drugs such as contraceptives.39 It is imperative, therefore, to investigate potential alternatives to synthetic sequestrants such as food protein-derived peptides.
Till date, most studies that evaluated the bile acid-binding properties of protein hydrolysates and peptides are based on isolated in vitro assays, which are not particularly reliable yardsticks for estimating possible in vivo functions considering the expected interactions of the peptides with the food matrix and physiological biomolecules. Due to favourable structural chemistry, some food protein hydrolysates have demonstrated substantial bile acid-binding activity,40–45 which is partly dependent on hydrophobicity of the amino acid residues of the pepetides42 although contradictory evidence has also been reported.40 Bile acids are amphipathic with hydrophobic skeletal structure and polar functionality and can thus bind other hydrophobic amino acids via weak hydrophobic forces.41 Consequently, food protein hydrolysates that contain high amounts of hydrophobic Leu and cationic Lys residues have shown high bile acid-binding capacity, and this could be attributed to the interaction of the amino acid residues with the hydrophobic core and anionic carboxylic acid group of bile acids, respectively. Moreover, the sequence and arrangement of the peptide molecules in space can also influence bioactivity although there is no literature evidence that demonstrated their impact on hypolipidaemic activity of food-derived peptides. In fact, a protein hydrolysate was observed to maintain similar activity after simulated gastrointestinal digestion indicating that the peptides were either resistant to further proteolysis or, more importantly, the peptide sequences, which may have been lost due to protease treatment, had meagre contributions to the observed bioactivity.
In addition, the bile acid-binding activity of enzymatic food protein hydrolysates depends on the type of bile acids, which possess different structural functionalities, such as variable number of hydroxyl groups that may influence their association with sequestrants. This has been demonstrated with different enzymatic protein hydrolysates that exhibited differential binding of different bile acid types43–45 although the structural requirements for activity and actual molecular interactions are yet to be elucidated. However, some protein hydrolysates have also been found to be ineffective in binding bile acids despite the moderate activities displayed by the unhydrolyzed proteins with up to 60% binding capacities for different bile acids.46 The use of the native protein in this case for controlling hyperlipidaemia is unrealistic since the proteins will less likely remain intact to exert their activity after oral intake due to gastrointestinal proteolysis.
Some studies have validated the hypolipidaemic effects of bile acid sequestrant protein hydrolysates in animal models. Fish protein hydrolysate with high amounts of glycine, taurine and hydrophobic amino acids was found to increase bile acid levels in the plasma of hyperlipidaemic rats with concomitant decreases in hepatic lipids, fasting plasma TG levels and visceral adipose tissue mass.47,48 It was noted that the presence of bile acids in the plasma played regulatory effects on plasma TG metabolism.47 Amino acids glycine and taurine can conjugate with bile acids in the hepatocytes, and their increased dietary intake can enhance bile acid processing and excretion.49 Moreover, peptide VAWWMY (soystatin) derived from soy glycinin (f129-134) exhibited the highest bile acid-binding activity of several glycinin-derived peptide fragments,22 which was also attributed mostly to its hydrophobic amino acid residues Trp and Tyr.50 As demonstrated in these studies, many hydrolysates and peptides displayed bile acid-binding activity in vitro to extents comparable to the activity of standard sequestrants such as cholestyramine. The adverse effects associated with most synthetic sequestrants are often associated with their high bile acid-binding activity.51 Therefore, food-derived hydrolysates and peptides that exhibit moderate bile acid-binding activity can potentially provide beneficial effects with less negative effects compared to synthetic drugs especially during mild hypercholesterolemia.
3.2. Alteration of cholesterol micellar solubility
The incorporation of dietary cholesterol into micelles is critical to absorption across the enterocytes into circulation. Many studies have reported that food protein hydrolysates and peptides inhibited cholesterol micellar solubility (CMS),52–55 and these effects can potentially impact intestinal absorption of cholesterol. High CMS inhibition associated with protein hydrolysates has led to substantial decrease in cholesterol uptake by cultured intestinal cells and corresponding reduction of lipid uptake with improved plasma lipid profiles in diet-induced hyperlipidaemic rats.52 The disruption of cholesterol micelles can be influenced by the duration of interaction55 and, most importantly, the abundance of highly hydrophobic amino acid residues in the protein hydrolysates.54 Based on high hydrophobicity index, a soy protein-derived hexapeptide WGAPSL was found to potently inhibit CMS in vitro.20 Hydrophobic peptides present in protein hydrolysates can possibly compete with cholesterol for incorporation into the micelles53 or displace cholesterol in the bile acid-phospholipid-cholesterol micelles.54 The availability of bile acid and phospholipids in the intestinal lumen can affect CMS since these components are critical to intestinal micelles formation. Therefore, the activity of hydrophobic peptides on CMS can possibly be due to their interaction with bile acids, making them unavailable for solubilisation of cholesterol in the micelles.
Recent studies have demonstrated that hydrophobic peptides can effectively bind different bile acids and also inhibit CMS,56,57 and similar dual effects observed for hydrophobic peptide VAWWMY compared favourably with the activity of cholestyramine at similar concentrations.21 These effects were thought to have contributed to the pronounced hypolipidaemic functions of the hexapeptide in inhibiting cholesterol absorption and distribution in the serum, liver and intestine of diet-induced rat model of hyperlipidaemia.21 Therefore, peptides that display multiple mechanisms of action can potentially provide stronger physiological hyperlipidaemic effects. Another important example is a β-lactoglobulin hydrolysate containing peptide IIAEK (lactostatin), which was found to reduce cholesterol uptake in cultured intestinal cells via CMS inhibition, also possibly contributing to the hypolipidaemic effects of lactostatin in the serum and hepatocytes of rats.18,58 With N-terminal hydrophobic (fragment IIA) and C-terminal hydrophilic (fragment EK) amino acid residues, the structure of lactostatin appears amphipathic, and the peptide may have favourably associated with phospholipids and disrupted the integrity of the micelles leading to decreased CMS. Therefore, inhibition of cholesterol solubility in intestinal micelles by peptidic amphiphiles in addition to bile acid-binding capacity of highly hydrophobic peptides can contribute immensely to the reduction of endogenous lipids associated with the absorption of dietary cholesterol and bile acids within the intestinal lumen. If reproduced in humans, these effects can provide a more natural approach for the treatment and management of mild hypercholesterolemia.
3.3. Alteration of hepatic and adipocytic enzyme activity, gene expression and lipid metabolism
Lipid homeostasis is important for proper physiological functioning59 and plasma lipid levels are often influenced by the amount of lipids consumed in the diet. The body's innate lipid production responds to exogenous intake by regulating de novo synthesis. There is considerable evidence that peptides can exert effects on endogenous biosynthesis of cholesterol and other lipids, particularly in the hepatocytes and adipocytes where lipid metabolism predominantly occurs.60 For instance, the reduction of plasma and hepatic lipids by whole soy milk protein hydrolysates was associated with the inhibition of fatty acid synthase (FAS) activity in the hepatocytes of rats.34 The expression of FAS gene is regulated by peroxisome proliferator-activated receptor (PPAR)-α, a nuclear receptor transcription factor that regulates a number of genes related to lipid metabolism.61 The hydrolysates also induced decreased activities of hepatic lipogenic malic enzyme and glucose-6-phosphate dehydrogenase (G6PDH), which may have potentiated hypolipidaemic functions. The inhibition of these enzymes has also been associated with the decreased plasma FFA, TG and TC without changes in hepatic TG and TC in obese diabetic rats.62 In addition to these effects, soy protein hydrolysates and its hydrophilic peptide fraction also inhibited hepatic phosphatidate phosphohydrolase (PAP) in fatty rats leading to positive lipid profile and decreased liver weight.63 PAP plays an important role in lipid metabolism and signal transduction. Apart from the enzyme inhibitory functions, inducers of LDL receptor (LDL-R) expression can also promote hypolipidaemia. Amongst several soy-derived peptides, an octapeptide FVVNASTN derived from the β chain of soy 7S β-conglycinin induced the strongest effect in upregulating LDL-R transcription in cultured HepT9A4 human hepatocytes, with the activities independent of peptide hydrophobicity.15 Cell surface LDL-R bind LDL leading to their assimilation and subsequent metabolism. Therefore, elevated expression of the receptors will enhance the removal of plasma LDL whereas a deficiency will lead to LDL accumulation.64 This function exhibited by FVVNASTN was thought to be responsible for the hypolipidaemic activity observed for soybean protein hydrolysates that contain the octapeptide.65
Moreover, food protein hydrolysates can regulate hepatic gene expressions related to lipid metabolism and energy expenditure. This has been demonstrated for brown rice protein hydrolysates, which induced a positive hepatic lipid profile and decreased adipose tissue mass by upregulating mRNA expression of proteins of fatty acid oxidation including PPARα, mitochondrial carnitine palmitoyltransferase 1 (CPT1) and peroxisomal acyl-coenzyme A oxidase 1 (ACOX1) as well as LDL-R and cholesterol-metabolising CYP7A1 in hepatocytes of hamsters.36 The hydrolysates also induced the downregulation of lipogenic stearoyl-CoA desaturase (SCD1), associated with improved energy expenditure, with concomitant decrease in lipid levels and deposition in adipose tissues. Other genes of lipogenic enzymes downregulated by protein hydrolysates treatments in animals include Δ5- and Δ6 desaturases, for endogenous fatty acid synthesis, and acyl-CoA cholesterol acyl transferase (ACAT),66 which catalyses the intestinal esterification of cholesterol prior to incorporation into chylomicrons. As earlier mentioned, there could be more than one peptide within the hydrolysates that exerted these beneficial effects. Accordingly, dipeptides DK, EK and WK, modelled after the C-terminal region of lactostatin, were reported to individually activate the upregulation of 7α-hydroxylase (CYP7A1) mRNA expression in HepG2 cells, and this effect can potentially activate cholesterol metabolism into bile acids for subsequent removal.67 Although the presence of other plant bioactives can potentiate hypolipidaemic functions of heterogeneous crude protein hydrolysates, there is considerable evidence that observed bioactivity could be due to peptides alone. In hepatic cell culture, enzymatic hydrolysates of isoflavone-free soybean β-conglycinin fraction increased LDL-R expression associated with decreased accumulation of apoB100, a functional unit of lipoproteins that originate from hepatocytes.68 This modulatory effect induced by the peptides within the hydrolysates can lead to decreased synthesis and endogenous levels of apoB100-associated LDL and VLDL. Similar effects have been demonstrated for pure soy dipeptides, which induced a decreased synthesis of TG (for dipeptides VK, SY and KA) and apoB accumulation (for dipeptide SY) in cultured hepatocytes; these effects were associated with the effects a soy protein hydrolysate fraction in inhibiting hepatic lipogenesis in fatty rats.63
Through a distinct mechanism, a highly hydrophobic opioid pentapeptide, soymorphin-5 (YPFVV), derived from soybean β-conglycinin β-subunit, was reported to upregulate PPARα expression, via elevation of adiponectin receptor (AdipoR2) gene expression, and this led to the activation of hepatic acyl-CoA oxidase, CPT1A and mitochondrial uncoupling protein (UCP)-2 concomitantly increasing fatty acid oxidation, energy expenditure and improving endogenous lipid profile of obese diabetic mice.69 Therefore, it is expected that the physiological effects of food protein hydrolysates on hepatic lipogenic proteins would potentially lead to overall modulation of hepatic lipid profiles. This has been demonstrated in other hyperlipidaemic animal models treated with various protein hydrolysates with observed modulation of elevated hepatic TG, TC and total lipids.23,25,35,52,54 Other unknown mechanisms can also contribute to the hepatic effects of these food protein hydrolysates considering the heterogeneity of the constituent peptides.
Furthermore, food protein hydrolysates can inhibit enzyme activity and modulate gene expression of lipid metabolism in adipocytes leading to a positive systemic hypolipidaemic effects. For example, in human adipocyte culture, enzymatic hydrolysates of α and α′ subunits of soybean β-conglycinin were found to inhibit FAS activity and pre-adipocyte differentiation (adipogenesis), characterized by decreased lipid accumulation in the cells.70 Likewise, fish protein hydrolysates were reported to induce elevated expression of mRNA of proteins that play key roles in fatty acid oxidation and energy expenditure in perirenal and retroperitoneal adipose tissues of rats, and these proteins include PPAR-γ coactivator-1α (PGC-1α), UCP-1, CPT1B, CPT2, medium chain acyl-CoA dehydrogenase (MCAD) and long-chain acyl-CoA dehydrogenase (LCAD).47 The reported gene regulation induced by the latter will ultimately result in upregulation of mitochondrial uncoupling functions and fatty acid oxidation, and these activities may have resulted in the corresponding positive impact of the hydrolysate product on endogenous lipid profiles and adipose tissue mass.
4. Multifunctional molecular roles of milk-derived lactostatin and β-lactotensin in modulating endogenous lipid profiles
Lactostatin (IIAEK, f71-75) and β-lactotensin (HIRL, f146-149) are derived from bovine β-lactoglobulin, and have both exhibited hypolipidaemic effects in animal models. Although lactostatin was found to reduce serum and hepatic TC and increase HDL cholesterol in rats via inhibition of CMS and binding of bile acids,18,35 more recent findings have indicated that the pentapeptide exhibit molecular effects on hepatic gene expression associated with cholesterol metabolism (Fig. 2). Lactostatin upregulated hepatic expression of 7α-hydroxylase (CYP7A1) via increased CYP7A1 promoter transcriptional activity, thereby enhancing cholesterol metabolism in HepG2 cells.71 The activity was attributed to the C-terminal fragment (EK) of lactostatin, as its absence led to reduction of CYP7A1 activity, and mediated by a Ca2+ signalling pathway. According to the study, intracellular Ca2+ influx due to treatment of HepG2 cells with lactostatin resulted in the activation of Ca2+/calmodulin-dependent protein kinase. This resulted in the activation of the MAPK/ERK kinase signal transduction pathway associated with the CYP7A1 promoter via bile acid response elements.71 On the other hand, β-lactotensin exhibited hypolipidaemic activity through a different mechanism mediated by neurotensin NT2 receptor and possibly by induction of bile acid secretion.72 Based on the study, the molecular functions of the milk protein-derived peptide induced a reduction of serum TC and LDL + VLDL cholesterol, with HDL unaltered, two days after oral administration of 100 mg of peptide per kg body weight in ICR mice that received high cholesterol diet. Since the peptides are derived from one protein source, their multiple mechanisms of action can enhance the lipid-lowering activity of enzymatic β-lactoglobulin hydrolysates containing lactostatin and β-lactotensin, and can possibly increase the utilization of the milk protein in the management of mild hyperlipidaemia.
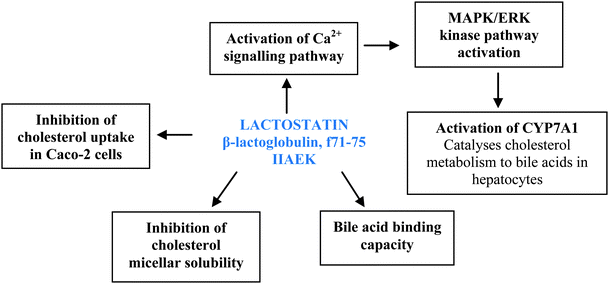 |
| Fig. 2 Molecular roles of milk-derived lactostatin in lowering lipids based on in vitro and cell culture studies. | |
5. Possible impact of absorption and bioavailability on hypolipidaemic functions
Several in vitro studies have provided insights regarding activity dynamics of potentially hypolipidaemic peptides, but are not reliable in determining their physiological bioavailability. A number of proteases and peptidases present within the gastrointestinal tract can act upon peptides, thus reducing or eliminating their bioactivity. It is believed that peptides of low molecular sizes, especially di- and tripeptides, are generally more resistant to hydrolysis by endogenous proteases and are more likely to enter into circulation intact to exert their systemic effects.73 Moreover, the abundance of certain amino acid residues can confer resistance to further peptidolysis; peptides containing high amounts of proline or hydroxyproline can survive further digestion by peptidases found within the brush-border of enterocytes.74 The molecular weights of known hypolipidaemic peptides range from 380 Da to 10 kDa. The hypolipidaemic peptides that exert their functions within the intestinal lumen by binding bile acids or disrupting micellar cholesterol solubility do not require transepithelial transport and can possess relatively higher molecular weights. However, peptides that act by altering hepatic or adipocytic enzymes and gene expression are required to enter into circulation. Therefore molecular size and resistance to further endogenous hydrolysis of the peptides are integral to their proper functioning. Nine hydroxyproline-containing di- and tripeptides appeared in the blood of healthy human subjects after oral intake of hypolipidaemic chicken collagen hydrolysates.25,75 However, there is currently a dearth of further evidence to support bioavailability of food protein-derived hypolipidaemic peptides, although the functions observed in hepatocytes and adipocytes indicate that the peptides, or at least their active fragments, are to some extent bioavailable in plasma and tissues.
Understanding peptide transport across the intestinal epithelium is crucial to elucidating their tissue distribution and functions. Caco-2 cell cultures are currently the most widely used model for evaluating peptide absorption since they display similar gene expression pattern as human intestinal cells.76 Following peptide transport studies, subsequent calculations are conducted to deduce the coefficient of permeability (Papp).76 Due to physical differences between physiological intestinal tissues and isolated Caco-2 cell monolayers, results from cell-based analysis may not reflect the actual absorption patterns of the peptides. Consequently, in situ evaluations of peptide transport have been explored for more reliable data. These methods involve perfusing a segment of rat intestine with solution of the peptide of interest, and measuring aliquots taken at specific time intervals77 followed by determination of the effective permeability (Peff).76,77
A number of peptide transporters are present within the intestinal epithelium. PepT1 can transport di- and tripeptides into the enterocytes via a proton gradient.78 However, there are a few exceptions; tripeptide VPP has been found to pass between enterocytes potentially escaping degradation by intracellular peptidases.79 Thus, other mechanisms of trans-epithelial transport of peptides exist and these include passage of hydrophilic peptides through enterocyte membrane tight junctions, transport of hydrophobic peptides directly through apical and basolateral membranes, and transport through membrane vesicles.78 Studies are needed to evaluate the absorption of hypolipidaemic peptides for better understanding of their bioavailability.
6. Production of hypolipidaemic peptides
The peptides described in this review, with the exception of lunasin, are produced through enzymatic hydrolysis of food proteins. This may occur during physiological or simulated gastrointestinal digestion, and can be facilitated using commercial exogenous proteases or food-grade microbial cultures. Proteases have varying physiochemical requirements, particularly pH and temperature, for optimal activity levels, and their function can be terminated by thermal or acid-induced inactivation when the desired degree of hydrolysis is achieved.80 Each protease exhibits a degree of cleavage site specificity. Endogenous proteases, such as pepsin and trypsin, display narrow peptide bond specificity resulting in a homogenous peptide mixture. Several hypolipidaemic peptides have been liberated from their parent proteins using gastrointestinal proteases. Protein hydrolysis with pepsin or trypsin has resulted in the production of various hypolipidaemic peptides including hexapeptide VAWWMY (soystatin) from soy glycinin,21 octapeptide IAVPGEVA from soy 11S globulin17 and hydrolysate fractions derived from egg white proteins.24 Moreover, pentapeptide IIAEK (lactostatin) was also liberated by tryptic digestion of β-lactoglobulin18 whereas hypolipidaemic LPYP and LPYPR were derived from the trypsin hydrolyzed fraction of soy glycinin.16 Lovati et al14 isolated lipid-lowering peptides after soy protein hydrolysis with a combination of pepsin and trypsin. In addition, some commercial proteases such as Alcalase can produce a vast array of hypolipidaemic peptides and hydrolysates from food proteins due to their broad specificity. For instance, hexapeptide WGAPSL was derived from soy proteins after hydrolysis with Alcalase.20 Likewise, hypolipidaemic hydrolysate fractions derived from white and brown rice proteins,36 amaranth seeds proteins,40 and bovine heart proteins52 were produced using Alcalase. Other microbial culture-derived proteolytic activities were also used for the production of hypolipidaemic FVVNATSN from soy proteins.15
Following proteolysis, the resulting products are often processed to enhance the activity of the constituent peptides. Frequently used post-hydrolysis processing methods include ultrafiltration, chromatography and filtration using activated carbon matrix.7 These techniques can separate peptides in the protein hydrolysates based on physiochemical parameters such as hydrophobicity, molecular size and net charge. Once separated, the peptides can be purified and amino acid sequence determined by mass spectrometry. For bile acid-binding hypolipidaemic peptides, hydrophobicity has been established as a major factor in determining activity. Reverse-phase (RP)-HPLC is a popular method for separating peptides based on hydrophobic properties. Several lipid-lowering peptides such as FVVNATSN,15 VAWWMY,22 LRVPAGTTFYVVNPDNDENLRMIA,14 IAVPGEVA,17 IIAEK18 and WGAPSL20 were identified after RP-HPLC separation. Moreover, Kagawa et al19 isolated hypolipidaemic VVYP after conducting both ultrafiltration and cationic fractionation on enzymatic hydrolysates of bovine heamoglobin. Furthermore, hexapeptide VAWWMY22 was identified after enzymatic soy protein hydrolysates were subjected to anion-exchange chromatography.
7. Commercial hypolipidaemic peptide products
Currently, a limited number of commercial hypolipidaemic biopeptide products exist in the market despite the extensive literature and active interest on the topic. Lunasin, a 43-amino acid residue soybean peptide, is the principal component of commercial products Lunasin XP™ and LunaSoy™.81 Lunasin has been reported to reduce the activity of 3-hydroxy-3-methyl-glutaryl-CoA reductase by down-regulating its gene expression, resulting in decreased de novo cholesterol synthesis.82 The soy peptide has also been reported to upregulate LDL-R expression,7,81 which will lead to further enhancement of plasma lipid profile. There is limited information regarding the 3-dimensional structure of lunasin as it relates to its physiological bioactivity, but a recent conformational study revealed that lunasin contains three α-helices within its structure.83 Lunasin has also been isolated from a variety of non-soy protein extracts including barley,84 rye,85 amaranth seeds86 and wheat.87 In addition to the lunasin-based products, Soyscience® is a soy peptide and phospholipid product claimed to possess hypolipidaemic function.88 Oral administration of the product to hypercholesterolaemic patients and rats that received high-fat diet resulted in reduction of plasma TC/LDL and increase in HDL.88 The product, which has been granted the “generally recognized as safe” status, was also reported to reduce cholesterol uptake in men that were fed a high-fat diet.88 Another product is Peptide Soup produced by Nippon Supplement Inc.;89 limited information is available regarding this product other than its use for reducing plasma lipids. With the available information, there is strong market opportunity in using functional food products containing hypolipidaemic peptides for health promotion.
8. Conclusion and future direction
Although most bioactive peptides are known to possess bitter taste and may contribute certain unfavourable quality in the food system through their involvement in biochemical processes (e.g. Maillard reaction), their putative health benefits compared to intact parent proteins have attracted particular interest in the recent past. Food protein hydrolysates containing bioactive peptides have shown promising activities for the prevention and management of CVD and associated health complications. Reduced plasma levels of LDL–cholesterol and TG as well as increased HDL–cholesterol are associated with reduced CVD risk. Based on recent literature, the major mechanisms involved in the reduction of lipids include bile acid-binding, disruption of micellar cholesterol solubility, and modification of hepatic and adipocytic enzyme activity and gene expression of lipogenic proteins. Although there is ample preclinical evidence based on animal and cell culture studies regarding hypolipidaemic activity, carefully conducted human-based studies are urgently needed in order to evaluate safety and substantiate efficacy of the food protein hydrolysates and peptides. Furthermore, cost-effective large-scale industrial production of potent peptides, a consistent challenge in research and development related to bioactive peptides, will immensely enhance commercialization of affordable hypolipidaemic products. Besides, alternative food protein sources of the active peptide sequences should be explored to ensure sustainability of the bioresources. For example, a search of the UniProtKB/Swiss-Prot database has revealed that lactostatin (IIAEK, bovine β-lactoglobulin f71-75) is highly conserved in β-lactoglobulin derived from water buffalo, domestic sheep, mouflon and domestic goat. The availability of the hypolipidaemic peptide in other milk protein sources can increase their application in health promotion, as their production would not be restricted to the bovine source. The same is applicable to lunasin, which has been found in other plant food sources. Till date, only a few specific hypolipidaemic peptide sequences have been identified with most research focused on protein hydrolysate fractions of known amino acid profiles. As amino acid composition is insufficient in understanding structure–activity relationships, the structure and spatial configuration of hypolipidaemic peptides, if known, will provide better insight on structural requirements for potency. Furthermore, the absorption and bioavailability of hypolipidaemic peptides should be strongly considered since the observed physiological functions can be due to peptide fragments that result from endogenous proteolysis.
References
- A. C. I. Boullart, J. de Graaf and A. F. Stalenhoef, Biochim. Biophys. Acta, 2012, 1821, 867–875 CrossRef CAS.
- Y. Momiyama, R. Ohmori, Z. A. Fayad, N. Tanaka, R. Kato, H. Taniguchi, M. Nagata and F. Ohsuzu, Atherosclerosis, 2012, 222, 577–580 CrossRef CAS.
- R. L. Page II, V. Grushchyan and K. Nair, American Health and Drug Benefits, 2011, 4, 280–288 Search PubMed.
- S. Sathasivam, Eur. J. Intern. Med., 2012, 23, 317–324 CrossRef CAS.
- S. Padala and P. D. Thompson, Atherosclerosis, 2012, 222, 15–21 CrossRef CAS.
- W. Hochholzer, D. D. Berg and R. P. Giugliano, Ther. Adv. Cardiovasc. Dis., 2011, 5, 227–240 CrossRef CAS.
- C. C. Udenigwe and R. E. Aluko, J. Food Sci., 2012, 77, 11–24 CrossRef.
- D. Martínez-Maqueda, B. Miralles, I. Recio and B. Hernández-Ledesma, Food Funct., 2012, 3, 350–361 Search PubMed.
- A. G. P. Samaranayaka and E. C. Y. Li-Chan, J. Funct. Foods, 2011, 3, 229–254 CrossRef CAS.
- D. Agyei and M. K. Danquah, Trends Food Sci. Technol., 2012, 23, 62–69 CrossRef CAS.
- C. Lico, L. Santi, R. M. Twyman, M. Pezzotti and L. Avesani, Plant Cell Rep., 2012, 31, 439–451 CrossRef CAS.
- J. T. Ryan, R. P. Ross, D. Bolton, G. F. Fitzgerald and C. Stanton, Nutrients, 2011, 3, 765–791 CrossRef CAS.
- S. Lordan, R. P. Ross and C. Stanton, Mar. Drugs, 2011, 9, 1056–1100 CrossRef CAS.
- M. R. Lovati, C. Manzoni, E. Gianazza, A. Arnoldi, E. Kurowska, K. K. Carroll and C. R. Sirtori, J. Nutr., 2000, 130, 2543–2549 CAS.
- S.-J. Cho, M. A. Juillerat and C.-H. Lee, J. Agric. Food Chem., 2008, 56, 4372–4376 CrossRef CAS.
- D. Y. Kwon, S. W. Oh, J. S. Lee, H. J. Yang, S. H. Lee, J. H. Lee, Y. B. Lee and H. S. Sohn, Food Sci. Biotechnol., 2002, 11, 55–61 CAS.
- V. V. Pak, M. S. Koo, T. D. Kasymova and D. Y. Kwon, Chem. Nat. Compd., 2005, 41, 710–714 CrossRef CAS.
- S. Nagaoka, Y. Futamura, K. Miwa, T. Awano, K. Yamauchi, Y. Kanamaru, K. Tadashi and T. Kuwata, Biochem. Biophys. Res. Commun., 2001, 281, 11–17 CrossRef CAS.
- K. Kagawa, H. Matsutaka, C. Fukuhama, Y. Watanabe and H. Fujino, Life Sci., 1996, 58, 1745–1755 CrossRef CAS.
- F. Zhong, X. Zhang, J. Ma and C. F. Shoemaker, Food Res. Int., 2007, 40, 756–762 CrossRef CAS.
- S. Nagaoka, A. Nakamura, H. Shibata and Y. Kanamaru, Biosci., Biotechnol., Biochem., 2010, 74, 1738–1741 CrossRef CAS.
- S.-K. Choi, M. Adachi and S. Utsumi, Biosci., Biotechnol., Biochem., 2002, 66, 2395–2401 CrossRef CAS.
- Y. Zhang, T. Kouguchi, K. Shimizu, M. Sato, Y. Takahata and F. Morimatsu, J. Nutr. Sci. Vitaminol., 2010, 56, 208–210 CrossRef CAS.
- M. A. Manso, M. Miguel, J. Even, R. Hernández, A. Aleixandre and R. López-Fandiño, Food Chem., 2008, 109, 361–367 CrossRef CAS.
- Y.-H. Lin, J.-S. Tsai, L.-B. Hung and B. S. Pan, Food Chem., 2011, 125, 397–401 CrossRef CAS.
- X. Liu, M. Zhang, C. Zhang and C. Liu, Food Chem., 2012, 134, 2134–2140 CrossRef CAS.
- O. A. Alhaj, A. D. Kanekanian, A. C. Peters and A. S. Tatham, Food Chem., 2010, 123, 430–435 CrossRef CAS.
- H. Ben Khaled, Z. Ghlissi, Y. Chtourou, A. Hakim, N. Ktari, M. A. Fatma, A. Barkia, Z. Sahnoun and M. Nasri, Food Res. Int., 2012, 45, 60–68 CrossRef CAS.
- E. Y. Jung, H.-S. Lee, H. J. Lee, J.-M. Kim, K.-W. Lee and H. J. Suh, Nutr. Res., 2010, 30, 783–790 CrossRef CAS.
- H. Wergedahl, O. A. Gudbrandsen, T. H. Røst and R. K. Berge, Nutrition, 2009, 25, 98–104 CrossRef CAS.
- C.-F. Zhu, G.-Z. Li, H.-B. Peng, F. Zhang, Y. Chen and Y. Li, Appl. Physiol., Nutr., Metab., 2010, 35, 797–804 CrossRef CAS.
- A. M. Turpeinen, M. Kumpu, M. Rönnback, L. Seppo, H. Kautiainen, T. Jauhiainen, H. Vapaatalo and R. Korpela, J. Funct. Foods, 2009, 1, 260–265 CrossRef CAS.
- Y. Bae, S. M. Kim, S. Lee and H. G. Lee, New Biotechnol., 2010, 27, 85–88 CrossRef.
- J.-Y. Choi, J.-E. Jeon, S.-Y. Jang, Y.-J. Jeong, S.-M. Jeon, H.-J. Park and M.-S. Choi, J. Agric. Food Chem., 2011, 59, 2584–2591 CrossRef CAS.
- Y. Wakasa, C. Tamakoshi, T. Ohno, S. Hirose, T. Goto, S. Nagaoka and F. Takaiwa, J. Agric. Food Chem., 2011, 59, 3845–3850 CrossRef CAS.
- H. Zhang, G. E. Bartley, C. R. Mitchell, H. Zhang and W. Yokoyama, J. Agric. Food Chem., 2011, 59, 10927–10933 CrossRef CAS.
- E. Revilla, C. Santa Maria, E. Miramontes, J. Bautista, A. García-Martínez, O. Cremades, R. Cert and J. Parrado, Food Res. Int., 2009, 42, 387–393 CrossRef CAS.
- D. J. Blom, S. Afr. Fam. Pract., 2011, 53(1), 11–18 Search PubMed.
- J. G. Shanes, Curr. Atheroscler. Rep., 2012, 14, 33–40 CrossRef CAS.
- A. Tiengo, E. M. P. Motta and F. M. Netto, Plant Foods Hum. Nutr., 2011, 66, 370–375 CrossRef CAS.
- G. Porez, J. Prawitt, B. Gross and B. Staels, J. Lipid Res., 2012, 53, 1723–1737 CrossRef CAS.
- J. U. Kongo-Dia-Moukala, H. Zhang and P. C. Irakoze, Int. J. Mol. Sci., 2011, 12, 1066–1080 CrossRef CAS.
- C. Barbana, A. C. Boucher and J. I. Boye, Food Res. Int., 2011, 44, 174–180 CrossRef CAS.
- Y. Yoshie-Stark, Y. Wada and A. Wäsche, Food Chem., 2008, 107, 32–39 CrossRef CAS.
- Y. Ma and Y. L. Xiong, J. Agric. Food Chem., 2009, 57, 4372–4380 CrossRef CAS.
- P. W. M. L. H. K. Marambe, P. J. Shand and J. P. D. Wanasundara, J. Am. Oil Chem. Soc., 2008, 85, 1155–1164 CrossRef CAS.
- B. Liaset, L. Madsen, Q. Hao, G. Criales, G. Mellgren, H.-U. Marschall, P. Hallenborg, M. Espe, L. Frøyland and K. Kristiansen, Biochim. Biophys. Acta, Mol. Cell Biol. Lipids, 2009, 1791, 254–262 CrossRef CAS.
- B. Liaset and M. Espe, Process Biochem., 2008, 43, 42–48 CrossRef CAS.
- D. A. Vessey, J. Whitney and J. L. Gollan, Biochem. J., 1983, 214, 923–927 CAS.
- T. Takeshita, M. Okuchi, R. Kato, C. Kaga, Y. Tomita, S. Nagaoka and H. Honda, J. Biosci. Bioeng., 2011, 112, 92–97 CrossRef CAS.
- I. Johnston, J. Nolan, S. S. Pattni and J. R. E. Walters, Curr. Gastroenterol. Rep., 2011, 13, 418–425 CrossRef.
- K. Nakade, H. Kaneko, T. Oka, A. M. Ahhmed, M. Muguruma, M. Numata and S. Nagaoka, Biosci., Biotechnol., Biochem., 2009, 73, 607–612 CrossRef CAS.
- M. del Mar Yust, M. del Carmen Millán-Linares, J. M. Alcaide-Hidalgo, F. Millán and J. Pedroche, J. Sci. Food Agric., 2012, 92, 1994–2001 CrossRef.
- H. Zhang, W. H. Yokoyama and H. Zhang, J. Sci. Food Agric., 2012, 92, 1395–1401 CrossRef CAS.
- C. Megías, J. Pedroche, M. del Mar Yust, M. Alaiz, J. Girón-Calle, F. Millán and J. Vioque, Plant Foods Hum. Nutr., 2009, 64, 86–93 CrossRef.
- J. U. Kongo-Dia-Moukala, J. Nsor-Atindana and H. Zhang, Asian J. Biochem., 2011, 6, 439–449 CrossRef CAS.
- Y.-H. Lin, J.-S. Tsai, L.-B. Hung and B. S. Pan, Food Chem., 2010, 123, 395–399 CrossRef CAS.
- C. Kirana, P. F. Rogers, L. E. Bennett, M. Y. Abeywardena and G. S. Patten, J. Agric. Food Chem., 2005, 53, 4623–4627 CrossRef CAS.
- L. Goedeke and C. Fernández-Hernando, Cell. Mol. Life Sci., 2012, 69, 915–930 CrossRef CAS.
- R. F. Schwabe and J. J. Maher, Gastroenterology, 2012, 142(1), 8–11 CrossRef.
- A. P. L. Jensen-Urstad and C. F. Semenkovich, Biochim. Biophys. Acta, Mol. Cell Biol. Lipids, 2012, 1821, 747–753 CrossRef CAS.
- M. Shimizu, S. Tanabe, F. Morimatsu, K. Nagao, T. Yanagita, N. Kato and T. Nishimura, Biosci., Biotechnol., Biochem., 2006, 70, 112–118 CrossRef CAS.
- N. Inoue, K. Nagao, K. Sakata, N. Yamano, P. E. R. Gunawardena, S.-Y. Han, T. Matsui, T. Nakamori, H. Furuta, K. Takamatsu and T. Yanagita, Lipids Health Dis., 2011, 10, 85 CrossRef CAS.
- G.-W. Go and A. Mani, Yale J. Biol. Med., 2012, 85, 19–28 CAS.
- S.-J. Cho, M. A. Juillerat and C.-H. Lee, J. Agric. Food Chem., 2007, 55, 10599–10604 CrossRef CAS.
- H. Wergedahl, B. Liaset, O. A. Gudbrandsen, E. Lied, M. Espe, Z. Muna, S. Mørk and R. K. Berge, J. Nutr., 2004, 134, 1320–1327 CAS.
- K. Morikawa, K. Ishikawa, Y. Kanamaru, G. Hori and S. Nagaoka, Biosci., Biotechnol., Biochem., 2007, 71, 821–825 CrossRef CAS.
- Y. Mochizuki, M. Maebuchi, M. Kohno, M. Hirotsuka, H. Wadahama, T. Moriyama, T. Kawada and R. Urade, J. Agric. Food Chem., 2009, 57, 1473–1480 CrossRef CAS.
- Y. Yamada, A. Muraki, M. Oie, N. Kanegawa, A. Oda, Y. Sawashi, K. Kaneko, M. Yoshikawa, T. Goto, N. Takahashi, T. Kawada and K. Ohinata, Am. J. Physiol., 2012, 302, E433–440 CAS.
- E. Gonzalez de Mejia, C. Martinez-Villaluenga, M. Roman and N. A. Bringe, Food Chem., 2010, 119, 1571–1577 CrossRef CAS.
- K. Morikawa, I. Kondo, Y. Kanamaru and S. Nagaoka, Biochem. Biophys. Res. Commun., 2007, 352, 697–702 CrossRef CAS.
- R. Yamauchi, K. Ohinata and M. Yoshikawa, Peptides, 2003, 24, 1955–1961 CrossRef CAS.
- A. Panchaud, M. Affolter and M. Kussman, J. Proteomics, 2012, 75, 3546–3559 CrossRef CAS.
- M. Segura-Campos, L. Chel-Guerro, B. Bentacur-Ancona and V. M. Hernandez-Escalante, Food Rev. Int., 2011, 27, 213–226 CrossRef CAS.
- K. Iwais, Y. Zhang, T. Kouguchi, A. Saiga-Egusa, M. Shimizu, T. Ohmori, Y. Takahata and F. Morimatsu, Nippon Shokuhin Kagaku Kogaku Kaishi, 2009, 56, 326–330 CrossRef.
- E. Le Ferrec, C. Chesne, P. Atursson, D. Brayden, G. Fabre, P. Gires, F. Guillou, M. Rousset, W. Rubas and M.-L. Scarino, ATLA, Altern. Lab. Anim., 2001, 29, 649–668 CAS.
- L. Barthe, J. Woodley and G. Houin, Fundam. Clin. Pharmacol., 1999, 13, 154–168 CrossRef CAS.
- V. Vermeirssen, J. Van Camp and W. Verstraete, Br. J. Nutr., 2004, 92, 357–366 CrossRef CAS.
- M. Satake, M. Enjoh, Y. Nakamura, T. Takano, Y. Kawamura, S. Arai and M. Shimizu, Biosci., Biotechnol., Biochem., 2002, 66, 378–384 CrossRef CAS.
- R. E. Aluko, J. AOAC Int., 2008, 91, 947–956 CAS.
-
Soy Labs, http://soylabs.com, accessed 26 July 2012.
-
C. C. Udenigwe and R. E. Aluko, in Bioactive Food Proteins and Peptides: Applications in Human Health, ed. N. S. Hettiarachchy, K. Sato, M. R. Marshall and A. Kannan, CRC Press, Taylor & Francis Group, Boca Raton, FL, USA, 2012, pp. 191–218 Search PubMed.
- P. Singh and K. Bisetty, S. Afr. J. Chem., 2012, 65, 115–124 CAS.
- H. J. Jeong, J. B. Jeong, C. C. Hsieh, B. Hernández-Ledesma and B. O. de Lumen, Nutr. Cancer, 2010, 62, 1113–1119 CrossRef CAS.
- H. J. Jeong, J. R. Lee, J. B. Jeong, J. H. Park, Y.-K. Cheong and B. O. de Lumen, Nutr. Cancer, 2009, 61, 680–686 CrossRef CAS.
- C. Silva-Sánchez, A. P. Barba de la Rosa, M. F. León-Galván, B. O. de Lumen, A. de León-Rodríguez and E. González de Mejía, J. Agric. Food Chem., 2008, 56, 1233–1240 CrossRef.
- H. J. Jeong, J. B. Jeong, D. S. Kim, J. H. Park, J. B. Lee, D.-H. Kweon, G. Y. Chung, E. W. Seo and B. O. de Lumen, Cancer Lett., 2007, 255, 42–48 CrossRef CAS.
-
Kyowa Hakko USA, http://www.kyowa-usa.com, accessed 27 July 2012.
-
Nippon Supplement, http://www.nippon-sapuri.com/english/index, accessed 4 August 2012.
|
This journal is © The Royal Society of Chemistry 2013 |
Click here to see how this site uses Cookies. View our privacy policy here.