Toxic effects of copper-based nanoparticles or compounds to lettuce (Lactuca sativa) and alfalfa (Medicago sativa)†
Received
14th October 2014
, Accepted 28th November 2014
First published on 28th November 2014
Abstract
The increased production and use of nanoparticles (NPs) has generated concerns about their impact on living organisms. In this study, nCu, bulk Cu, nCuO, bulk CuO, Cu(OH)2 (CuPRO 2005, Kocide 3000), and CuCl2 were exposed for 15 days to 10 days-old hydroponically grown lettuce (Lactuca sativa) and alfalfa (Medicago sativa). Each compound was applied at 0, 5, 10, and 20 mg L−1. At harvest, we measured the size of the plants and determined the concentration of Cu, macro and microelements by using ICP-OES. Catalase and ascorbate peroxidase activity was also determined. Results showed that all Cu NPs/compounds reduced the root length by 49% in both plant species. All Cu NPs/compounds increased Cu, P, and S (>100%, >50%, and >20%, respectively) in alfalfa shoots and decreased P and Fe in lettuce shoot (>50% and >50%, respectively, excluding Fe in CuCl2 treatment). Biochemical assays showed reduced catalase activity in alfalfa (root and shoot) and increased ascorbate peroxidase activity in roots of both plant species. Results suggest that Cu NPs/compounds not only reduced the size of the plants but altered nutrient content and enzyme activity in both plant species.
Environmental impact
The data on the effects of Cu-based nanoparticles in terrestrial plants is very limited. Moreover, the effects of Cu-based nanoparticles on nutrient quality of food crops have yet to be reported. In this research we found that all Cu-based nanoparticles reduced root size of alfalfa and lettuce. We also found species-specific effects of Cu-based NPs on nutrient elements uptake and translocation. On one hand, all Cu-based NPs reduced P and Fe in lettuce leaves; on the other hand, all Cu-based NPs reduced Fe but increased P, S, and Cu in alfalfa leaves. In addition, the activity of stress enzyme CAT was not affected in lettuce and sharply reduced in alfalfa shoot.
|
Introduction
Nanoparticles (NPs) are materials with at least two dimensions between 1 and 100 nm.1 The small size and large surface area provide NPs with different physical strength, chemical reactivity, electrical conductivity, magnetism, and optical effects, compared to bulk materials.2,3 These special properties allow NP utilization in electronics, engineering, energy production, catalysis, pharmaceutics, cosmetics, textiles, food industry, and agricultural products, among others.4–6 Recent statistics indicate that 1317 products containing NPs were on the market in 2010,7 and it has been estimated that there was a global release of 22
000–80
400, 1100–29
200, and 590–4800 metric tons of engineered nanomaterials into soil, water and atmosphere, respectively.8 The increase in NP utilization has raised concerns about their release into the environment and possible impacts on living organisms.9,10
Copper nanoparticles (nCu) and copper oxide nanoparticles (nCuO) are widely used in different fields.11–14 Very few studies have reported the effects of these two Cu-based NPs to terrestrial plants.10,15–21 Shah and Belozerova15 found that the shoot/root ratio in lettuce plants treated for 15 days with 0.013% (w/w) nCu in soil was 2.7, while in control plants the ratio was 1.4. This suggests nCu affects lettuce growth. Another report indicates that, compared to control, 1000 mg nCu/L reduced by 77% the root length and by 90% the biomass of Zucchini (Cucurbita pepo) grown in hydroponics.10 Similarly, Musante and White16 observed that nCu not only affected root length and biomass of zucchini, but also reduced the transpiration volume by 51% in plants exposed to 100 mg nCu/L and 61% in plants treated with 500 mg nCu/L. Lee et al.17 reported that the seedlings' length of Mung bean (Phaseolus radiatus) and wheat (Triticum aestivum L.) were reduced by 60% and 75%, respectively, when exposed to 1000 mg nCu/L. They also observed less reduction in shoot growth compared to root, which could be associated with low translocation of NPs from roots to shoots.18nCuO has also shown to affect plants in different ways. Reports indicate that nCuO did not affect seed germination in zucchini10 and maize (Zea mays L.);18 however, other reports mentioned root length reduction in wheat19 and duckweed (Landoltia punctata),20 and DNA damage in radish (Raphanus sativus), perennial ryegrass (Lolium perenne), and annual ryegrass (Lolium rigidum).21
The activity of antioxidant enzymes such as catalase (CAT) and ascorbate peroxidase (APX) can be affected in Cu exposed plants. These enzymes, which are overproduced under abiotic or biotic stress,22 protect plants from reactive oxygen species (ROS) damage. Excess ROS can damage proteins, lipids, and DNA.23 Hou et al.24 reported that Cu2+, up to 10 mg L−1, increased CAT activity in duckweed in a concentration-dependent manner; however, a reduction in CAT activity was observed when Cu2+ was higher than 10 mg L−1. A similar trend was observed in APX activity on duckweed treated with copper sulfate.25nCuO were also found to increase CAT activity in cucumber (Cucumis sativus) and wheat.26,27 Besides the effects of nCuO on seedlings growth and CAT and APX activity, no reports were found about the effects of Cu NPs/compounds on nutrient uptake by crop plants.
Recent literature has shown that nanoparticulate forms of Cu are more effective against pathogenic fungi than the corresponding bulk forms.28 Thus, very likely, in the near future, there will be an intensive use of nCu and nCuO in agricultural practices. In addition, CuPRO 2005 and kocide 3000 (Cu(OH)2-based materials) have been cataloged as nanoparticulate Cu species. These compounds are intensely used in agricultural production due to their fungicidal properties.29–31 Thus, the possibility of Cu-based NPs contamination of food supply and entrance into the food chain is increasing. However, it is not well understood yet if their effects are different from those of Cu bulk materials. Thus, three Cu-based NPs and other Cu compounds were evaluated in lettuce (Lactuca sativa) and alfalfa (Medicago sativa). The plants were selected because they are intensely produced and exposed to pesticides. Alfalfa is one of the most important forage crops worldwide32 and lettuce is cultivated worldwide and eaten in a raw form by many people. In this study, root and shoot elongations were measured with a ruler, macro and micronutrients' uptake by using inductively couple plasma-optical emission spectroscopy (ICP-OES), and enzyme activity with UV-Vis.
Materials and methods
Characterization of Cu NPs/Compounds and preparation of suspensions/solutions
The Cu particles used in this study include nanoparticulate CuO (denoted nCuO), micron-sized Cu and CuO (denoted bulk Cu and CuO respectively; all obtained from Sigma Aldrich), nanoparticulate Cu (US Research Nanomaterials, denoted nCu), Kocide 3000 (Dupont, Wilmington, DE), and CuPRO 2005 (SePRO, Carmel, IN). Reagent grade CuCl2 salt was obtained from Sigma Aldrich. The size and surface charge of particles at pH 7 (0.5 mM phosphate buffer) were determined by measuring hydrodynamic diameter (HDD) and zeta (ζ) potential using a Zetasizer Nano-ZS90 (Malvern, UK). A previous study33 showed that phosphate buffer, at the concentration used, had only minimal effects on zeta potential measurements. Primary particle size and morphology were determined via scanning electron microscopy (FEI XL40 Sirion) equipped with an Oxford INCA energy-dispersive X-ray spectroscopy (EDS) probe. Copper content (wt%) of each particle was determined via ICP-AES (iCAP 6300, Thermo Scientific).34,35 The main copper phase and crystal structure of particles were determined via X-ray diffraction (XRD, Bruker D8 Advance).
Cu NPs/compounds' suspensions/solutions were prepared at 0, 5, 10, and 20 mg L−1 in modified Hoagland's nutrient solution36 and homogenized by sonication in a water bath (Crest Ultrasonics, Trenton, NJ) at 25 °C for 30 min. There were four replicates per treatment.
Seed germination and plant growth
Seeds of alfalfa (Medicago sativa L.) Mesa variety and black seeded Simpson lettuce (Lactuca sativa L.) were stirred in 4% ClONa solution for 30 min, rinsed with deionized water (DI) three times and kept in DI for 24 h. Subsequently, seeds were rolled in autoclaved wet germination paper towels, as described by Carrillo-Castañeda et al.37 Ten drops of antimycotic/antibiotic solution (Sigma 5955) were added to the seeds before the paper was rolled. The rolls were put into Mason jars containing approximately 10 ml of DI, incubated in the dark for four days and exposed to light for one day. After that, the young plants were transferred into magenta boxes containing 300 mL of modified Hoagland's nutrient solution. All the boxes and lids were covered with aluminum foil to prevent algae growth. Aquarium pumps were used to aerate boxes, which were put in an Environmental Growth Chamber (TC2 Microcontroller, Chagrin falls, OH) with light intensity of 300 μmol m−2 s−1, 25/20 °C day/night temperature, and 65% relative humidity.
After 10 days of growth in the nutrient solution, seedlings were transferred to magenta boxes containing the Cu NPs/compounds suspended in nutrient solution and were cultivated for 15 days. Subsequently, plants were removed from the growth medium, washed with tap water and rinsed with DI. The length of the primary root and shoot for each seedling was measured with a ruler and the samples were saved for further analyses. There were 20 young plants per replicate.
Sample preparation for ICP-OES
At the end of exposure time, plants were removed from the growth medium, washed with tap water and rinsed with DI, as previously described in the literature.18,19,21 Subsequently, the samples were put in paper envelops, oven dried at 70 °C for 72 h, weighed, and digested with plasma pure HNO3 (65%) and H2O2 (30%) (1
:
4). Standard Reference Material NIST 1547 (Gaithersburg, MD) and samples spiked with 5, 10, and 20 mg L−1 of Cu NPs were processed and read as samples for quality control (QC) and quality assurance (QA). Digestion was performed in a CEM microwave accelerated reaction system (MarsX, Mathews, NC). The digests were adjusted to 15 ml with DI water and analyzed for Cu, micro and macroelement concentrations using inductively coupled plasma-optical emission spectroscopy (ICP-OES, PerkinElmer Optima 4300 DV, Shelton, CT)
Enzyme assays
Determination of catalase specific activity.
The CAT (EC 1.11.1.6) enzyme activity was measured according to Gallego et al.38 Plant samples were grinded in 0.1 M KH2PO4 buffer (pH 7.0) and centrifuged at 4500 rpm for 5 min (Eppendorf AG bench centrifuge 5417 R, Hamburg, Germany). The supernatant was separated, added with 73 mM H2O2, and measured at 240 nm through UV/Vis (Perkin Elmer Lambda 14 Spectrometer).
Determination of ascorbate peroxidase activity.
The APX (EC 1.11.1.11) enzyme activity was determined following the method of Nakano and Asada.39 Tissue samples were homogenized in phosphate buffer (pH 7.4) with a ratio of 10% w/v. The homogenate was centrifuged at 4 °C for 25 min at 14
000 rpm. Then, 100 μL of the supernatant was added with 886 μL of KH2PO4 buffer (0.1 M, pH 7.4), 4 μL of 25 mM ascorbate, and 10 μL of 17 mM H2O2. Absorbance was recorded at 290 nm using UV/Vis Spectrometer.
Statistics analysis
The data (means from four replicate/treatments) was analyzed using One-way ANOVA, followed by Tukey-Honestly Significant Difference multiple comparisons test (SPSS 19.0 package, Chicago, IL).
Results and discussion
Characterization of the Cu NPs/Compounds
Major physicochemical properties of the particles are presented in Table S1.†nCuO has particles between 10–100 nm, but much larger particles were found in nCu (up to 10 μm; Fig. 1). A reasonable fraction of bulk CuO particles were less than 1 μm (as indicated by SEM and dynamic light scattering analyses) but bulk Cu particles were much larger and diverse in morphology. Bulk Cu contains dendritic, plate-like, and irregularly-shaped particles (Fig. 1). Kocide 3000 and CuPRO 2005 mainly consist of micron-sized spherical particles, and the main copper phase in them are orthorhombic Cu(OH)2. While bulk Cu only contained Cu as the main phase, the presence of Cu2O was observed in nCu, possibly due to higher reactivity of the nanoparticles which may lead to slight oxidation during synthesis and/or storage. All the particles were negatively charged at pH 7 as confirmed by zeta potential measurement. The presence of a small amount of carbon was observed in the nanoparticles, possibly from a surfactant used by the manufacturer to stabilize the NPs. In addition to carbon, Kocide 3000 and CuPRO 2005 also contain oxygen, sodium, aluminum, and silicon (Fig. 2).
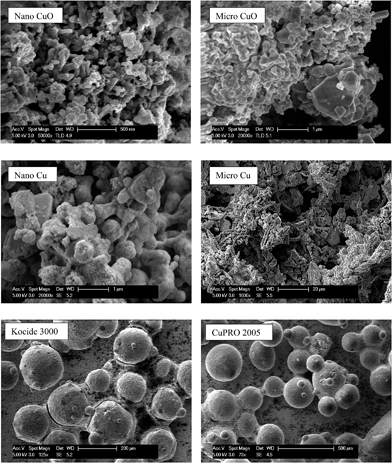 |
| Fig. 1 SEM micrographs of particles used in this study. | |
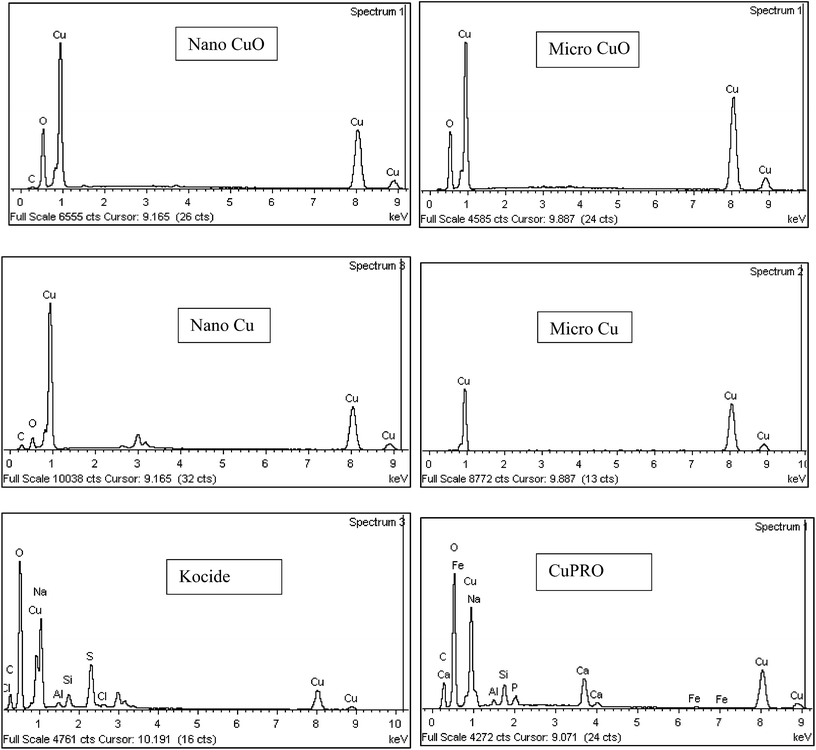 |
| Fig. 2 Energy-dispersive X-ray spectroscopy (EDS) of particles used in this study. | |
Effects of Cu NPs/compounds on root/shoot elongation
The size of roots of alfalfa and lettuce plants treated for 15 days with Cu NPs/compounds is shown in Fig. S1.† After 15 days of treatment, the roots of plants treated with Cu NPs/compounds, showed brown color compared to controls. As seen in Fig. S1,† at all concentrations, all Cu NPs/compounds significantly reduced root length in both plant species. The shortest root in lettuce (15.9 ± 2.4 cm) and alfalfa (16.2 ± 0.2 cm) occurred in plants treated with 20 mg L−1nCuO and nCu, respectively. The reduction of root length was 49.5% ± 7.8% in lettuce and 47.6% ± 1.0% in alfalfa. Similar results have been reported for Mung bean, zucchini, and wheat.10,16,17 Lee et al.17 reported that nCu at 200 mg L−1 affected the roots of wheat, while only at 800 mg L−1 reduced wheat shoot length. Another report indicates that 10 mg nCuO/L reduced the length of root and shoot in radish seedlings by 46% and 4%, respectively.21 Moreover, 1000 mg nCuO/L, decreased the root and shoot length of radish by 97% and 79%, respectively. In our study, although the Cu concentration was high in alfalfa shoots, compared to lettuce, there was no shoot length reduction; however, bulk Cu, CuPRO 2005, Kocide 3000, and CuCl2 at 20 mg L−1, significantly reduced lettuce shoot length (ESI Fig. S2†). This suggests a species-specific response to Cu toxicity. A previous report indicated that in Vigna unguiculata, Cu caused a reduction in shoot growth because it also caused a reduction in Fe.40 In our study, almost all the Cu NPs/compounds reduced Fe in tissues of lettuce and alfalfa (iron uptake is shown in Fig. 6 and will be discussed later on). We hypothesize that differences on Cu accumulation (Fig. 3) were responsible for the different effects of Cu NPs/compounds on plant growth in both plant species. Copper in tissues triggers direct production of ROS via Fenton and induces root growth inhibition.41
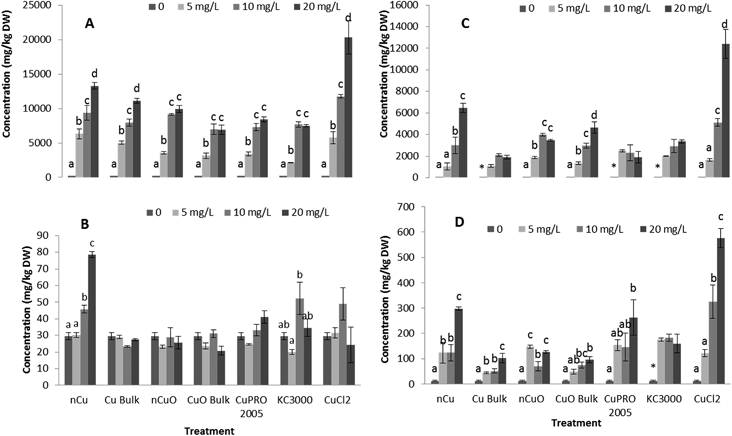 |
| Fig. 3 Copper concentration in lettuce root (A), lettuce shoot (B), alfalfa root (C), and alfalfa shoot (D) exposed for 15 days in hydroponics to 0, 5, 10, 20 mg L−1 of Cu NPs or compounds. Data are averages of four replicates ± SE. Different letters stand for statistical differences at p ≤ 0.05. * shows significant difference compared with the other treatments. | |
Uptake of Cu by alfalfa and lettuce
Fig. 3 shows Cu concentration in roots and shoots of alfalfa and lettuce hydroponically grown for 15 days with Cu NPs/compounds at 0, 5, 10, 20 mg L−1. As shown in this figure, Cu increased in roots as the treatment concentration in the medium increased. The highest Cu concentrations (20
000 mg kg−1 and 12
000 mg kg−1 for lettuce and alfalfa root, respectively) occurred in plants treated with 20 mg L−1 CuCl2, which was very likely due to the bioavailability of the Cu ions (Table S2†). Comparing the Cu uptake in roots of the two species of plants from all Cu forms, except CuCl2, it can be seen that there were plant species and treatment concentration effects (Tables S2 and S4†). In lettuce root, Cu accumulation from nCu at 5 mg L−1 and 20 mg L−1 was higher, compared to the other compounds (Table S2,†p ≤ 0.05). However, there was no clear difference in Cu accumulation in alfalfa root, at all treatment concentrations (Table S4†). Wang et al.18 reported 1.8 times more Cu in maize roots treated with nCuO compared to bulk CuO. The accumulation of Cu in alfalfa shoot followed a similar trend than in root (Fig. 3D). Except for CuPRO 2005 and bulk CuO, all compounds, at all concentrations, significantly increased Cu accumulation in alfalfa shoots, compared to control. In the case of lettuce shoot, only nCu at 10 and 20 mg L−1 significantly increased Cu accumulation, respect to control (Fig. 3B). Differences in Cu translocation from diverse Cu compounds have been previously reported. The shoots of wheat exposed to nCuO accumulated more Cu (375 mg kg−1) than plants treated with bulk CuO (254 mg kg−1).27 Perhaps the smaller size of NPs (Table S1†) allowed higher uptake and translocation within the plant. In our experiment, exposure to nCu at 20 mg L−1 produced significantly higher Cu concentration in both the lettuce shoot (Table S3†) and alfalfa shoot (Table S5†), compared to bulk Cu at 20 mg L−1. Also, under all treatments, alfalfa translocated about 3–5% of Cu from root to shoot, while only 0.5–0.6% was translocated in lettuce. This suggests the uptake and translocation of Cu is associated to the copper compound and plant species. In this study, particle size could be a significant factor influencing Cu uptake (Table S1†).
Effects of Cu NPs/compounds on nutrient elements' uptake
Under the conditions of this research, Cu NPs/compounds did not alter the uptake of Ca, Mg, Mo, Mn, Zn, and Na in lettuce and alfalfa exposed for 15 days to the Cu treatments. However, the uptake of P (Fig. 4), S (Fig. 5), and Fe (Fig. 6) was significantly affected.
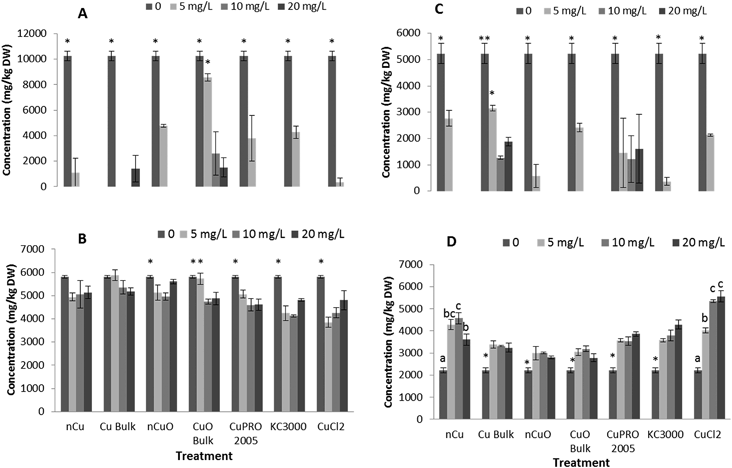 |
| Fig. 4 Phosphorus concentration in lettuce root (A), lettuce shoot (B), alfalfa root (C), and alfalfa shoot (D) exposed for 15 days in hydroponics to 0, 5, 10, 20 mg L−1 of Cu NPs/compounds. Data are averages of four replicates ± SE. Different letters stand for statistical differences at p ≤ 0.05. * shows significant difference compared with the other treatments. | |
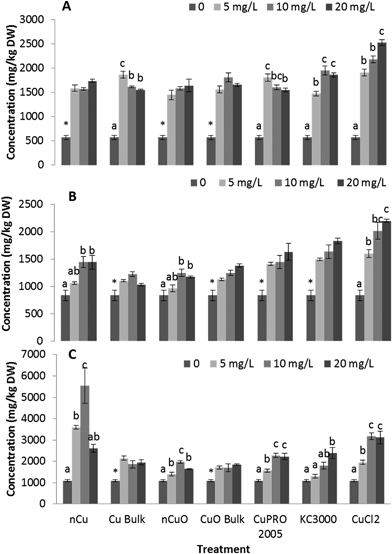 |
| Fig. 5 Sulfur concentration in lettuce root (A), alfalfa root (B), and alfalfa shoot (C) exposed for 15 days in hydroponics to 5, 10, 20 mg L−1 of Cu NPs/compounds. Data are averages of four replicates ± SE. Different letters stand for statistical differences at p ≤ 0.05. * shows significant difference compared with the other treatments. | |
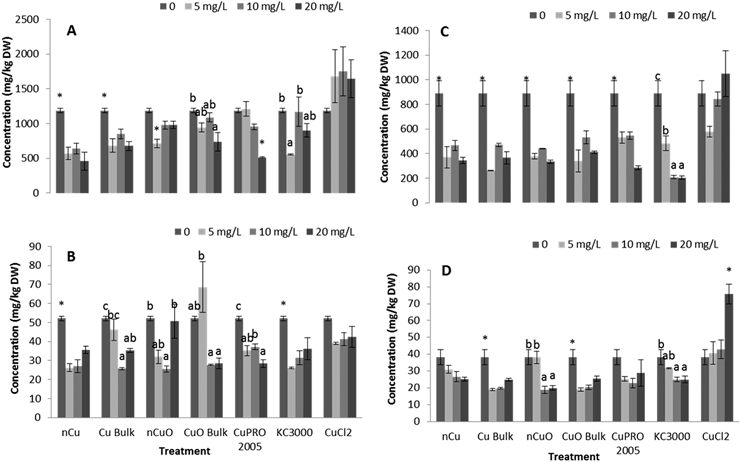 |
| Fig. 6 Iron concentration in lettuce root (A), lettuce shoot (B), alfalfa root (C), and alfalfa shoot (D) exposed for 15 days in hydroponics with 0, 5, 10, 20 mg L−1 of Cu NPs/compounds. Data are averages of four replicates ± SE. Different letters stand for statistical differences at p ≤ 0.05. * shows significant difference compared with the other treatments. | |
Phosphorus uptake.
All Cu compounds, at all concentrations, reduced P uptake in roots of both plant species (Fig. 4A and C). Moreover, P was not detected in roots of both plant species at 10 and 20 mg L−1 treatment concentrations. Similar results were reported by Ali et al.42 who found that P content decreased in root of maize exposed for 15 days to 15.7, 78.5, and 157 μM of Cu. This could be due to the formation of Cu phosphate, which cannot be taken up by plant roots43 or to damage on root cell plasmalemma, resulting in loss of ions.42 Phosphorus was also reduced in shoots of Cu treated lettuce plants (Fig. 4B) but it increased in alfalfa shoot (Fig. 4D). At 5 and 10 mg L−1 treatments, nCu increased P accumulation in alfalfa shoot, compared with bulk Cu (Table S5,†p ≤ 0.05). At 10 and 20 mg L−1 treatments, CuCl2 resulted in highest P accumulation in alfalfa shoot but lower P accumulation in lettuce shoot (Table S3,†p ≤ 0.05). Copper concentration in alfalfa shoot was much higher than in lettuce shoot (Fig. 3), which suggests different transport mechanisms. The root uptake of P from solution is controlled by specialized transporters, while the movement within plant tissues is due to other transporters.44 Pht1 transporters are specific for P acquisition from soil solution; while Pht2 transporters are responsible for P translocation to the aboveground plant parts.45 In our study, it looks like all Cu NPs/compounds, at all concentrations down-regulated Pht1 in both plant species, as the P concentration in roots was significantly lower compared to control (p ≤ 0.05). As per Fig. 4A, bulk CuO exerted lower effects on lettuce, compared to the other treatments; however, in alfalfa, lower effects were found with bulk Cu treatments (Fig. 4C). Data on Fig. 4B and D suggest that modulation of Pht2 expression was affected by the plant species and the Cu form more than by the concentration used. As seen in these figures, all compounds at all concentrations increased P in alfalfa shoots, suggesting up-regulation of Pht2 (Fig. 4D). On the other hand, P concentration in shoot of control, nCu, and bulk Cu treated lettuce plants were similar, suggesting no up-regulation of Pht2. This suggests a species specific response to excess Cu.
Sulfur uptake.
As shown in Fig. 5, all Cu treatments increased S concentration in lettuce root (Fig. 5A), alfalfa root (Fig. 5B), and alfalfa shoot (5C), but did not affect S accumulation in lettuce shoot (results not shown). There were no obvious differences in S accumulation between NPs and bulk material treatments in lettuce root. However, highest S accumulation was found in alfalfa root treated with the highest concentration of CuCl2. In alfalfa shoot, CuPRO 2005 and CuCl2 at 10 and 20 mg L−1 exhibited the highest S accumulation, except for nCu at 10 mg L−1. At 5 and 10 mg L−1 treatments, nCu increased S accumulation in alfalfa shoot, compared to the other treatments (Table S5,†p ≤ 0.05). Exposure of plants to toxic metals may affect the uptake and assimilation of S due to the formation of sulfur-rich phytochelatins and metallothioneins.46,47 According to another study,48 plants increase S uptake to mitigate Cu toxicity; but Cu could also induce over expression of sulfate transporters by affecting the signal transduction pathway, resulting in higher S accumulation. As previously reported, copper activates the biosynthesis of phytochelatins and interferes with S accumulation.49 In Chinese cabbage, high Cu concentration up-regulated activity of sulphate transporters Sultr1; 2 and Sultr2; 2 in the roots; however, only the shoots showed increased in sulfate.49 Very likely, these and other members of the sulfate transporter family were activated in lettuce and alfalfa. However, the fact that S did not increase in lettuce shoots suggests no up-regulation of Sultr2; 2 in this plant,45 as this transporter “is localized specifically in the phloem of roots and in vascular bundle sheath cells of leaves.”50
Iron uptake.
As shown in Fig. 6, except for CuCl2, all the Cu treatments significantly reduced Fe concentration in shoots and roots of alfalfa and lettuce. CuPRO 2005 and Kocide 3000 at 20 mg L−1 caused the lowest Fe accumulation in the shoot of lettuce (512 ± 10 mg kg−1) and alfalfa (203 ± 13 mg kg−1), respectively. It is possible that Fe, which is more active than Cu, formed insoluble hydroxides that were retained in roots.51 CuCl2 did not change Fe concentration in both plants, except at 20 mg L−1 in alfalfa shoot. Previous studies have shown that copper sulfate can cause Fe deficiency in rice (Oryza sativa L.) and beans (Phaseolus vulgaris L.).52 Patsikka et al.53 also reported that the Cu treatment reduced leaf chlorophyll concentration and the thylakoid membrane network in beans. However, the adverse effects were reverted by adding excess Fe. Ouzounidou et al.54 reported that the increase in Fe concentration in spinach leaves reduced the toxic effects of Cu on photosynthesis. In addition, Schmidt et al.55 stated that “Cu can displace Fe from chelating molecules in the growth medium, inhibiting the induction of Fe stress response.” This suggests that Cu and Fe could share the same uptake pathway.56
Enzyme activities
After entering root cells, CuNPs/compounds and the released Cu ions may cause the formation of reactive oxygen species (ROS), changing the activity of stress enzymes. Fig. 6 shows the activity of CAT in alfalfa tissues (there was no effect on CAT in lettuce tissues) and APX in lettuce and alfalfa root (there was no effect on shoot APX activity in both plant species). As seen in this figure, all Cu treatments, at all concentrations, significantly reduced CAT activity in alfalfa shoot, compared to control (Fig. 7A). The highest reduction was found with nCuO at 10 mg L−1. A similar trend was observed in alfalfa root where the most striking results were produced by CuPRO 2005 and bulk CuO at 5 mg L−1 (Fig. 7B). At 10 mg L−1 bulk Cu, nCuO, and Kocide 3000 did not reduce CAT activity in alfalfa root, compared to control; while at 20 mg L−1, all the treatments, except nCuO, significantly reduced CAT activity, compared to control. Kim et al.26 also reported that nCuO at 500 and 1000 mg L−1 increase CAT activity in Cucumis sativus. In our study, CaCl2 imposed a concentration-dependent reduction on alfalfa root CAT activity, which at 20 mg L−1 reduced it by 87% (Fig. 7B). Hou et al.24 reported that Cu2+ at 5 mg L−1 increased CAT in duckweed, but at 10 mg L−1 inhibited CAT activity. This suggests the effects of Cu on CAT activity are associated to the plant species and the form of Cu.
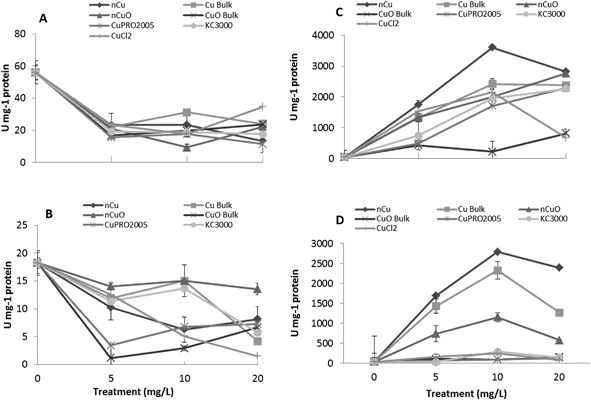 |
| Fig. 7 Antioxidant enzyme activity in alfalfa and lettuce treated for 15 days in hydroponics with 0, 5, 10, 20 mg L−1 Cu of NPs/compounds. CAT activity in alfalfa shoot (A) and alfalfa root (B); APX activity in lettuce root (C) and alfalfa root (D). Values are averages of four replicates ± SE. CAT activity in lettuce tissues and APX activity in shoots of both plant species were not affected. | |
The APX activity in the roots of lettuce (Fig. 7C) and alfalfa (Fig. 7D) showed contrasting results, compared to CAT activity in alfalfa root (Fig. 7B). At all concentrations, all Cu treatments significantly increased APX activity in lettuce root, compared to control, except for bulk CuO (Fig. 7C). In lettuce root (Fig. 7C), CuCl2 at 20 mg L−1 had lower impact on APX activity, compared to 10 mg L−1, but still the difference was significant (p ≤ 0.05), compared to control. In alfalfa root (Fig. 7D), APX activity significantly increased at all Cu treatments, except with both Cu(OH)2 compounds. Notoriously, in alfalfa root, four compounds at 20 mg L−1 presented a decrease in APX activity; however, the activity was still significantly higher, compared to control. Similar results were reported in Brassica juncea exposed to Cu;57 but in duckweed, it was found that APX activity was inhibited by Cu at 0.25 μM.25 These results suggest that, to some extent, in lettuce and alfalfa roots, CAT and APX are down regulated and up regulated, respectively, by the same external stimulus, in this case the Cu treatment. Additionally, in both plants species, the highest APX activity was recorded with Cu NPs, which indicates this form of Cu induces the production of more ROS in lettuce plants.
In summary, this study has shown that all Cu treatments, even at low concentration (5 mg L−1), reduced root length in hydroponically grown lettuce and alfalfa. At high concentration (20 mg L−1), both plant species absorbed more Cu when they were treated with nCu than bulk Cu. Cu NPs/compounds reduced P and Fe accumulation in plant tissues, while S concentration increased in alfalfa shoot and in the roots of both plant species. The activity of stress enzyme CAT was not affected in lettuce and sharply reduced in alfalfa shoot. The APX activity in lettuce and alfalfa roots increased at all Cu treatments, except for bulk Cu in lettuce and Cu(OH)2 in alfalfa root.
Acknowledgements
This material is based upon work supported by the National Science Foundation and the Environmental Protection Agency under Cooperative Agreement Number DBI-0830117. Any opinions, findings, and conclusions or recommendations expressed in this material are those of the author(s) and do not necessarily reflect the views of the National Science Foundation or the Environmental Protection Agency. This work has not been subjected to EPA review and no official endorsement should be inferred. The authors also acknowledge the USDA grant number 2011-38422-30835 and the NSF Grant # CHE-0840525. This work was also supported by Grant 2G12MD007592 from the National Institutes on Minority Health and Health Disparities (NIMHD), a component of the National Institutes of Health (NIH). J. L. Gardea-Torresdey acknowledges the Dudley family for the Endowed Research Professorship and the Academy of Applied Science/US Army Research Office, Research and Engineering Apprenticeship program (REAP) at UTEP, grant #W11NF-10-2-0076, sub-grant 13-7.
References
- ASTM, Standard Terminology Relating to Nanotechnology, http://www.astm.org/Standards/E2456.htm.
- S. J. Klaine, P. J. Alvarez, G. E. Batley, T. F. Fernandes, R. D. Handy, D. Y. Lyon, S. Mahendra, M. J. McLaughlin and J. R. Lead, Environ. Toxicol. Chem., 2008, 27, 1825–1851 CrossRef CAS
.
- J. R. Peralta-Videa, L. Zhao, M. L. Lopez-Moreno, G. de la Rosa, J. Hong and J. L. Gardea-Torresdey, J. Hazard. Mater., 2011, 186, 1–15 CrossRef CAS PubMed
.
- P. Biswas and C.-Y. Wu, J. Air Waste Manage. Assoc., 2005, 55, 708–746 CAS
.
- H. Chen, J. Weiss and F. Shahidi, Food Tech., 2006, 60, 30–36 CAS
.
- A. Maynard, R. J. Aitken, T. Butz, V. Colvin, K. Donaldson, G. Oberdörster, M. A. Philbert, J. Ryan, A. Seaton and V. Stone, Nature, 2006, 444, 267 CrossRef CAS PubMed
.
- W. Center, Nanotech-Project Consumer Product Analysis, http://www.nanotechproject.org/inventories/consumer/analysis_draft/.
- A. A. Keller, S. McFerran, A. Lazareva and S. Suh, J. Nanopart. Res., 2013, 15, 1–17 CrossRef
.
- C. Buzea, I. I. Pacheco and K. Robbie, Biointerphases, 2007, 2, MR17–MR71 CrossRef
.
- D. Stampoulis, S. K. Sinha and J. C. White, Environ. Sci. Technol., 2009, 43, 9473–9479 CrossRef CAS PubMed
.
- J.-g. Yang, T. Okamoto, R. Ichino, T. Bessho, S. Satake and M. Okido, Chem. Lett., 2006, 35, 648–649 CrossRef CAS
.
- A. G. Nasibulin, P. P. Ahonen, O. Richard, E. I. Kauppinen and I. S. Altman, J. Nanopart. Res., 2001, 3, 383–398 CrossRef
.
- J. Zhu, D. Li, H. Chen, X. Yang, L. Lu and X. Wang, Mater. Lett., 2004, 58, 3324–3327 CrossRef CAS PubMed
.
- I. Blinova, A. Ivask, M. Heinlaan, M. Mortimer and A. Kahru, Environ. Pollut., 2010, 158, 41–47 CrossRef CAS PubMed
.
- V. Shah and I. Belozerova, Water, Air, Soil Pollut., 2009, 197, 143–148 CrossRef CAS
.
- C. Musante and J. C. White, Environ. Toxicol., 2012, 27, 510–517 CrossRef CAS PubMed
.
- W. M. Lee, Y. J. An, H. Yoon and H. S. Kweon, Environ. Toxicol. Chem., 2008, 27, 1915–1921 CrossRef CAS
.
- Z. Wang, X. Xie, J. Zhao, X. Liu, W. Feng, J. C. White and B. Xing, Environ. Sci. Technol., 2012, 46, 4434–4441 CrossRef CAS PubMed
.
- C. O. Dimkpa, D. E. Latta, J. E. McLean, D. W. Britt, M. I. Boyanov and A. J. Anderson, Environ. Sci. Technol., 2013, 47, 4734–4742 CrossRef CAS PubMed
.
- J. Shi, A. D. Abid, I. M. Kennedy, K. R. Hristova and W. K. Silk, Environ. Pollut., 2011, 159, 1277–1282 CrossRef CAS PubMed
.
- D. H. Atha, H. Wang, E. J. Petersen, D. Cleveland, R. D. Holbrook, P. Jaruga, M. Dizdaroglu, B. Xing and B. C. Nelson, Environ. Sci. Technol., 2012, 46, 1819–1827 CrossRef CAS PubMed
.
- S. S. Gill and N. Tuteja, Plant Physiol. Biochem., 2010, 48, 909–930 CrossRef CAS PubMed
.
- C. H. Foyer and S. Shigeoka, Plant Physiol., 2011, 155, 93–100 CrossRef CAS PubMed
.
- W. Hou, X. Chen, G. Song, Q. Wang and C. Chi Chang, Plant Physiol. Biochem., 2007, 45, 62–69 CrossRef CAS PubMed
.
- H. Teisseire and V. Guy, Plant Sci., 2000, 153, 65–72 CrossRef CAS
.
- S. Kim, S. Lee and I. Lee, Water, Air, Soil Pollut., 2012, 223, 2799–2806 CrossRef CAS
.
- C. O. Dimkpa, J. E. McLean, D. E. Latta, E. Manangón, D. W. Britt, W. P. Johnson, M. I. Boyanov and A. J. Anderson, J. Nanopart. Res., 2012, 14, 1125–1129 CrossRef
.
- K. Giannousi, I. Avramidis and C. Dendrinou-Samara, RSC Adv., 2013, 3, 21743–21752 RSC
.
- R. J. Worthington, J. J. Richards and C. Melander, Org. Biomol. Chem., 2012, 10, 7457–7474 CAS
.
- K. E. Hendricks, R. S. Donahoo, P. D. Roberts and M. C. Christman, Am. J. Plant Sci., 2013, 4, 282 CrossRef
.
- G. Puopolo, A. Cimmino, M. C. Palmieri, O. Giovannini, A. Evidente and I. Pertot, J. Appl. Microbiol., 2014, 117, 1168–1180 CrossRef CAS PubMed
.
-
A. A. Hanson, D. Barnes and R. Hill Jr, Alfalfa and alfalfa improvement, American Society of Agronomy, Crop Science Society of America, Soil Science Society of America, 1988 Search PubMed
.
- A. S. Adeleye, J. R. Conway, T. Perez, P. Rutten and A. A. Keller, Environ. Sci. Technol., 2014, 48, 12561–12568 CrossRef CAS PubMed
.
- A. S. Adeleye and A. A. Keller, Water Res., 2014, 49, 236–250 CrossRef CAS PubMed
.
- A. S. Adeleye, A. A. Keller, R. J. Miller and H. S. Lenihan, J. Nanopart. Res., 2013, 15, 1–18 CrossRef
.
- J. Peralta, J. Gardea-Torresdey, K. Tiemann, E. Gomez, S. Arteaga, E. Rascon and J. Parsons, Bull. Environ. Contam. Toxicol., 2001, 66, 727–734 CAS
.
- G. Carrillo-Castañeda, J. Juárez Muños, J. Peralta-Videa, E. Gomez, K. Tiemannb, M. Duarte-Gardea and J. Gardea-Torresdey, Adv. Environ. Res., 2002, 6, 391–399 CrossRef
.
- S. M. Gallego, M. P. Benavídes and M. L. Tomaro, Plant Sci., 1996, 121, 151–159 CrossRef CAS
.
- Y. Nakano and K. Asada, Plant Cell Physiol., 1981, 22, 867–880 CAS
.
- T. R. Northfield, A. R. Sheldon, P. M. Kopittke and N. W. Menzies, Plant Soil, 2011, 342, 359–367 CrossRef CAS
.
- T. Remans, S. Thijs, S. Truyens, N. Weyens, K. Schellingen, E. Keunen, H. Gielen, A. Cuypers and J. Vangronsveld, Ann. Bot., 2012, 110, 239–252 CrossRef CAS PubMed
.
- N. A. Ali, M. P. Bernal and M. Ater, Plant Soil, 2002, 239, 103–111 CrossRef CAS
.
- S. Ziemniak, M. Jones and K. Combs, J. Solution Chem., 1992, 21, 1153–1176 CrossRef CAS
.
- D. P. Schachtman, R. J. Reid and S. M. Ayling, Plant Physiol., 1998, 116, 447–453 CrossRef CAS PubMed
.
- P. Buchner, H. Takahashi and M. J. Hawkesford, J. Exp. Bot., 2004, 55, 1765–1773 CrossRef CAS PubMed
.
- H. Rouached, D. Secco and A. B. Arpat, J. Plant Physiol., 2009, 166, 893–902 CrossRef CAS PubMed
.
- W. H. Ernst, G. J. KRAUSS, J. A. Verkleij and D. Wesenberg, Plant, Cell Environ., 2008, 31, 123–143 CAS
.
- M. Shahbaz, M. Hwei Tseng, C. E. E. Stuiver, A. Koralewska, F. S. Posthumus, J. H. Venema, S. Parmar, H. Schat, M. J. Hawkesford and L. J. De Kok, J. Plant Physiol., 2010, 167, 438–446 CrossRef CAS PubMed
.
- M. Shahbaz, C. Stuiver, F. Posthumus, S. Parmar, M. Hawkesford and L. Kok, Plant Biol., 2014, 16, 68–78 CrossRef CAS PubMed
.
- H. Takahashi, A. Watanabe-Takahashi, F. W. Smith, M. Blake-Kalff, M. J. Hawkesford and K. Saito, Plant J., 2000, 23, 171–182 CrossRef CAS
.
- J. Trujillo-Reyes, S. Majumdar, C. E. Botez, J. R. Peralta-Videa and J. L. Gardea-Torresdey, J. Hazard. Mater., 2014, 267, 255–263 CrossRef CAS PubMed
.
- N. K. Fageria, Pesqui. Agropecu. Bras., 2002, 37, 1765–1772 CrossRef PubMed
.
- E. Pätsikkä, M. Kairavuo, F. Šeršen, E.-M. Aro and E. Tyystjärvi, Plant Physiol., 2002, 129, 1359–1367 CrossRef PubMed
.
- G. Ouzounidou, I. Ilias, H. Tranopoulou and S. Karataglis, J. Plant Nutr., 1998, 21, 2089–2101 CrossRef CAS
.
- W. Schmidt, M. Bartels and C. FÜHNER, New Phytol., 1997, 135, 659–666 CAS
.
- R. Welch, W. Norvell and S. Schaefer, Planta, 1993, 190, 555–561 CrossRef CAS
.
- R. Pantola and G. Shekhawat, J. Pharm. Biomed. Sci., 2012, 15, 1–6 Search PubMed
.
Footnote |
† Electronic supplementary information (ESI) available. See DOI: 10.1039/c4em00551a |
|
This journal is © The Royal Society of Chemistry 2015 |
Click here to see how this site uses Cookies. View our privacy policy here.