DOI:
10.1039/C3BM60109A
(Paper)
Biomater. Sci., 2013,
1, 1166-1171
Surface properties of spider silk particles in solution†
Received 27th April 2013, Accepted 7th July 2013
First published on 25th July 2013
Abstract
Recombinant spider silk proteins, such as eADF4(C16), can be used for various applications. Colloidal particles of eADF4(C16) show potential as drug delivery systems. Tuning the colloidal properties of suspensions of eADF4(C16) particles represents a major prerequisite for their use in pharmaceutical formulations. In this study we determined the surface properties concerning inter-particle interactions by means of electrophoretic mobility and direct force measurements. The surface charge of eADF4(C16) spider silk particles was determined as a function of ionic strength and pH, respectively. The resulting electrophoretic mobility can be described using the O'Brien and White theory and is directly related to the amino acid sequence of the protein. We determined the extension of a fuzzy protein layer protruding into the solution by direct force measurements using a colloidal probe technique. This soft layer leads to deviations in the electrophoretic mobility and is responsible for additional repulsive forces at small separation distances. These steric forces lead to a stabilization of the particle suspension at high ionic strength.
Introduction
Spider silk is known to combine properties such as biocompatibility and mechanical strength.1 Recombinant spider silk production provides spider silk proteins in large quantities and enables the development of silk-based materials for a large number of applications.2,3 In addition to the naturally occurring spider silk fibers, recombinant spider silk proteins can be assembled into various other shapes, such as films and particles, being particularly useful for distinct drug delivery applications.3–6One prerequisite for applying spider silk particles in pharmaceutical formulations is the stability of the colloidal suspensions, e.g. over a wide range of electrolyte concentrations.4 A rational approach to tune the properties of spider silk particles for specific applications is based on the control of the resulting inter-particle forces and colloidal properties such as surface charge, roughness or mechanical properties. Here, we resolved the surface properties of spider silk particles made of the recombinant spider silk protein eADF4(C16) by their electrophoretic mobility as well as by direct force measurements. We further demonstrate that the colloidal properties of the spider silk particles are directly related to the amino-acid sequence of the underlying eADF4(C16).
Results and discussion
Amino acid composition and surface properties of spider silk particles
eADF4(C16) spider silk particles (Fig. 1a) were obtained by salting out the protein using potassium phosphate as described previously.5 As shown in Scheme 1, one eADF4(C16) molecule contains 16 glutamic acid residues (one per C-module), which represent the only functional groups dissociated at a moderate pH value, as their pKa is around 4.3. The termini of eADF4(C16) provide one amino- and carboxyl-group, respectively. However, these contribute to a much smaller extent to the charging behaviour due to their highly acidic/basic pKa values and their low occurrence. The methionine residue at the amino-terminus is most likely post-translationally removed in E. coli, resulting in a terminal alanine residue that has a similar pKa value.7 Each C-module also contains two tyrosine residues (32 in total per eADF4(C16)), with a relatively high pKa, and thus tyrosine residues are only of importance at very basic pH values. Furthermore, the T7-Tag contains one arginine residue with an extremely high pKa, which has been neglected in the following as it is not in the examined pH-range.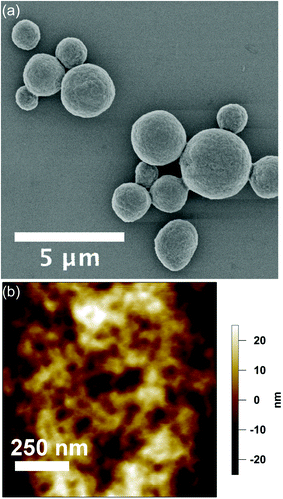 |
| Fig. 1 (a) SEM-image of eADF4(C16) particles in the dehydrated state. (b) Surface topography acquired at the apex of an eADF4(C16) particle in solution by AFM in TappingMode. | |
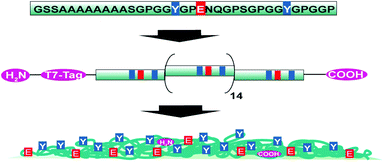 |
| Scheme 1 Schematic representation of the consensus sequence of ADF4, the engineered recombinant eADF4(C16) and the particle surface distribution of selected amino acids. | |
Table 1 compiles the ionizable entities in eADF4(C16) with their relative occurrence per module and the corresponding pKa. The pKa of the surface can be approximated by the pKa for the isolated amino acids8 due to their low occurrence per molecule, which results in a large separation between ionizable groups. From their occurrence per protein one can directly infer in a first order approximation the surface coverage Γ (in groups per area) of the spider silk particle. This approximation assumes that the surface composition of the spider silk particles does not deviate significantly from the composition per molecule and that the amino acids occupy comparable volumes. Its validity is experimentally directly accessible by determining the electrophoretic mobility of the particles.
Table 1 Ionizable groups of eADF4(C16)
| C-module | | C-terminus | N-terminus | T7-Tag |
---|
Amino acid | Glutamic acid E | Tyrosine Y | Glycine G | Methionine M | Arginine R |
pKa | 4.25 | 10.07 | 2.34 | 9.21 | 12.48 |
Groups/molecule | 16 | 32 | 1 | 1 | 1 |
Hydropathy index | −3.5 | −1.3 | −0.4 | 1.9 | −4.5 |
Γtheo [nm−1] | 0.0625 | 0.125 | 0.0039 | 0.0039 | 0.0039 |
Γmob [nm−1] | 0.06 ± 0.02 | 0.12 ± 0.04 & (0.03 ± 0.01) | 0.004 ± 0.001 | 0.004 ± 0.001 | 0 |
Dehydrated particles (Fig. 1a) have an average diameter of 2.1 ± 0.8 μm, which increases upon hydration with a volume-swelling factor of 2.3.9 The resulting particle diameter of 2.8 μm is used in the following. The particle morphology remains relatively constant over one batch and is shown in detail for the dried state in the ESI.† The particle surface retains its smooth topography in solution with a root mean square (rms) roughness of 17.3 ± 4.3 nm as obtained by TappingMode AFM in liquid (Fig. 1b).
Electrophoretic mobility
Fig. 2a shows the electrophoretic mobility at two different ionic strengths (i.e. 1 mM and 10 mM, respectively) as a function of pH. As expected from the amino acid composition and the pKa, the negative value of the electrophoretic mobility increases with increasing pH yielding a plateau above pH 8. At neutral pH no mobilities could be determined due to the ill-defined pH-values on the time scale of the mobility measurements in this regime. The experimental data can be readily compared to calculations based on the theory of O'Brien and White10 and a surface composition as given by Γmob in Table 1. In contrast to the Debye–Hückel and Helmholtz–Smoluchowski equations, normally used to relate ζ-potential and electrophoretic mobility, the O'Brien and White theory takes the relaxation effect of the surrounding ion cloud into account.10,11 Therefore, we varied Γmob by about ±30%, as indicated by the coloured area in Fig. 2, to allow calculations for a variation of the surface composition of eADF4(C16) particles in respect to the properties of single eADF4(C16) molecules. Furthermore, the surface coverage of the tyrosine residues could be significantly lower at the surface due to steric contributions and a more positive hydropathy index, which has been taken into consideration by the values in brackets in Table 1. The experimental data are in very good agreement with the theoretical calculations, especially at acidic pH. The observed charge reversal is in agreement with the theoretical isoelectric point of 3.48 for single eADF4(C16) molecules.2 The plane of shear, determining when hydrodynamic drag acts on the ion layer, has been chosen as 0.25 nm in agreement with comparable studies.12,13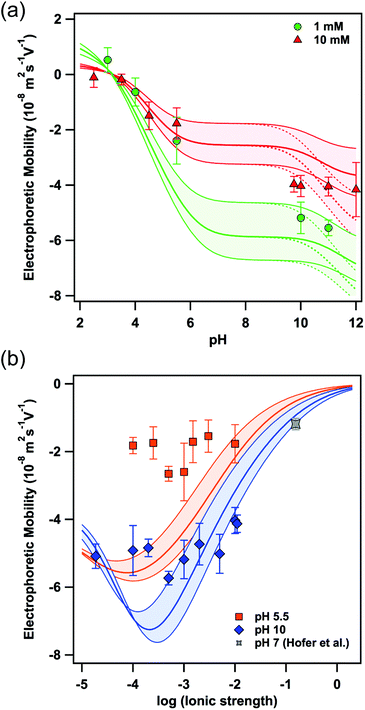 |
| Fig. 2 Electrophoretic mobility measured as a function of (a) pH and (b) ionic strength. The lines represent the calculation according to the O'Brien and White theory with a constant eADF4(C16) particle radius of 2.8 μm and a surface composition according to Γmob in Table 1, where the colored areas include the upper and lower limits for Γmob, respectively. The gray data point has been reported by Hofer et al. and represents the electrophoretic mobility in an electrolyte solution resembling physiological conditions in terms of ionic strength and pH.6 | |
In order to validate the applicability of the O'Brien and White theory to describe the electrophoretic mobility, we next varied the ionic strength at constant pH (Fig. 2b). The minima in the electrophoretic mobility disagree with the Smoluchowsky equation predicting a monotonic decrease of the absolute values for the electrophoretic mobility with increasing ionic strength (at constant pH). The existence of such minima in mobility as a function of ionic strength has been reported previously, albeit for hard colloidal particles made of latex or silica.12–14 For spider silk particles the minima in mobility occur at ionic strengths as predicted by the calculations according to the O'Brien and White theory10 and the values in Table 1. However, the absolute value of the mobilities is somewhat lower than calculated. As this effect is more pronounced at low pH, we assume this deviation to result from a diffuse protein layer, i.e. a soft interface on the spider silk particles, which leads to the diffuse plane of shear and lower mobility as pointed out by Ohshima and others.15 Analogous effects can be observed for other macromolecules.16 For applications in drug delivery the mobility under physiological conditions is relevant. Corresponding measurements of electrophoretic mobility for analogous spider particles have been reported previously.6 The resulting electrophoretic mobilities can be described as well in the framework of the here-presented model (cf. Fig. 2b and ESI†).
Direct force measurements
In order to experimentally corroborate the presence of such a diffuse protein layer on the surface of eADF4(C16) spider silk particles, we determined their interfacial properties by direct force measurements using a colloidal probe. Spider silk particles were immobilized on a silane-modified glass surface together with colloidal silica particles from the same batch as used for the colloidal probe (Fig. 3a). The silica particles served as “internal standards” for an incompressible surface and allowed to additionally determine the diffuse layer properties of the colloidal probe during the measurements on a well-known surface in situ.17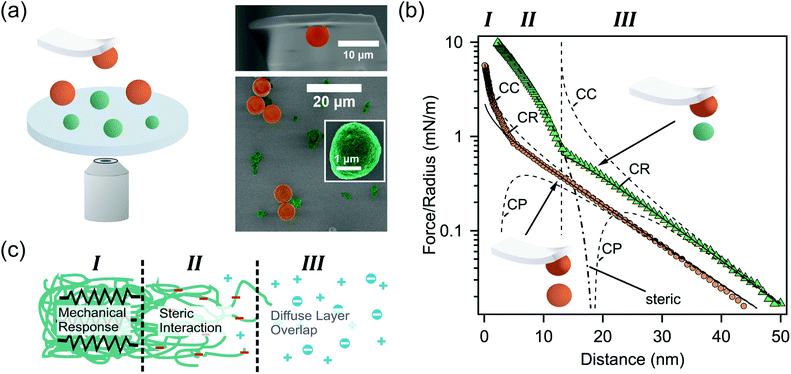 |
| Fig. 3 (a) Schematic representation of direct force measurements (left side). A colloidal silica probe and the sample with immobilized silica (orange) and eADF4(C16) particles (green) are additionally shown in SEM-images (right panel). (b) Representative force profiles for the interaction between silica (orange) and eADF4(C16) (green) particles, respectively. The diffuse layer overlap has been fitted according to the full solutions of the Poisson–Boltzmann equation with classical boundary conditions of constant charge (CC) and constant potential (CP), as well as the constant regulation approximation (CR). (c) Different contributions to the force profiles in dependence of the separation for the colloidal probe versus the eADF4(C16) particles. | |
Fig. 3b shows the interaction force profile between a colloidal silica probe and a silica particle (immobilized on the substrate) at pH 5.5 and an ionic strength of I = 1 mM. The interaction forces have been normalized to the effective radius of the sphere–sphere geometry (cf. eqn (1) in the experimental methods).18,19 The interaction force profile is based on the overlap of diffuse layers originating from the silica particles. The interaction is quantitatively evaluated by fits using the full solutions of the Poisson–Boltzmann equation including the classical boundary conditions of constant charge (CC) and constant potential (CP), as well as charge regulation in the constant regulation approximation (CR).20 The latter accurately describes experimental data over nearly the whole interaction range. The resulting decay length at large separation distances is κ−1 = 10.6 nm and corresponds to the Debye-length calculated from the nominal ionic strength I within reasonable accuracy. Assuming two identical silica particles, one obtains a diffuse layer potential of ψSiOx = −46.5 mV in agreement with the values reported in the literature.18,19,21 Nevertheless, heat treatment of silica particles is known to have an influence on their surface chemistry, resulting in higher diffuse layer potentials.22 We attribute the additional repulsive forces at small separation distances (i.e. <5 nm) to soluble protein originating from the eADF4(C16) particles deposited on the sample.
The interaction profile between the colloidal probe and eADF4(C16) particles is more complex than the one between the silica particles, since different interactions occur as a function of separation distance. At large separations the interaction forces result primarily from diffuse layer overlaps and are due to charges present on the surface of the eADF4(C16) particles. In this regime (i.e. separations >10–15 nm and region III as indicated in Fig. 3c) the decay length is identical to the one for the silica–silica interaction, leading to parallel force profiles. Due to the intrinsic surface roughness of the eADF4(C16) particles (cf. Fig. 1b) the charge distribution is more complicated, and one obtains generally smaller diffuse layer potentials.23,24 Here, we obtained a diffuse layer potential of ψ = −23.8 mV for the eADF4(C16) particles, which is compatible with the electrophoretic mobility at pH 5.5 and 1 mM ionic strength. However, this potential is based on defining a plane of charge located at a separation of 13 nm (cf. the dashed vertical line in Fig. 3b), coinciding with the onset of an additional repulsive force attributed to primarily steric interactions. These steric forces result from the complex and fuzzy surface structure of the particles. However, they can be described in an approximate manner by an Alexander–deGennes type of interaction between polymer brushes (cf. the dash-point line in Fig. 3b and region II in Fig. 3c).25 Based on our fits, an extension of 30–50 nm was obtained from the force profile, which is approximately in the same regime as the measured rms-roughness using TappingMode in liquid.
A further increase in force leads to an elastic deformation of the entire particle and results from the overall elasticity of the soft particles, which is examined in more detail elsewhere.9 The onset for this regime of elastic compression (cf. region I in Fig. 3b and c) occurs at contact in the force profile. No variation of the elastic behaviour could be observed by varying the idle time (i.e. dwell time away from the surface) between different force cycles. The interfacial spring constant for the eADF4(C16) particles in solution was 2.7 N m−1 obtained by comparing the cantilever response on the hard silica particle and the soft eADF4(C16) particles (cf. eqn (2) in the Experimental section). This interfacial spring constant is in agreement with the elastic modulus of approximately 3.0 MPa determined by compression experiments over a significantly larger force regime.9
Conclusions
Both electrophoretic mobility and direct force measurements allow us to propose a model for eADF4(C16) particles as schematically depicted in Fig. 3c: a fuzzy, interfacial layer of protruding eADF4(C16) strands has an extension of about 30–50 nm and varies within a batch, being most likely responsible for the decrease in magnitude of electrophoretic mobilities upon longer storage times (several months) accompanied by the disappearance of the local minimum in Fig. 2b. The variability of this interfacial layer allows a transition of a primarily hard particle (with a thin layer) to an increasingly soft particle permeable for ions, whose electrophoretic mobility would have to be described in the framework of the Ohshima-theory.15 This interpretation is in agreement with the identified increase in size of eADF4(C16) particles upon prolonged storage.6 The steric forces caused by this layer would explain the detected colloidal stability of spider silk particles at high ionic strength or under physiological conditions.4,6Spider silk particles have a great potential for drug delivery applications. However, their surface properties have to be tunable in a defined manner to adapt to different administration and stability of the colloidal suspensions. We could demonstrate that the overall inter-particle long-range interaction forces are based on two contributions, namely electrostatic and steric forces. The long-range electrostatic interaction results from the overlap of diffuse layers and is, thus, directly related to the protein sequence. In order to influence this parameter, the protein sequence can be tuned. The large diffuse layer potentials determined for eADF4(C16) particles indicate one charged group per C-module to be efficient to provide sufficiently strong inter-particle forces for electrostatic stabilization in solution in combination with the steric interaction forces.
Materials and methods
Formation of eADF4(C16) particles
The recombinant spider silk protein eADF4(C16) was produced and purified as described previously.2 For protein precipitation, eADF4(C16) in 10 mM Tris (hydroxymethyl)–aminomethane–HCl (Tris-buffer), pH 8 at a concentration of 6 mg mL−1 was dialyzed against 1 M potassium phosphate (pH 8) for 45 min at room temperature using a dialysis membrane with a molecular weight cut-off of 6000–8000 Da (Spectra/Por, Rancho Dominuez, CA, USA). After particle formation, the suspension was centrifuged for 15 min at 17
000g followed by three washing steps with Milli-Q water.Particle immobilization
Circular glass slides for the AFM-cell (Borofloat, Irlbacher Blickpunkt Glas GmbH, Schönsee, Germany) were cleaned by sonication in a 2% Hellmanex III (Hellma, Müllheim, Germany) solution at 40 °C, followed by sonication in a mixture of isopropanol and water (v/v = 3
:
1) at room temperature. Afterwards they were treated with a modified RCA-cleaning procedure consisting of a mixture of Milli-Q water, hydrogen peroxide and ammonia (v/v/v = 5
:
1
:
1) at 80 °C for 20 min.26 The substrates were then thoroughly rinsed with Milli-Q water and dried in a nitrogen stream. Directly before silanization with 3-aminopropyldiisopropylethoxysilane (ABCR, Karlsruhe, Germany) the glass substrates were treated with O2-plasma. Silanization was carried out from the gas phase in a desiccator for at least 8 h. After silanization, the substrates were rinsed with ethanol and tempered at 80 °C for 2 h. Suspensions of silica particles (d = 6.8 μm, Bangs Laboratories Inc., Fishers, IN, USA) and eADF4(C16) were drop-cast on these amino-functionalized glass slides and dried. Then, the samples were allowed to equilibrate in the electrolyte solution before the direct force measurements for 2 h.AFM imaging of eADF4(C16) particles
The surface topography of the silk particles was determined by TappingMode in liquid on an AFM MFP-3D (Asylum Research, CA). Double-beam silicon nitride cantilevers (SNL-10, Bruker) with a nominal resonance frequency of 56 kHz and a nominal spring constant of 0.24 N m−1 were used for imaging in TappingMode.Scanning electron microscopy (SEM)
The particle suspension was placed on cleaned silicon wafers and air-dried. The samples were sputtered with 1.3–2.6 nm thick layers of platinum (Sputter coater 208 HR, Cressington). The SEM-measurements (Leo 1530 VP Gemini, Zeiss) were performed at 3.00 kV.Electrophoretic mobility
The electrophoretic mobility was determined by video microscopy on the single particle level. The measurements were carried out using a ZetaView PMX 100 (ParticleMetrix GmbH, Meerbusch, Germany) equipped with a laser scattering microscope allowing direct observation of the migrating particles. The electrophoretic mobilities have been calculated according to the theory of O'Brien and White based on the algorithm by Hunter.13,27Preparation of colloidal probes
For the preparation of colloidal probes tipless AFM-cantilevers from silicon were used (NSC12 with no coating, Mikromasch), which had a nominal spring constant of 0.3 N m−1. A recently reported procedure for the sintering of colloidal silica particles has been used in a modified form.28 The AFM-cantilevers were cleaned by rinsing with Milli-Q water and ethanol. After drying, they were treated with oxygen plasma. A diluted suspension of Ludox silica particles (Ludox AM 30, Sigma-Aldrich) was mixed with an UV-curable glue (Norland Optical Adhesives No. 63, Norland Products, Cranbury, NJ, USA). A μm-sized drop of this mixture was placed on the end of a tipless cantilever using a micromanipulator (Märzhäuser, DC-3 KS, Wetzlar, Germany) and an etched tungsten wire. Afterwards μm-sized colloidal particles from the same batch as used for sample preparation were placed on top of this glue-drop by means of another etched wire. Finally, the cantilever with the immobilized colloidal particle was treated in a muffle furnace at 1250 °C for 2 h.Direct force measurements
Interaction forces were measured by the colloidal probe technique on a closed-loop AFM (MFP-3D, Asylum Research, CA). These measurements were carried out in sphere–sphere geometry in a semi-closed fluid cell.18 The particles were coarsely aligned by optical microscopy. Then, force maps were used to determine the apex of the immobilized particle with a resolution of less than 50 nm. For each particle combination about 50 force distance curves were acquired with a velocity of 800 nm s−1 and loading forces of approximately 15–20 nN. The spring constant of the cantilevers was determined by the thermal noise method.29 The raw data were converted according to previously described procedures.18 In particular, the data sets for each particle combination were averaged and normalized by the effective radius |  | (1) |
Here, R1 (i.e. colloidal probe) was determined by optical microscopy and R2 (i.e. immobilized particles) from height data acquired in the force maps and optical micrographs, respectively. The diffuse layer potentials were fitted using the Poisson–Boltzmann equation according to the constant regulation approximation.20 The stiffness of the spider silk particles was calculated according to the following equation:
| 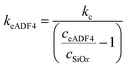 | (2) |
where
ceADF4 and
cSiOx are force responses (
i.e. force
vs. piezo displacement) of the cantilever in contact with the eADF4(C16) and silica particles, respectively.
30 The cantilever force constant is
kc.
Acknowledgements
The authors thank C. Kunert for help with the scanning electron microscopy. The authors further thank A. Fery and M. Neubauer for valuable discussions and M. Hofer as well as J. Engert for generously providing their original data for the electrophoretic mobility at high ionic strength (cf. ref. 6). This work was supported in parts by the Deutsche Forschungsgemeinschaft (SFB 840) as well as Bundesministerium für Bildung und Forschung (BMBF, grant number 13N11340) and by the Deutsche Forschungsgemeinschaft (DFG, grant number SCHE 603/9-1).Notes and references
-
(a) J. M. Gosline, P. A. Guerette, C. S. Ortlepp and K. N. Savage, J. Exp. Biol., 1999, 202, 3295–3303 CAS;
(b) L. Römer and T. Scheibel, Prion, 2008, 2, 154–161 CrossRef;
(c) M. Humenik, A. M. Smith and T. Scheibel, Polymers, 2011, 3, 640–661 CrossRef CAS.
- D. Huemmerich, C. W. Helsen, S. Quedzuweit, J. Oschmann, R. Rudolph and T. Scheibel, Biochemistry, 2004, 43, 13604–13612 CrossRef CAS.
- K. Spiess, A. Lammel and T. Scheibel, Macromol. Biosci., 2010, 10, 998–1007 CrossRef CAS.
- A. Lammel, M. Schwab, M. Hofer, G. Winter and T. Scheibel, Biomaterials, 2011, 32, 2233–2240 CrossRef CAS.
- C. Blüm and T. Scheibel, BioNanoScience, 2012, 2, 67–74 CrossRef.
- M. Hofer, G. Winter and J. Myschik, Biomaterials, 2012, 33, 1554–1562 CrossRef CAS.
- P. H. Hirel, M. J. Schmitter, P. Dessen, G. Fayat and S. Blanquet, Proc. Natl. Acad. Sci. U. S. A., 1989, 86, 8247–8251 CrossRef CAS.
- D. L. Nelson and M. M. Cox, Lehninger: Principles of Biochemistry, Palgrave Macmillan, 2012 Search PubMed.
- M. P. Neubauer, C. Blüm, E. Agostini, J. Engert, T. Scheibel and A. Fery, Biomater. Sci., 2013 10.1039/c3bm60108k.
- R. W. O'Brien and L. R. White, J. Chem. Soc., Faraday Trans. 2, 1978, 74, 1607–1626 RSC.
- A. V. Delgado, F. Gonzalez-Caballero, R. J. Hunter, L. K. Koopal and J. Lyklema, J. Colloid Interface Sci., 2007, 309, 194–224 CrossRef CAS.
- M. Antonietti and L. Vorwerg, Colloid Polym. Sci., 1997, 275, 883–887 CAS.
- M. Borkovec, S. H. Behrens and M. Semmler, Langmuir, 2000, 16, 5209–5212 CrossRef CAS.
- M. Kobayashi, Colloid Polym. Sci., 2008, 286, 935–940 CAS.
-
(a) H. Ohshima, J. Colloid Interface Sci., 1994, 163, 474–483 CrossRef CAS;
(b) J. F. L. Duval and H. P. van Leeuwen, Langmuir, 2004, 20, 10324–10336 CrossRef CAS.
- E. Rotureau, F. Thomas and J. F. L. Duval, Langmuir, 2007, 23, 8460–8473 CrossRef CAS.
- I. Popa, P. Sinha, M. Finessi, P. Maroni, G. Papastavrou and M. Borkovec, Phys. Rev. Lett., 2010, 104, 228301 CrossRef.
- S. Rentsch, R. Pericet-Camara, G. Papastavrou and M. Borkovec, Phys. Chem. Chem. Phys., 2006, 8, 2531–2538 RSC.
- V. Kuznetsov and G. Papastavrou, Langmuir, 2012, 28, 16567–16579 CrossRef CAS.
- R. Pericet-Camara, G. Papastavrou, S. H. Behrens and M. Borkovec, J. Phys. Chem. B, 2004, 108, 19467–19475 CrossRef CAS.
- P. G. Hartley, I. Larson and P. J. Scales, Langmuir, 1997, 13, 2207–2214 CrossRef CAS.
- M. Kobayashi, M. Skarba, P. Galletto, D. Cakara and M. Borkovec, J. Colloid Interface Sci., 2005, 292, 139–147 CrossRef CAS.
- S. Bhattacharjee, C. H. Ko and M. Elimelech, Langmuir, 1998, 14, 3365–3375 CrossRef CAS.
- L. Suresh and J. Y. Walz, J. Colloid Interface Sci., 1997, 196, 177–190 CrossRef CAS.
- H. J. Butt, M. Kappl, H. Mueller, R. Raiteri, W. Meyer and J. Ruhe, Langmuir, 1999, 15, 2559–2565 CrossRef CAS.
- W. Kern and D. A. Puotinen, RCA Rev., 1970, 31, 187–206 CAS.
- R. J. Hunter, Zeta-Potential in Colloid Science, Academic Press, 1989 Search PubMed.
- V. Kuznetsov and G. Papastavrou, Rev. Sci. Instrum., 2012, 83, 116103–116103 CrossRef CAS.
- J. L. Hutter and J. Bechhoefer, Rev. Sci. Instrum., 1993, 64, 1868–1873 CrossRef CAS.
- W. A. Ducker, Z. G. Xu and J. N. Israelachvili, Langmuir, 1994, 10, 3279–3289 CrossRef CAS.
Footnote |
† Electronic supplementary information (ESI) available. See DOI: 10.1039/c3bm60109a |
|
This journal is © The Royal Society of Chemistry 2013 |