DOI:
10.1039/C1SC00726B
(Perspective)
Chem. Sci., 2012,
3, 45-52
Unusual magnetic properties of graphene and related materials
Received
27th September 2011
, Accepted 31st October 2011
First published on 9th November 2011
Abstract
High-temperature ferromagnetism in graphene and other graphite-derived materials reported by several workers has attracted considerable interest. Magnetism in graphene and graphene nanoribbons is ascribed to defects and edge states, the latter being an essential feature of these materials. Room-temperature ferromagnetism in graphene is affected by the adsorption of molecules, especially hydrogen. Inorganic graphene analogues formed by layered materials such as BN and MoS2 also show such ferromagnetic behaviour. Magnetoresistance observed in graphene and graphene nanoribbons is of significance because of the potential applications.
Introduction
Occurrence of high-temperature ferromagnetism in graphite-related materials is a topic of considerable interest. Ferromagnetism has been shown to exist in graphene subjected to irradiation.1,2 Glassy carbon produced by laser ablation is also reported to exhibit ferromagnetic-like behavior with magnetic hysteresis.3 Localized unpaired spins arising from topological and bonding defects are suggested to be responsible for ferromagnetism observed in such nanomaterials. Nanometre-sized graphenes with triangular and hexagonal shapes terminated by zig-zag edges have been predicted to be magnetic.4 Yazyev et al.5 show that magnetism in graphene can be induced by the vacancy defect or by hydrogen chemisorption. Ferromagnetic or antiferromagnetic coupling is observed depending on whether the defects correspond to the same or to different hexagonal sublattices of the graphene lattice. Single-atom defects can induce ferromagnetism in graphene-based materials.6 Vacancies, substitutional atoms (e.g., B or N) and adatoms could also be responsible for the magnetism of graphene.7,8 Some workers suggest that the zig-zag edges are responsible for the magnetic properties of graphene.9 For example, zig-zag edges, longer than 3–4 repeated units are predicted to be magnetic irrespective of whether the edges are regular or irregular. An interplay between mechanical and magnetic properties can occur in graphene wherein the tensile strain along the zig-zag direction enhances the ferromagnetic stability.10 The presence of van Hove singularities can also lead to magnetism and such singularities are generated by the rotation between the stacked layers of graphene.11
In this Perspective, we discuss the magnetic properties of nanographite and related materials, with particular emphasis on graphene and graphene nanoribbons. The effect of adsorption of various molecules, in particular hydrogen, is examined. Magnetic properties of graphene-like BN and MoS2 are discussed, in view of the commonality in morphology and other factors related to the magnetic properties. Since magnetoresistance in materials is of importance in spintronics and other applications, we briefly examine magnetoresistance in graphene and graphene nanoribbons.
Magnetism of nanographite and related materials
The magnetic properties of nanographite prepared by the conversion of nanodiamond at different temperatures have been investigated in detail by Enoki et al.12 The susceptibility at 300 K is negative for all the samples and becomes positive for a sample prepared at 1600 °C which shows Curie-like behavior at low temperatures. The enhanced spin–lattice relaxation rates in the case of more graphitized samples heat-treated at 1400 and 1600 °C are considered to arise from localized edge-state electrons. The number of spins calculated from the Curie constants decrease with the increase in the annealing temperature.13 The inhomogeneous distribution of ferromagnetic structures of nanographene sheets has been observed below 20 K.14 The magnetism of activated carbon fibres (ACF) heated to different temperatures comprising a disordered network of nanographites has been reported.15 The temperature dependence of the susceptibility of ACFs heated from 800 to 1500 °C for 1 h at 1 T is shown in Fig. 1(a). The sample heated at temperatures less than 1100 °C obeys the Curie–Weiss law whereas samples heated to 1200 °C or higher deviate from the Curie–Weiss law below 20 K. Fig. 1(b) shows the field-cooling effect on the susceptibility of the samples heated at 800 and 1100 °C. The samples heated at 1100 °C with a cusp show a difference between the zero-field and the field-cooled data below 15 K. This behavior is explained in terms of disordered magnetism caused by random strengths of inter-nano-graphite antiferromagnetic interactions mediated by p-conduction carriers.
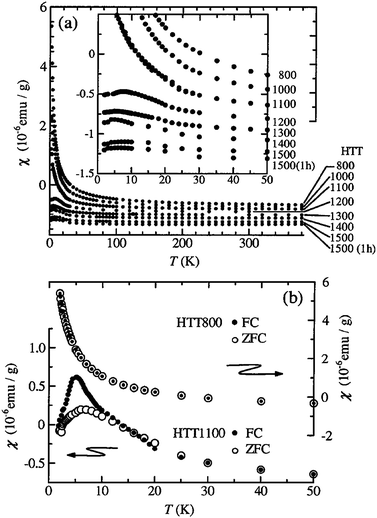 |
| Fig. 1 (a) Temperature dependence of the susceptibility at 1 T for the activated carbon fibres vacuum heat treated up to 1500 °C. The inset shows detailed behavior at low temperatures. (b) Field cooling effects on χ heated at 800 and 1100 °C at 1 T. The measurements were performed in heating runs after the cooling processes down to 2 K with H = 0 (○) and 1 T (•). (Reproduced with permission from ref. 15. Copyright 2000 American Physical Society.) | |
Microporous carbon exhibits high-temperature ferromagnetism originating from topological disorder associated with curved graphene sheets. In Fig. 2, we show zero-field cooled (ZFC) and field-cooled (FC) data on a microporus carbon sample at different fields.16 The difference between values of ZFC and FC magnetization indicates that the microporus carbon possesses a disordered magnetism similar to diluted magnetic semiconductors17,18 and doped rare earth manganites,19 suggesting a percolative-type transition at low temperatures.
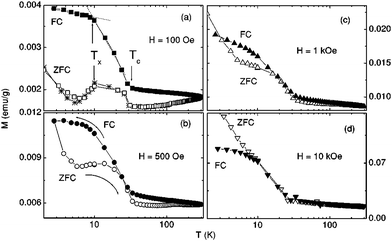 |
| Fig. 2 (a–d) M(T,H) of a microporous carbon sample under ZFC (open symbols) and FC (solid symbols) conditions at various applied magnetic fields.(Reproduced with permission from ref. 16. Copyright 2003 American Physical Society.) | |
Room-temperature ferromagnetism has been reported in highly oriented pyrolytic graphite materials. Macroscopic quantities of magnetic graphite (MG) are produced by controlled etching of graphite employing a redox reaction in a closed system.20MG exhibits a strong ferromagnetic response at room temperature due to topographic defects as shown by magnetic force microscopy. Susceptibility measurements show two transitions, temperatures at 115 K and 315 K, associated with the weak coupling between ferromagnetic regions related to defects and to the ferromagnetism inside the defect regions, respectively.21 Nano fluid magnetic graphite prepared by sonication of magnetic graphite in the presence of a cationic surfactant exhibits room-temperature ferromagnetism.22 Ferromagnetic behavior in highly oriented pyrolytic graphite (HOPG) and Kish graphite samples has been attributed to topological defects and strong Coulomb interaction between electrons.23
Magnetism has been induced in carbon-related materials by irradiation with different ions. Thus, ferro- or ferri-magnetism is triggered in HOPG by proton irradiation of 2.25 MeV. SQUID and MFM measurements show that the magnetic ordering is stable at room temperature.1 Irradiation of HOPG by H, C and N ions in the MeV range triggers Curie-type paramagnetism, ferromagnetism or an anomalous paramagnetic state.2 Room-temperature ferromagnetism in HOPG produced by low-energy carbon-ion implantation can easily be tuned by controlling the implantation dose.24 On bombarding graphite with protons at low temperatures and low fluences, a significant increase in the magnitude of the magnetic order is observed.25 Spectromicroscopy study at room temperature of proton-irradiated metal-free carbon using X-ray magnetic circular dichroism reveals that magnetic order arises from the carbon π electron system.26 Nanosized diamond particles implanted with nitrogen and carbon show ferromagnetic hysteresis at room temperature.27 Ferromagnetic order has been evidenced at defect structures in HOPG using magnetic force microscopy and bulk magnetization measurements at room temperature.28 Ferromagnetic spots created by protons irradiation on graphite surface has been examined by magnetic force microscopy.29Carbon nano-particles produced by a pulsed arc submerged in ethanol show room-temperature magnetic hysteresis.30
Wang et al.31 reported room temperature ferromagnetism in a graphene sample prepared by the partial reduction of graphene oxide with hydrazine followed by annealing the samples at different temperatures in an argon atmosphere. Fig. 3 shows magnetic hysteresis loops measured at 300 K in the field range of −10 kOe < H < +10 kOe for two samples (a) and (b) annealed at 400 °C and 600 °C. Saturation magnetization (Ms) values are 0.004 and 0.020 emu g−1 in (a) and (b) after subtracting the diamagnetic background. These authors suggested long-range coupling of spin units (defects) to be responsible for ferromagnetism.31
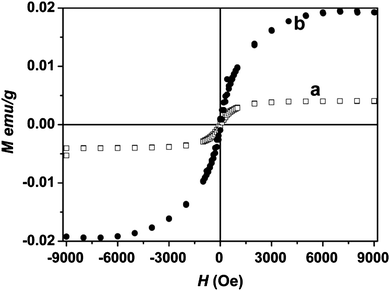 |
| Fig. 3 Magnetization hysteresis loops at 300 K in the range of −10 kOe < H < +10 kOe. (a) Sample Graphene-400 (b) Sample Graphene-600. (Reproduced with permission from ref. 31. Copyright 2009 American Chemical Society.) | |
Magnetic properties of graphene samples prepared by exfoliation of graphite oxide (EG), conversion of nanodiamond (DG) and arc evaporation of graphite in hydrogen (HG) have been studied. The number of graphene layers in EG, DG and HG were estimated to be 6–7, 4–5 and 2–3 respectively.32–34 All these samples show divergence between the FC and ZFC data, starting around 300 K. In Fig. 4(a), we show the temperature-dependence of the magnetization of EG and HG samples measured at 500 Oe. The divergence nearly disappears on application of 1 T as can be seen from the insets in Fig. 4(a). The divergence between the FC and ZFC data in the graphene samples is comparable to that in magnetically frustrated systems such as spin-glasses and superparamagnetic materials. The Curie–Weiss temperatures obtained from the high-temperature inverse susceptibility data were negative in all these samples, indicating the presence of antiferromagnetic interactions. Interestingly, we observe well-defined maxima in the magnetization at low temperatures, the maxima becoming prominent in the data recorded at 1 T (see insets in Fig. 4(a)). Such magnetic anomalies are found when antiferromagnetic correlations compete with ferromagnetic (FM) order. Application of high fields can align the FM clusters and decrease the divergence between FC and ZFC data as is indeed observed. It is possible that the data correspond to percolation type of situation, wherein different types of magnetic states coexist. The FM clusters in such a case would not be associated with a well-defined global ferromagnetic transition temperature. This behavior is similar to that of microporous carbon and some phase-separated members of the rare earth manganite family, Ln1−xAxMnO3 (Ln = rare-earth, A = alkaline earth).35,36 Theoretical calculations predict the presence of antiferromagnetic states in the sheets and ferromagnetic states at the edges of graphene.37
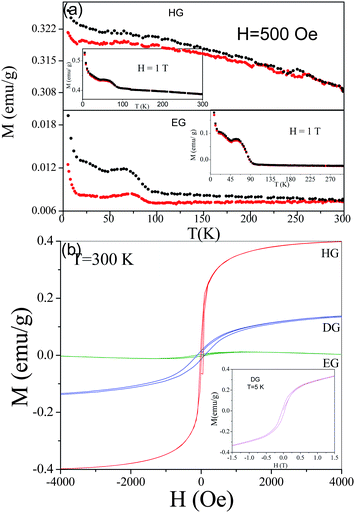 |
| Fig. 4 (a) Temperature variation of the magnetization of few-layer graphenes EG and HG at 500 Oe showing the ZFC and FC data. The insets show the magnetization data at 1 T. (b) Magnetic hysteresis in EG, DG and HG at 300 K. Inset shows magnetic hysteresis in DG at 5 K. (Reproduced with permission from ref. 34. Copyright 2019 American Chemical Society.) | |
The above mentioned graphene samples show magnetic hysteresis at room temperature (Fig. 4(b)) and the Ms increases with increase in temperature. Of the three samples, HG shows the best hysteretic features with saturation. While DG shows saturation, with Ms low compared to HG. We see that θp, Mr and Ms are highest in case of HG, which also shows a higher value of magnetization than the other samples at all temperatures. We have plotted the values of the various magnetic properties of the samples (Ms at 300 K) in Fig. 5(a) to demonstrate how the properties vary as HG > DG > EG. In Fig. 5(b) we show the variation of the average area and the number of layers in these three samples. It is noteworthy that both the area and the number of layers vary in the order of EG > DG > HG. It is likely that edge effects would be greater in samples with a smaller number of layers as well as small areas. In the case of HG, hydrogenation occurred to some extent, thereby favouring ferromagnetism. Magnetic properties of DG samples prepared at different temperatures show a systematic decrease in magnetization with increase in the temperature of preparation. Enough care was taken during the preparation of the various graphene samples described above, in order to ensure that there was no magnetic transition metal impurities, graphene samples were washed with complexing agents. Thus, in the case of EG-H, GO was washed with a solution of 8-hydroxy-quinoline-5-sulfonic acid prior to the reduction. The graphene so obtained also showed magnetic hysteresis at room temperature.
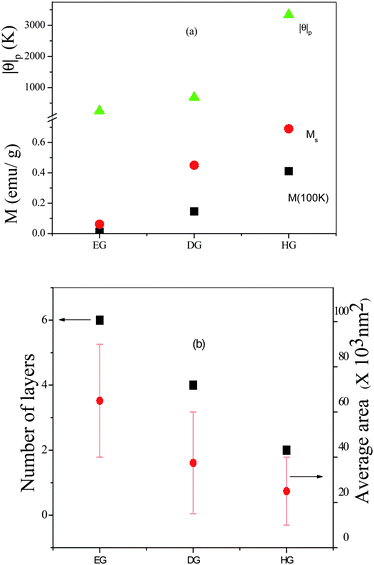 |
| Fig. 5 (a) Comparison of the magnetic properties of the few-layer graphenes, EG, DG and HG. (b) Variation of the number of layers and sample area in the graphene samples. (Reproduced with permission from ref. 34. Copyright 2009 American Chemical Society.) | |
Since the presence of antiferromagnetic interactions as well as magnetic hysteresis are found in the graphene samples, a behavior somewhat like that of frustrated magnetic systems, ac susceptibility measurements have been carried out on HG and DG in the frequency range from 97 to 9997 Hz, but no frequency-dependent features in the ac susceptibility data were found in the 3–300 K range, suggesting that the observed ferromagnetism is not due to spin-glass behavior.
EPR measurements in the 2.5–300 K range on EG, DG and HG show a signal with a line-width of 0.7–2.9 mT and a g-value in the 2.006–2.013 range. The small value of the line-width and the small deviation in the g-value from the free-electron value suggest that the spins do not originate from transition-metal impurities but from the spin species in the graphene sheets. Temperature variation of the EPR intensity is consistent with the magnetization data showing a marked increase at low temperatures. Spin-density measurements on EG, DG and HG samples gave values of 2.86 × 1012, 1.48 × 1013 and 2.46 × 1014 respectively.
Sepioni et al.38 have recently reported the magnetic properties of graphene nanocrystals prepared from HOPG by exfoliation in different organic solvents. The suspensions were centrifuged and passed through alumina to obtain crystallites forming micron-thick laminates. These samples did not show ferromagnetism at any temperature, exhibiting weak paramagnetism at low temperatures, attributed to defects. It may be noted that the synthetic procedure employed by Sepioni et al. may have yielded graphene samples with a smaller concentration of defects as evidenced by the Raman spectrum.39 On the other hand, the few-layer graphene samples discussed earlier contained more defects as well as free spins at the edges and broken bonds. Rout et al.40 found room-temperature ferromagnetism in graphitic petal arrays grown on Si substrates by microwave plasma chemical vapour deposition without any catalyst. They have shown that upon O2annealing, saturation magnetization and corecivity decrease.40
Aryl-radical functionalization of epitaxial graphene leads to disordered magnetism in the graphene sheet, due to the presence of a mixture of ferromagnetic, superparamagnetic and antiferromagnetic regions.41 The functionalization enhances the magnetic properties of the epitaxial graphene. These workers also find that pristine single-layer graphene is inhomogeneous with respect to the number of layers and other features and suggest that the magnetism is confined to the surface of graphene with a distribution of magnetic regions over the surface.42
Effect of adsorbed molecules
Adsorption of different guest molecules on graphene gives rise to a reversible low-spin/high-spin magnetic switching phenomenon which depends on the nature of the guest species. Adsorption of H2O,43 interaction with acids44 and intercalation with potassium clusters reduce the magnetization of nanographite.45 The reduction in magnetization has been interpreted as due to the interaction with lone pair orbitals as well as charge-transfer with graphene sheets. The edge sites participating in host–guest interactions can give rise to magnetic phenomenon. Guest molecules accommodated through physisorption mechanically compress the flexible nanographite domains, leading to a significant reduction in the inter-nanographene sheet distance. Such a reduction in the intersheet distance could align the magnetic moments in anti-parallel and reduce the net magnetic moment.46
Adsorption of benzene solutions of tetrathiafulvalane (TTF) and tetracyanoethylene (TCNE) is found to profoundly affect the magnetic properties of few-layer graphene. In Fig. 6(a), we show typical results on the effect of adsorbing 0.05 M solution of TTF on the magnetic properties of HG. The value of the magnetization drastically decreases on adsorption of TTF and TCNE, although the basic trend in the temperature-variation of magnetization remains the same. Thus, the graphene sample continues to show room-temperature hysteresis. On increasing the concentration of TTF or TCNE, the magnetization value decreases progressively. Interestingly, TTF has a greater effect than TCNE, even though the magnitude of adsorption of TCNE on HG is greater. The value of Ms at 300 K decreases on adsorption of TTF and TCNE, the decrease being larger in the case of former. Clearly, a charge-transfer interaction between graphene and TTF (TCNE)47 is responsible for affecting the magnetic properties. The large reversible concentration-dependent effects of adsorbed TTF and TCNE on the magnetic properties of graphene lend additional evidence to the fact that the magnetic properties of the graphene samples are intrinsic to them.
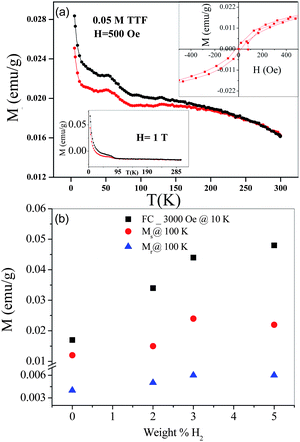 |
| Fig. 6 Temperature-variation of the magnetization of the few-layer graphene HG (500 Oe) after adsorption of 0.05 M solutions of TTF. The magnetization data given in the figure are corrected for the weight of adsorbed TTF. Magnetic hysteresis data at 300 K are shown as insets. Magnetization data at 1 T are shown as an inset. Magnetization data of HG with adsorbed TCNE are similar to those with TTF, except that the decrease in magnetization relative to pure HG is much smaller. (Reproduced with permission from ref. 34. Copyright 2009 American Chemical Society.) (b) Comparison of the magnetic properties of the hydrogenated few-layer graphene, HG : HGH_1, HGH_2 and HGH_3. | |
Effect of interaction of hydrogen
Graphene-like systems on hydrogenation become semiconducting and the edge structures as well as the electronic properties of these materials get modified by hydrogen adsorption. Hydrogenation of graphene can induce magnetism since the formation of tetrahedral carbons can reduce the connectivity of the π-sheets and the π–π energy gap of the localized double bonds and hence the ring current diamagnetism. Such changes in structure can therefore cause an increase in magnetic susceptibility.48
Partial hydrogenation of graphene leads to the formation of unpaired electrons and the remanent delocalized bonding network appears to be responsible for the observed ferromagnetism on epitaxial graphene grown on 4H SiC-(0001).49 Furthermore at the interface between graphene and graphane, magnetism arising from the edges can be tuned.50 Semi-hydrogenated graphene is predicted to be a ferromagnetic semiconductor with a small indirect gap and a Curie temperature between 278 K to 417 K.51 Magnetic properties of graphene with adsorbed hydrogen clusters have been examined.52Hydrogen saturation appears to stabilize the vacancy structure and induce magnetic coupling between the defects.53
Hydrogenated graphene samples with varying hydrogen contents have been prepared by employing birch reduction.54 The samples containing 2 wt%, 3 wt% and 5 wt%, designated HGH_1, HGH_2 and HGH_3 respectively, have been examined for the magnetic properties. We have carried out temperature dependence of magnetization of the few-layer HG along with the hydrogenated samples at 3000 Oe. We observe a gradual increase in the magnetic moment with increase in the hydrogen content. We also measured dependence of magnetization of HG and HGH_2 at 500 Oe. It is also evident that there is an anomaly from 50 K to 80 K in case of ZFC of HGH_2 when compared to HG. This anomaly could be due to percolation type of situation arising from different types of magnetic states. It appears that there is a change in the magnetic structure in HGH_2 compared to HG. In Fig. 6(b) we show the remanent magnetization (Mr), saturation magnetization (Ms) and magnetization at 3000 Oe (FC@10 K) of HG with different weight percentages of hydrogen. The values of these properties increase with hydrogen content. On dehydrogenation at 500 °C for 4 h, the samples exhibit a decrease in the magnetic moment (Table 1). This observation confirms that the increase in the magnetic properties arises from hydrogenation. On dehydrogenation, the hydrogenated samples revert to starting graphene samples.
Table 1 Magnetic properties of the hydrogenated graphene samples
Sample name |
Wt (%) of hydrogen |
M (emu g−1) (FC @ 10 K) |
Saturation magnetization (Ms) |
3000 Oe |
1 T |
100 K |
300 K |
HG
|
|
0.017 |
0.025 |
0.012 |
0.01 |
HGH_1
|
2 |
0.034 |
0.07 |
0.015 |
0.012 |
HGH_2
|
3 |
0.044 |
0.092 |
0.024 |
0.02 |
HGH_3
|
5 |
0.048 |
0.099 |
0.022 |
0.016 |
HGH_2_DH
|
— |
0.038 |
0.048 |
0.019 |
0.015 |
GNRs
The important role of zig-zag edges on the magnetic properties of graphene is underscored by several theoretical studies on graphene nanoribbons (GNR) which predict magnetism in these materials. The electronic states of graphite ribbons with arm-chair and zig-zag edges have been calculated and the latter show a sharp peak of density of states at the Fermi level.55 Pisani et al.56 have shown that the electronic structure of graphene ribbons with zig-zag edges is unstable with respect to magnetic polarization of the edge states and it stabilized by charge polarization or structural distortions. Magnetic interaction of the edge states is in long range, mediated by the delocalized π-orbitals. Mean-field theory predicts that the ground state of zig-zag nanoribbons have unusually stiff parallel spin alignment along each edge and antiferromagnetic inter-edge super-exchange interactions.57 Different magnetic properties can arise in GNRs because of the sensitivity of their band structure to the edge alignment.58 The width of the nanoribbon as well as impurities and defects have effects on the magnetic properties of nanoribbons.59 Magnetism of GNRs is also affected by hydrogenation,60hydroxylation61 and the presence of impurities.62 A few-layer GNR synthesized by chemical vapor deposition method has been studied using near-edge X-ray absorption fine structure and electron-spin resonance. The studies confirm the existence of magnetic edge states that originate from open nanographene edges.63 Half-metallicity in nanometre-scale graphene ribbons is predicted by first-principles calculations. By applying the electric fields across the zig-zag- edges, their magnetic properties can be controlled.64 Tao et al.65 have studied sub-nanometre-resolved scanning tunneling microscopy and spectroscopy on GNRs synthesized from the unzipping of carbon nanotubes. The measurements reveal the presence of edge states, corroborating the theoretical calculations of GNRs of similar width and chirality.
Magnetoresistance
Hwang and Das Sarma66 proposed a theory for graphene magnetotransport in the presence of carrier spin polarization and predicted a negative magnetoresistance (MR) for graphene. Epitaxial graphene and the isostructural hexagonal boron nitride (h-BN) are proposed as efficient ultrathin covalent spacers for traditional multilayer magnetoresistive junctions.67 Negative tunnel magnetoresistance has been observed in ferromagnetic graphene (FG) and normal graphene (NG) employing FG/NG/FG junctions.68Graphite and graphene on (111) fcc or (0001) hcp Ni or Co has led to the prediction of spin filtering.69 The magnetoresistance of Co/multilayer graphene/Co spin valve structure has been studied by Nishioka and Goldman70 who find a positive MR of up to 0.39% at 2 K. Spin-dependent properties of single-layer graphene have been studied by spin-valve measurements at room temperature and the nonlocal magnetoresistance was found to be proportional to conductivity. This study also shows electron-hole symmetry.71 A study of tunnelling spin junction from Co to single-layer graphene has yielded a large value of MR.72 Suspended bilayer graphene devices are shown to exhibit quantum Hall states that are fully quantized at low magnetic fields and show extremely high magnetoresistance that scales as the magnetic field divided by temperature.73 Linear MR is found in multi-layer epitaxial graphene grown on SiC exhibits74 MR between 80–250% at 12 T. In dilute fluorinated graphene a large MR has been observed.75 Tunable magnetometers have been created by combining the Hall effect and the MR of graphene.76 Preliminary experiments in this laboratory have shown considerable MR in the few-layer graphene HG which also has high carrier mobility.77
Graphene quantum sheets with zig-zag edge states obtained from GNRs by ultra sonication show room temperature ferromagnetism with strong exchange bias field and around 35% of magnetoresistance at moderate fields and at room temperature.78 These authors proposed these properties can be used for the spintronic devices. The magnetic data and hysteresis curves found by these authors are very similar to those reported by Matte et al.34 Based on first-principle calculations, magnetized zig-zag nanoribbons are shown to induce thermally spin transport and substantial MR.79 Zig-zag GNR bridging two metallic graphene electrodes appear to show spin-polarized electron transport and large magnetoresistance.80 Theoretical studies show that GNRs can exhibit large MR. A GNR field-effect transistor has been found to exhibit negative magnetoresistance.81 The MR could be tuned by source drain bias. Fig. 7(a) & (b) show the current ratio and negative magnetoresistance versus source–drain bias at different temperatures and Vg = 3 V. The maximum current ratio decreases from more than 10,000 at 1.6 K (magnetoresistance = −95%) as the conductance suppression due to the conduction band gap and/or Coulomb blockade effect is weakened with the increase in temperature. The conduction band gap apparently disappears at room temperature as the device shows the linear transport behavior as shown in Fig. 7(c).81
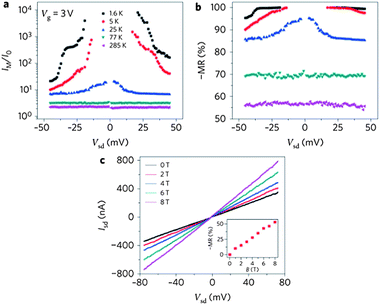 |
| Fig. 7 Temperature-dependent magneto-transport properties. Current ratio I(8 T)/I(0 T) (a) and negative magnetoresistance (MR) (b) as a function of source–drain bias at 1.6, 5, 25, 77 and 285 K. The device was gated at 3 V. (c) Room-temperature (285 K) I–V characteristic (Vg = 3 V) with different applied magnetic fields. The inset shows the negative magnetoresistance increasing nearly linearly with the applied magnetic field. (Reproduced with permission from ref. 81. Copyright 2010 Nature publishing group.) | |
Graphene analogues of other inorganic materials such as BN and dichalcogenides of molybdenum and tungsten have been explored recently.82–85 There are literature reports of room-temperature magnetism in graphene analogues. Nanosheet-like MoS2 films exhibit weak magnetism and 2.5% magnetoresistance and this behavior is related to the presence of edge spins on the prismatic edges of the nanosheets where the terminating atoms are unsaturated.86 Spin-polarized density-functional theory (DFT) calculations on triangular shaped clusters show that magnetic states are present in clusters terminated by 0% S-covered Mo edge and 100% S-covered S edge.86 Dev et al.87 suggest that defects, such as cation vacancies, could induce intrinsic long-range magnetic order in BN. Intrinsic ferromagnetism at room temperature has been observed in graphene-like BN. The observed magnetism is not from the magnetic impurities but arises from free spins available for the ferromagnetic ordering due to conversion of sp3 to sp3-sp2 hybridization or from the unpaired spin electron from the defects.88 Presence of zig-zag edges is clearly a cause of magnetism in these materials just as in graphene. Magnetic properties of graphene analogues of MoS2, WS2, and BN with different numbers of layers have been studied in this laboratory. They also exhibit room-temperature ferromagnetism. (Fig. 8) There is an increase in the magnetic moment with the decreasing number of layers as illustrated in Fig. 8(d) in the case of BN. Similar results have been found with the graphene-like MoS2. We show the presence of edges in graphene-like MoS2 in Fig. 9.
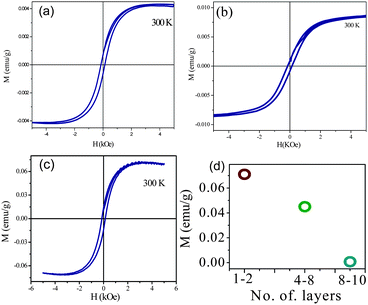 |
| Fig. 8 Magnetic hysteresis of graphene-like (a) MoS2 (b) WS2 and (c) BN at 300 K. (d) shows layer dependent magnetism of BN. | |
Conclusions
Many of the studies on few-layer graphenes show the presence of room-temperature ferromagnetism. While defects can be of importance, the role of edges in these materials seems to be vital. Since edges of zig-zag type are generally present in graphenes, one would surmise that the magnetism is an intrinsic feature of most graphene samples. It is therefore understandable that the adsorption of electron donor and acceptor molecules affects the magnetic properties of graphene. The interaction of hydrogen significantly enhances the magnetization of few-layer graphenes. Observation of room-temperature ferromagnetism in graphene analogues of other inorganic materials also suggests the importance of edge effects and defects. It is noteworthy that defect-related magnetism is observed in nanoparticles of inorganic nanomaterials such as ZnO and Al2O3 which are otherwise nonmagnetic.89 Observation of substantial magnetoresistance in graphene-like materials suggests the possible use in device applications.
Acknowledgements
The authors thank Dr Ranjan Datta for TEM images.
Notes and references
- P. Esquinazi, D. Spemann, R. Hohne, A. Setzer, K. H. Han and T. Butz, Phys. Rev. Lett., 2003, 91, 227201 CrossRef CAS.
-
M. A. Ramos, J. Barzola-Quiquia, P. Esquinazi, A. Muñoz-artin, A. Climent-Font and M. G. Hernandez, Phys. Rev. B, 81, p. 214404 Search PubMed.
- A. V. Rode, E. G. Gamaly, A. G. Christy, J. G. Fitz Gerald, S. T. Hyde, R. G. Elliman, B. Luther-Davies, A. I. Veinger, J. Androulakis and J. Giapintzakis, Phys. Rev. B: Condens. Matter Mater. Phys., 2004, 70, 054407 CrossRef.
- J. Fernandez-Rossier and J. J. Palacios, Phys. Rev. Lett., 2007, 99, 177204 CrossRef CAS.
- O. V. Yazyev and L. Helm, Phys. Rev. B: Condens. Matter Mater. Phys., 2007, 75, 125408 CrossRef.
- O. V. Yazyev, Phys. Rev. Lett., 2008, 101, 037203 CrossRef.
- R. Singh and P. Kroll, J. Phys.: Condens. Matter, 2009, 21, 196002 CrossRef.
- M. M. Ugeda, I. Brihuega, F. Guinea and J. M. Gomez-Rodriguez, Phys. Rev. Lett., 2010, 104, 096804 CrossRef CAS.
- S. Bhowmick and V. B. Shenoy, J. Chem. Phys., 2008, 128, 244717 CrossRef.
- L. Z. Kou, C. Tang, W. L. Guo and C. F. Chen, ACS Nano, 2011, 5, 1012 CrossRef CAS.
- G. H. Li, A. Luican, J. dos Santos, A. H. C. Neto, A. Reina, J. Kong and E. Y. Andrei, Nat. Phys., 2009, 6, 109 CrossRef.
- O. E. Andersson, B. L. V. Prasad, H. Sato, T. Enoki, Y. Hishiyama, Y. Kaburagi, M. Yoshikawa and S. Bandow, Phys. Rev. B: Condens. Matter, 1998, 58, 16387 CrossRef CAS.
- B. L. V. Prasad, H. Sato, T. Enoki, Y. Hishiyama, Y. Kaburagi, A. M. Rao, P. C. Eklund, K. Oshida and M. Endo, Phys. Rev. B: Condens. Matter, 2000, 62, 11209 CrossRef CAS.
- V. L. J. Joly, K. Takahara, K. Takai, K. Sugihara, T. Enoki, M. Koshino and H. Tanaka, Phys. Rev. B: Condens. Matter Mater. Phys., 2010, 81, 115408 CrossRef.
- Y. Shibayama, H. Sato, T. Enoki and M. Endo, Phys. Rev. Lett., 2000, 84, 1744 CrossRef CAS.
- Y. Kopelevich, R. R. da Silva, J. H. S. Torres, A. Penicaud and T. Kyotani, Phys. Rev. B: Condens. Matter Mater. Phys, 2003, 68, 092408 CrossRef.
- N. Theodoropoulou, A. F. Hebard, M. E. Overberg, C. R. Abernathy, S. J. Pearton, S. N. G. Chu and R. G. Wilson, Phys. Rev. Lett., 2002, 89, 107203 CrossRef CAS.
- S. Chaudhary, M. K. Chattopadhyay, K. J. Singh, S. B. Roy, P. Chaddah and E. V. Sampathkumaran, Phys. Rev. B: Condens. Matter, 2002, 66, 014424 CrossRef.
- J. Dho, W. S. Kim and N. H. Hur, Phys. Rev. Lett., 2002, 89, 027202 CrossRef.
- H. Pardo, R. Faccio, F. M. Araújo-Moreira, O. F. de Lima and A. W. Mombrú, Carbon, 2006, 44, 565 CrossRef CAS.
- A. W. Mombru, H. Pardo, R. Faccio, O. F. de Lima, E. R. Leite, G. Zanelatto, A. J. C. Lanfredi, C. A. Cardoso and F. M. Araujo-Moreira, Phys. Rev. B: Condens. Matter Mater. Phys., 2005, 71, 100404 CrossRef.
- N. S. Souza, S. Sergeenkov, C. Speglich, V. A. G. Rivera, C. A. Cardoso, H. Pardo, A. W. Mombru, A. D. Rodrigues, O. F. de Lima and F. M. Araujo-Moreira, Appl. Phys. Lett., 2009, 95, 233120 CrossRef.
- P. Esquinazi, A. Setzer, R. Hohne, C. Semmelhack, Y. Kopelevich, D. Spemann, T. Butz, B. Kohlstrunk and M. Sche, Phys. Rev. B: Condens. Matter, 2002, 66, 024429 CrossRef.
- H. Xia, W. Li, Y. Song, X. Yang, X. Liu, M. Zhao, Y. Xia, C. Song, T.-W. Wang, D. Zhu, J. Gong and Z. Zhu, Adv. Mater., 2008, 20, 4679 CrossRef CAS.
- J. Barzola-Quiquia, P. Esquinazi, M. Rothermel, D. Spemann, T. Butz and N. Gracia, Phys. Rev. B: Condens. Matter Mater. Phys., 2007, 76, 161403 CrossRef.
- H. Ohldag, T. Tyliszczak, R. Hohne, D. Spemann, P. Esquinazi, M. Ungureanu and T. Butz, Phys. Rev. Lett., 2007, 98, 187204 CrossRef CAS.
- S. Talapatra, P. G. Ganesan, T. Kim, R. Vajtai, M. Huang, M. Shima, G. Ramanath, D. Srivastava, S. C. Deevi and P. M. Ajayan, Phys. Rev. Lett., 2005, 95, 097201 CrossRef CAS.
- J. Cervenka, M. I. Katsnelson and C. F. J. Flipse, Nat. Phys., 2009, 5, 840 CrossRef CAS.
- K. H. Han, D. Spemann, P. Esquinazi, R. Höhne, V. Riede and T. Butz, Adv. Mater., 2003, 15, 1719 CrossRef CAS.
- N. Parkansky, B. Alterkop, R. L. Boxman, G. Leitus, O. Berkh, Z. Barkay, Y. Rosenberg and N. Eliaz, Carbon, 2008, 46, 215–219 CrossRef CAS.
- Y. Wang, Y. Huang, Y. Song, X. Y. Zhang, Y. F. Ma, J. J. Liang and Y. S. Chen, Nano Lett., 2009, 9, 220 CrossRef CAS.
- C. N. R. Rao, K. S. Subrahmanyam, H. S. S. Ramakrishna Matte, B. Abdulhakeem, A. Govindaraj, B. Das, P. Kumar, A. Ghosh and D. J. Late, Sci. Technol. Adv. Mater., 2010, 11, 054502 CrossRef.
- C. N. R. Rao, A. K. Sood, K. S. Subrahmanyam and A. Govindaraj, Angew. Chem., Int. Ed., 2009, 48, 7752 CrossRef CAS.
- H. S. S. R. Matte, K. S. Subrahmanyam and C. N. R. Rao, J. Phys. Chem. C, 2009, 113, 9982 CAS.
- V. B. Shenoy, D. D. Sarma and C. N. R. Rao, ChemPhysChem, 2006, 7, 2053 CrossRef CAS.
- V. B. Shenoy and C. N. R. Rao, Philos. Trans. R. Soc. London, Ser. A, 2008, 366, 63 CrossRef CAS.
- S. Dutta, S. Lakshmi and S. K. Pati, Phys. Rev. B: Condens. Matter Mater. Phys., 2008, 77, 073412 CrossRef.
- M. Sepioni, R. R. Nair, S. Rablen, J. Narayanan, F. Tuna, R. Winpenny, A. K. Geim and I. V. Grigorieva, Phys. Rev. Lett., 2010, 105, 207205 CrossRef CAS.
- Y. Hernandez, V. Nicolosi, M. Lotya, F. M. Blighe, Z. Sun, S. De, I. T. McGovern, B. Holland, M. Byrne, Y. K. Gun'Ko, J. J. Boland, P. Niraj, G. Duesberg, S. Krishnamurthy, R. Goodhue, J. Hutchison, V. Scardaci, A. C. Ferrari and J. N. Coleman, Nat. Nanotechnol., 2008, 3, 563 CrossRef CAS.
- C. S. Rout, A. Kumar, N. Kumar, A. Sundaresan and T. S. Fisher, Nanoscale, 2011, 3, 900 RSC.
- J. Hong, S. Niyogi, E. Bekyarova, M. E. Itkis, P. Ramesh, N. Amos, D. Litvinov, C. Berger, W. A. de Heer, S. Khizroev and R. C. Haddon, Small, 2011, 7, 1175 CrossRef CAS.
- S. Niyogi, E. Bekyarova, J. Hong, S. Khizroev, C. Berger, W. de Heer and R. C. Haddon, J. Phys. Chem. Lett., 2011, 2, 2487 CrossRef CAS.
- H. Sato, N. Kawatsu, T. Enoki, M. Endo, R. Kobori, S. Maruyama and K. Kaneko, Solid State Commun., 2003, 125, 641 CrossRef CAS.
- S. Hao, K. Takai, K. Feiyu and T. Enoki, Carbon, 2008, 46, 110 CrossRef CAS.
- K. Takai, S. Eto, M. Inaguma, T. Enoki, H. Ogata, M. Tokita and J. Watanabe, Phys. Rev. Lett., 2007, 98, 017203 CrossRef.
- T. Enoki and K. Takai, Dalton Trans., 2008, 3773 RSC.
- C. N. R. Rao and R. Voggu, Mater. Today, 2010, 13, 34 CrossRef CAS.
-
T. Makarova and F. Palacio, Carbon Based Magnetism (Elsevier, Amsterdam, 2006) Search PubMed.
- L. Xie, X. Wang, J. Lu, Z. Ni, Z. Luo, H. Mao, R. Wang, Y. Wang, H. Huang, D. Qi, R. Liu, T. Yu, Z. Shen, T. Wu, H. Peng, B. Ozyilmaz, K. Loh, A. T. S. Wee, Ariando and W. Chen, Appl. Phys. Lett., 2011, 98, 193113 CrossRef.
- M. J. Schmidt and D. Loss, Phys. Rev. B: Condens. Matter Mater. Phys., 2010, 81, 165439 CrossRef.
- J. Zhou, Q. Wang, Q. Sun, X. S. Chen, Y. Kawazoe and P. Jena, Nano Lett., 2009, 9, 3867 CrossRef CAS.
- A. Ranjbar, M. S. Bahramy, M. Khazaei, H. Mizuseki and Y. Kawazoe, Phys. Rev. B: Condens. Matter Mater. Phys., 2010, 82, 165446 CrossRef.
- W. F. Li, M. W. Zhao, X. A. Zhao, Y. Y. Xia and Y. G. Mu, Phys. Chem. Chem. Phys., 2010, 12, 13699 RSC.
- K. S. Subrahmanyam, P. Kumar, U. Maitra, A. Govindaraj, K. Hembram, U. V. Waghmare and C. N. R. Rao, Proc. Natl. Acad. Sci. U. S. A., 2011, 108, 2674 CrossRef CAS.
- M. Fujitha, K. Wakabayashi, K. Nakada and K. Kusakabe, J. Phys. Soc. Jpn., 1996, 65, 1920 CrossRef.
- L. Pisani, J. A. Chan, B. Montanari and N. M. Harrison, Phys. Rev. B: Condens. Matter Mater. Phys., 2007, 75, 064418 CrossRef.
- O. V. Yazyev and M. I. Katsnelson, Phys. Rev. Lett., 2008, 100, 047209 CrossRef.
- B. Sahu, H. K. Min and S. K. Banerjee, Phys. Rev. B: Condens. Matter Mater. Phys., 2010, 82, 115426 CrossRef.
- D. W. Boukhvalov and M. I. Katsnelson, Nano Lett., 2008, 8, 4373 CrossRef CAS.
- H. Xiang, E. Kan, S.-H. Wei, M.-H. Whangbo and J. Yang, Nano Lett., 2009, 9, 4025 CrossRef CAS.
- D. W. Boukhvalov, Chem. Phys. Lett., 2011, 501, 396 CrossRef CAS.
- R. C. Longo, J. Carrete and L. J. Gallego, J. Chem. Phys., 2011, 134, 024704 CrossRef CAS.
- V. L. J. Joly, M. Kiguchi, S.-J. Hao, K. Takai, T. Enoki, R. Sumii, K. Amemiya, H. Muramatsu, T. Hayashi, Y. A. Kim, M. Endo, J. Campos-Delgado, F. Lapez-Urias, A. Botello-Menndez, H. Terrones, M. Terrones and M. S. Dresselhaus, Phys. Rev. B: Condens. Matter Mater. Phys., 2010, 81, 245428 CrossRef.
- Y.-W. Son, M. L. Cohen and S. G. Louie, Nature, 2006, 444, 347 CrossRef CAS.
- C. Tao, L. Jiao, O. V. Yazyev, Y.-C. Chen, J. Feng, X. Zhang, R. B. Capaz, J. M. Tour, A. Zettl, S. G. Louie, H. Dai and M. F. Crommie, Nat. Phys., 2011, 7, 616 CrossRef CAS.
- E. H. Hwang and S. Das Sarma, Phys. Rev. B: Condens. Matter Mater. Phys., 2009, 80, 075417 CrossRef.
- O. V. Yazyev and A. Pasquarello, Phys. Rev. B: Condens. Matter Mater. Phys., 2009, 80, 035408 CrossRef.
- J. F. Zou, G. J. Jin and Y. Q. Ma, J. Phys.: Condens. Matter, 2009, 21, 126001 CrossRef.
- V. M. Karpan, G. Giovannetti, P. A. Khomyakov, M. Talanana, A. A. Starikov, M. Zwierzycki, J. van den Brink, G. Brocks and P. J. Kelly, Phys. Rev. Lett., 2007, 99, 176602 CrossRef CAS.
- M. Nishioka and A. M. Goldman, Appl. Phys. Lett., 2007, 90, 252505 CrossRef.
- W. Han, W. H. Wang, K. Pi, K. M. McCreary, W. Bao, Y. Li, F. Miao, C. N. Lau and R. K. Kawakami, Phys. Rev. Lett., 2009, 102, 137205 CrossRef.
- W. Han, K. Pi, K. M. McCreary, Y. Li, J. J. I. Wong, A. G. Swartz and R. K. Kawakami, Phys. Rev. Lett., 2010, 105, 167202 CrossRef.
- B. E. Feldman, J. Martin and A. Yacoby, Nat. Phys., 2009, 5, 889 CrossRef CAS.
- A. L. Friedman, J. L. Tedesco, P. M. Campbell, J. C. Culbertson, E. Aifer, F. K. Perkins, R. L. Myers-Ward, J. K. Hite, C. R. Eddy, G. G. Jernigan and D. K. Gaskill, Nano Lett., 2010, 10, 3962 CrossRef CAS.
- X. Hong, S. H. Cheng, C. Herding and J. Zhu, Phys. Rev. B: Condens. Matter Mater. Phys., 2011, 83, 085410 CrossRef.
- S. Pisana, P. M. Braganca, E. E. Marinero and B. A. Gurney, Nano Lett., 2010, 10, 341 CrossRef CAS.
- D. J. Late, A. Ghosh, K. S. Subrahmanyam, L. S. Panchakarla, S. B. Krupanidhi and C. N. R. Rao, Solid State Commun., 2010, 150, 734 CrossRef CAS.
- S. K. Saha, M. Baskey and D. Majumdar, Adv. Mater., 2010, 22, 5531 CrossRef CAS.
- M. G. Zeng, Y. P. Feng and G. C. Liang, Nano Lett., 2011, 11, 1369 CrossRef CAS.
- F. Munoz-Rojas, J. Fernandez-Rossier and J. J. Palacios, Phys. Rev. Lett., 2009, 102, 136810 CrossRef CAS.
- J. W. Bai, R. Cheng, F. X. Xiu, L. Liao, M. S. Wang, A. Shailos, K. L. Wang, Y. Huang and X. F. Duan, Nat. Nanotechnol., 2010, 5, 655 CrossRef CAS.
- C. N. R. Rao and A. Nag, Eur. J. Inorg. Chem., 2010, 2010, 4244 CrossRef.
- H. S. S. Ramakrishna Matte, A. Gomathi, A. K. Manna, D. J. Late, R. Datta, S. K. Pati and C. N. R. Rao, Angew. Chem., Int. Ed., 2010, 49, 4059 Search PubMed.
- H. S. S. R. Matte, B. Plowman, R. Datta and C. N. R. Rao, Dalton Trans., 2011, 40, 10322 RSC.
- A. Nag, K. Raidongia, K. P. S. S. Hembram, R. Datta, U. V. Waghmare and C. N. R. Rao, ACS Nano, 2010, 4, 1539 CrossRef CAS.
- J. Zhang, J. M. Soon, K. P. Loh, J. Yin, J. Ding, M. B. Sullivian and P. Wu, Nano Lett., 2007, 7, 2370 CrossRef CAS.
- P. Dev, Y. Xue and P. Zhang, Phys. Rev. Lett., 2008, 100, 117204 CrossRef.
- B. Song, J. C. Han, J. K. Jian, H. Li, Y. C. Wang, H. Q. Bao, W. Y. Wang, H. B. Zuo, X. H. Zhang, S. H. Meng and X. L. Chen, Phys. Rev. B: Condens. Matter Mater. Phys., 2009, 80, 153203 CrossRef.
- A. Sundaresan and C. N. R. Rao, Nano Today, 2009, 4, 96 CrossRef CAS.
|
This journal is © The Royal Society of Chemistry 2012 |