Problem types in synthetic organic chemistry research: Implications for the development of curricular problems for second-year level organic chemistry instruction
Received
22nd July 2011
, Accepted 3rd January 2012
First published on 17th January 2012
Abstract
Understanding of the nature of science is key to the development of new curricular materials that mirror the practice of science. Three problem types (project level, synthetic planning, and day-to-day) in synthetic organic chemistry emerged during a thematic content analysis of the research experiences of eight practising synthetic organic chemists. Project-level problems include the overarching purpose of synthesizing target molecules. Synthetic planning problems include both the retrosynthetic analysis of target molecules and subsequent development of synthetic pathway proposals. Day-to-day problems include the ‘hurdles’ faced in research laboratories while attempting to realize proposed synthetic pathways. Recommendations are made as to how understanding of the three problem types impact undergraduate-level organic chemistry instruction.
Introduction
Understanding of the nature of science is a necessary component of the collegiate science curriculum (Edelson, 1998). Both practical experiences of chemistry research and pseudo-research experiences (e.g., the work of the Center for Authentic Science Practice in Education in incorporating research into the undergraduate laboratory instruction, see www.purdue.edu/dp/caspie) show merit for improving student understanding of the nature of science, persistence in the study of chemistry, and interest in careers in chemistry (Bowen and Roth, 2000; Lindsay and McIntosh, 2000; Russell and Weaver, 2011; Samarapungaven et al., 2006). Unfortunately, financial resources, laboratory space, and large course enrollments preclude many institutions of higher learning from incorporating research-like laboratory experiences into the curriculum for all students (majors and non-majors, alike).
The development of problem-solving abilities is a frequently cited and fundamental outcome of the chemistry and broader educational curriculum (Heyworth, 1999; American Chemical Society, 2008; Zoller, 1987). The American Chemical Society (ACS) Committee on Professional Training has proposed that “problem solving is one of the most empowering learning experiences for science students” (2002, p. 3). It has been argued that scientific research is fundamentally problem solving (Bodner, 1991; Nersessian, 1995), and that through instruction on problem solving, the practice of science can be taught (Bodner and Domin, 2000; Society Committee on Education, 2003).
Because of these goals, the authors (JRR & MHT) then set out to develop pseudo-research experiences for the lecture component of second-year organic chemistry courses through instructional problem sets, a much less resource dependent part of the chemistry curriculum than the instructional laboratory. Pseudo-research experiences seek to incorporate research type problems and situations into the context of the traditional curriculum.
Using an instructional design methodology (IDM) discussed by Jonassen and Hernandez-Serrano (2002), the authors sought to transform the problem-solving experiences of practising organic chemists into instructional problems for undergraduate students. A preliminary component of the IDM was to establish an understanding of problem-solving experiences in synthetic organic chemistry, i.e. the nature of synthetic organic practice. This understanding was achieved through a review of the science education and organic chemistry literature, a review of graduate-level instructional textbooks and research overviews, and interviews with eight practising synthetic organic chemists (six graduate students and two postdoctoral researchers) in academia. Presentation of the results from interviews with the eight practising synthetic organic chemists is the purview of this article.
The three problem types found in synthetic research, as discussed by the eight practising synthetic organic chemist participants, will first be presented. This understanding of the practice of synthetic organic chemistry will be followed by a discussion of implications for the design and development of sophomore-level organic chemistry instructional problems.
Methodology
Problem solving is an experience of a problem solver in a problem-solving environment seeking, developing, and implementing a solution to a problem. In order to refine problems for the undergraduate classroom, a sense of the problem-solving experience of practising organic chemists had to be established. The results described herein are one component of a larger study on the holistic problem-solving experiences of practising synthetic organic chemists based on an instructional design model. Details of the instructional design model, participants, interview structure, and data analysis will be outlined in this section.
Instructional design model
Jonassen and Hernandez-Serrano (2002) outlined a methodology for designing instructional materials from interviews and think-aloud protocols with practitioners. This instructional design model (IDM) was utilized as a framework for an overarching study that outlined the three types of problems found in synthesis research and presented in this paper. The IDM is based on the idea that instructional problems can be developed from the problem-solving experiences of “skilled practitioners.” For the purposes of this study, these are individuals, not deemed experts, that are able to solve problems intended for novice learners and articulate the knowledge and skills necessary to solve said problems. Data from the “skilled practitioners” problem-solving experiences and reflections on these experiences were used to generate and refine new instructional problems. This model informed the selection of participants, development of interview protocols, and data analysis
Description of participants
Eight practising synthetic organic chemists were sampled from a research-focused academic institution. Participants were given a pseudonym for this paper to protect their anonymity. (Paraphrased statements and quotations from these chemists will be noted by a first name pseudonym.) The participants included six males, and two females; seven from a chemistry department and one from a medicinal chemistry department; and, five US citizens and three International persons. Participants' educational background spanned from 3rd year graduate student to postdoctoral researcher. The participant sample was purposefully constrained to graduate students and postdoctoral researchers in accordance with the instructional design methodology's recommendation to select “skilled practitioners.” While the authors recognize that this excludes synthetic organic chemists working in industry, the participant sample was able to articulate a level of knowledge and skills appropriate for this study. Participants worked in several sub-discipline and application areas of organic chemistry: biochemistry, medicinal chemistry, total synthesis and methodological. Five of the eight participants worked on the design of drugs and medicinal chemistry-focused research. Research group settings ranged from 9 to 25 members. Experience before doctoral studies included internships, research experiences, and work in field biology, inorganic chemistry, materials science, medicinal chemistry, organic synthesis, and pharmaceutical industry (both research and pharmacy practice).
Description of interview protocols
Two semi-structured interviews were intended for each practising synthetic organic chemist participant. The first interview, based on a literature reported protocol (Jonassen et al., 2006), asked questions about the participant's background as a chemist and general experience solving organic synthesis problems (i.e., general definition of a research problem, problem-solving strategies, observed changes in problem-solving ability, and a discussion of recent and ongoing research problems). Interview 1 lasted from 20 to 47 min. The second interview, completed by six of the eight participants, consisted of a think-aloud problem-solving protocol followed by reflection questions on whether the participant had experienced similar obstacles in their research experience. Two participants opted not to participate in the second interview of the study due to scheduling conflicts; data from their first interview informs the results presented herein and were thus included in data analysis. Interview 2 lasted from 35 to 57 min.
Description of think-aloud problems
Five “classroom” problems for practising organic chemists were developed in consultation with a practising organic chemist prior to the think-aloud interviews of the eight participants. The consulting chemist had both discipline expertise and experience teaching sophomore-level organic chemistry; this background made the chemist qualified to provide support and guidance for the project. These “classroom” problems were developed utilizing the IDM in conjunction with literature accounts and the authors' understanding of the practice of organic chemistry. The problems were developed with the intent of sparking further thought and reflection on types of problems and the problem-solving process of synthetic organic chemistry. A description of the five “classroom” problems will now be described so that a reader may discern the impact of the “classroom” problems on the “research” problem types reported in this paper.
Two problems asked participants to confirm the identity of a desired product and to “prepare items necessary to discuss your work for Friday's research group meeting” based on experimental data (including proton and carbon-13 nuclear magnetic resonance spectroscopy data for both starting materials and obtained reaction products). Nuclear magnetic resonance spectroscopy is an experimental technique used to identify the types, number, and chemical environment of observed atoms.
One problem asked participants to consider two provided synthetic pathways to a given target molecule and “recommend an improved synthetic route” with the intent of “reducing the number of synthetic steps and increasing the overall selectivity and yield of the synthetic pathway.”
One problem asked participants to develop experimental procedures for a given series of compounds based on a literature-reported reaction methodology.
One problem asked participants to conduct a retrosynthetic analysis and to propose a synthetic pathway for a given biologically active target molecule. Retrosynthetic analysis is a backward thinking strategy where the researcher begins with the target molecule in mind and then “disconnects” the molecule using viable reactions until commercially available reagents remain.
Six of the participants completed two to three of these “classroom” problems. The choice, number, and order of the problems utilized for each participant was decided by the interviewer (author JRR), in the context of the interview, in response to the interview length and ability of participants to generate solutions to and reflect on the “classroom” problems. The goal of the think-aloud protocol was to generate reflection on the types of problems and the problem-solving processes of synthetic organic chemists; therefore, the process of think aloud problem selection is consistent with the goal of obtaining data-rich reflections by the participant's on their “research” problem-solving experiences. Each “classroom” problem, except one, was sampled three times; the total synthesis problem was sampled four times.
Thematic content analysis
Data analysis began with transcription of the audiotaped interviews. As transcription occurred, memos were written about particular passages of interest including initial thoughts, reflections, and potential problem types (Patton, 2002). Segments of each interview where problems were described were extracted. These problem episodes were then categorized based on similar features and themes. Through a process of constant comparison (Lincoln and Guba, 1985; Patton, 2002), problem categories (i.e., themes) were condensed into three main problem types reported in the next section.
Types of problems in research process
Three types of “research” problems emerged from our analysis of interviews with practising synthetic organic chemists: project-level problems, synthetic planning problems, and day-to-day problems. These problems types span the research process in synthetic organic chemistry from project conception to completion.
Project-level problems
Project-level problems encompass the identification and selection of target molecules for synthesis. Target molecules (e.g., natural products and analogs) are chosen for their complexity and functionalities (“Alberto;” participant pseudonyms will be noted in quotation marks throughout). In general, synthetic targets could be an exact structure (e.g., a natural product with bioactivity), a particular functionality or property (e.g., synthetic polymeric units), or a novel structure (e.g., longifolene at the time of its discovery). (See Scheme 1 for an example of an exact structure target molecule, salvinorin A, a compound with implications for understanding hallucinogens.)
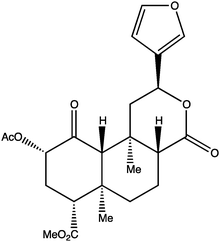 |
| Scheme 1 An example of a project-level target molecule, salvinorin A. | |
Any molecule “that can be drawn [and] pretty much anything that has been isolated from nature can be [synthesized] with enough effort” (“Ignatius;” & cf.Deslongchamps, 1984). The choice of a target molecule is governed by several factors identified by the participants: chemical resources, funding sources, available laboratory equipment, synthetic difficulty, the opportunity to test a new synthetic methodology, researcher's and research team's knowledge, therapeutic potential, client requirements, etc. As chemists evolve in their skills and abilities, the complexity of target molecules and research projects increases (“Aloysuis”). Target molecule complexity was seen as a function of the open-endedness of the project-level problems; the more open-ended, the more complex the target molecule (“Xavier”). “Alberto” saw the open-endedness of the problem as a direct function of the number of possible synthetic pathways to a given target molecule; if every possible pathway was considered, an upwards of “thousands” of paths could be posited for any given target molecule.
Project-level problems give insight into the societal application of the target molecule's specific structure or functionality. In the opinion of “Aloysuis,” target molecules have most recently been limited to biologically active molecules. A review of Feature Articles from the Journal of Organic Chemistry in 2010 and 2011 shows that biologically active compounds including antibacterial, antibiotic, anticancer, antifungal, and disease inhibitors have been a key focus of synthetic organic chemistry research.
Project-level problems as discussed by the practising organic chemists were centered on total syntheses of target molecules; however, this is a limitation of the participant sample. A second category of project-level problems is ‘methodological.’ Three of the practising synthetic organic chemists mentioned this problem type; however, they stated that methodological research was not considered to be the main focus of their research initiatives. “Alberto” described methodology as…more of optimizing a, a reaction. So, you kind of create a new reaction, a new method to build up the structure. And, and, the problem is having probably poor yields or side products that you don't want, ah side products that are major. So, you try to reverse basically the ratio between the compounds. “Aloysuis” saw total synthesis and methodology problems working in tandem; “when you are working on total synthesis, without knowing, you would be working on methodology too.”
The definition of project-level problems, as reported by the participants, originates with the principal investigator, a faculty-level research advisor. The graduate student participants felt obligated to adopt the project-level problems and methods for solving those problems as directed by their research advisor. “Aloysuis”, a sixth year graduate student, and the two postdoctoral research associate participants noted that as their research careers progressed, their research advisors invited them to participate more in the definition of project-level problems and the direction necessary to solving the problems.
Synthetic planning problems
Two closely linked problems arise in the course of synthetic planning: (1) retrosynthetic analysis of the target molecule, and (2) developing several proposed synthetic pathways. Both these problems arise and are solved in tandem while planning syntheses. The overall ability to develop synthetic plans relies on a vast knowledge of reactions and the ability to foresee how reactions can lead to desired products. In developing a proposed synthetic route, a project-level problem begins to take shape and the reality of the synthesized product becomes more apparent.
Retrosynthetic analysis, a method introduced to the organic chemistry community by E. J. Corey, was explicitly discussed by five of the eight practising organic chemist participants. Corey received the Nobel Prize in 1990 for his idea of retrosynthetic analysis (see Corey, 1988, 1991; Corey and Cheng, 1995). Retrosynthetic analysis is a process of proposing possible “disconnections” (“Robert”), the reverse of forming bonds (see Fig. 1 for an “Edmund's” example of a retrosynthetic analysis).
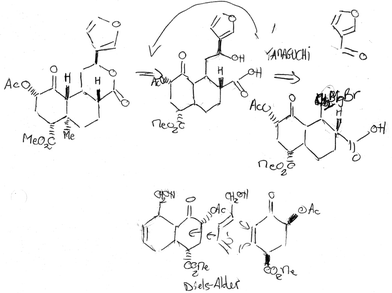 |
| Fig. 1 “Edmund's” partial retrosynthetic analysis of salvinorin A. “Edmund” uses double-tailed arrows to show his backward thinking from the target molecule to precursor molecules. He notes reaction names and reagents in this figure while considering how to synthesize the target molecule. | |
By making several disconnections within a target molecule, a researcher intends to disconnect the target molecule into a set of commercially available starting materials; this process may not be as easy as stated and may involve the development of new and modification of several known reaction types. The retrosynthetic analysis, with possible disconnections, becomes the foundation for proposing synthetic pathways.
If every possible disconnection were considered, the number of pathways to a given target would be extremely large. As discussed by the participants, several goals guide retrosynthetic analysis and the proposal of synthetic pathways. These goals include enantioselectivity, cost—inexpensive starting materials and reactants, efficiency—greatest yield, efficiency—shortest pathway (total number of steps), environmentally friendly (Anastas and Warner, 1998), intricacy—multiple bonds/stereocenters formed in one reaction (Fuchs, 2001), no unwanted products (i.e., side reactions), no use of protecting groups, and no waste (i.e., atom economy; Trost, 1991). As possible disconnections are considered and pathways are proposed, these goals inform and constrain the synthetic planning process.
As “Xavier” noted, once a pathway is set “then it's just a matter of getting the reactions to go.” “Xavier” is stating that at the conclusion of the planning process, the next step is to realize the planning in the laboratory through synthesis and experiment. The next section will describe the day-to-day routines in which the participants get their “reactions to go” and the problems that emerge during those routines.
Day-to-day problems
Day-to-day problems were the most frequently discussed problems by the eight participants; however, literature evidence of this problem type, beyond doctoral dissertations accounts, is sparse.
Day-to-day problems, as defined by the participants, are most typical of what would be considered a “problem” in the problem-solving literature (Hayes, 1989; Wheatley, 1984). “Aloysuis” defined a problem as a “challenge that keeps you from going to the next step;” he also described problems as “dead ends.” “Bernadette” stated that a “problem then is anything that needs to be fixed. Not working the way it should;” she also used the term “hiccup” to describe these kinds of problems. “Xavier” described day-to-day problems as anything “unexpected” or “hurdles” to be jumped. These three participant's problem definitions mirror two commonly referenced definitions from the problem-solving literature: Hayes (1989) has stated that “whenever there is a gap between where you and now and where you want to be, and you don't know how to find a way to cross the gap, you have a problem.” And, Wheatley (1984) has stated that problem solving is “what you do, when you don't know what to do.” Elements of both of these literature definitions were found in the participant's responses.
Problems of the day-to-day type arise in the context of four routine processes: (1) The physical setup of glassware, heating and stirring apparatuses, and vessels and tools for adding reagents to the reaction vessel (“Edmund”). (2) The purification of reactants and products (“Edmund,” & “Ignatius”). (3) The characterization of products (“Bernadette,” “Edmund,” “Ignatius,” & “Xavier”). (4) Property testing of products (e.g., biological activity; “Alberto,” “Bernadette,” “Claudia,” “Edmund”).
Within these four routine processes, the eight participants identified seven common day-to-day problems: (1) The formation of byproducts or unexpected products. This problem often emerges during the characterization process. Under certain circumstances a single unwanted product is the only product isolated. Under other circumstances, mixtures of byproducts and the intended product are obtained. Mixtures of products often lead to re-purification and re-characterization.
(2) Impure starting materials and reactants. Participants discussed that after discovering that the expected product was not obtained that they would often refer back to the characterization of the starting materials. By following the characterization backwards, being conscious of potential byproducts, participants were able to determine whether the starting material was impure or not the compound originally thought.
(3) Insoluble starting materials, reactants, or products. When all solvent was removed from the reaction mixture, the products solidified into an insoluble product that prohibited it to be characterized by common methods (i.e., liquid phase nuclear magnetic resonance spectroscopy).
(4) Instrument failure. Instruments being used in synthesis research are most commonly associated with characterization of the product; although, for those conducting their own biological activity testing, this also could include instruments used as such in that process.
(5) Reaction does not go to completion; low yields are obtained. This was determined by comparing their obtained product yields to those reported in the literature. The second way this is determined is through monitoring the reaction and noting residual starting materials.
(6) Reaction does not produce any product. This problem type is of two forms: First, no reaction is observed; only starting materials were obtained after a given amount of time determined by the participant and research advisor. Second, a reaction occurred but labile products were obtained (i.e., decomposition of starting materials and products occurred); these labile products were unable to be separated and characterized. In both these cases, some variable associated with the reaction conditions led to the lack of formation of products or the decomposition of products.
(7) The reaction is not reproducible from literature accounts or previous research experience. When the reproducibility problem arose, the participant was either repeating literature procedures or their own procedures and was unable to get the reaction to occur or go to completion (e.g., achieve a similar yield as previously obtained).
Implications for undergraduate education
Problem-based learning (PBL), most notably used in medical education (Hmelo, 1998), is a viable pedagogical method for exploring how to incorporate the practice of organic chemistry into the instruction of organic chemistry. PBL, when utilized in chemical education, has largely focused on creating problem scenarios for more “meaningful” learning (Jonassen, 1997, 2003) of the traditional canon of organic chemical knowledge (American Chemical Society, 1972, 1976) rather than taking “advantage of the new directions and existing research that chemists are pursuing” to develop new teaching methods (Jacobs, 2003). Problem choice is critical to PBL instructional practices (Norman, 1988). The types of problems that will be proposed in this section expand upon the traditional knowledge base of sophomore-level organic chemistry and include education on the nature of organic chemistry practice; these problems “begin to focus our attention on teaching the process of problem solving instead of focusing so heavily on the outcomes of problem solving” (Cartrette, 2003, p. 216).
Problem-based learning “situates learning in complex problem-solving contexts. It provides students with opportunities to consider how the facts they acquire relate to a specific problem at hand” (Hmelo-Silver, 2004, p. 261). The three problem types found in this study provide the context (Jonassen, 2003) necessary for instructional designers to develop instructional problems centered on “authentic” problem-solving contexts (Overton and Potter, 2008). The implications of each problem type on developing instructional problems will be discussed.
Project-level problems
In the opinion of the authors, there is difficulty in describing how a student may encounter a project-level problem given that research advisors (i.e., course instructors in the classroom) most always are defining and directing the nature of research problems. Nevertheless, the broader implication is in the societal application of the chosen problems. As was previously noted, each project-level problem resides in a given societal application; such a focus provides additional opportunity for meaningful learning (Bretz, 2001; Edelson, 1998; Jonassen and Land, 2000; Novak, 1977). When developing instructional problems, target structures for multiple-step synthesis problems should be chosen with the potential societal application in mind. For example, Scheme 2 shows a valuable target, 1, for developing new antibiotics and non-specific kinase inhibitors (Lackner et al., 2005), a medicinal chemistry focused target.
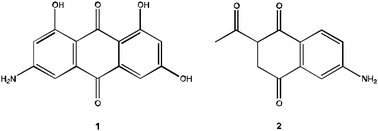 |
| Scheme 2 Sample target molecules for multiple-step synthesis problems. | |
The analogous target, 2, could be given as a viable multiple-step synthetic target appropriate for sophomore-level students; synthesis of target 1 is beyond the knowledge of a sophomore-level organic chemistry student. However used in a problem prompt, 1 and 2 should include a discussion on where the molecule, 1, was discovered, the importance of synthesizing analogues in medicinal chemistry (if this has not already been presented), data on the biological activity, and references to appropriate literature articles discussing the target molecule. Some have attempted to include such societal applications in their curriculum (Doxsee, 1990; Ferguson, 1980; Harrison, 1989; Kelley and Gaither, 2007); however, broad based adoption of this implication has not occurred in the organic chemistry curriculum.
Synthetic planning problems
This type of problem is most commonly thought of in terms of instructional problems already in use; sophomore-level students should be familiar with multiple-step synthesis problems. The development of synthetic pathways from simultaneous forward and backwards thinking (Cartrette, 2003) is a common problem-solving behavior. However, this problem type, as it is currently used in instruction, does not always embrace and give due diligence to importance of synthetic planning in research. It should be argued that retrosynthetic analysis is a key tenet to the field; it is rooted in the fundamental idea of beginning with the end in mind. It is a type of thinking that has application in reverse engineering, artistic sculpting, and party planning to name a few. Of course, the tools and knowledge necessary to solve each of those problems is different; the implications for teaching this critical problem-solving skill are enormous.
Organic chemistry instructors give more “predict-the-product” problems than multiple-step syntheses problems (Raker and Towns, 2010). Why? Because they are easier to grade? Multiple-step syntheses often have multiple correct answers that could complicate a rigid grading scheme. But, very seldom, if ever, do practising organic chemists recite their knowledge of chemical reactions as a canon of individual reactions. References materials are available to practising chemists that have a tangible organization of their knowledge (Caruthers and Coldham, 2004; Gallego and Sierra, 2004; Li, 2003; Mackie et al., 1999); however, each reaction unto itself is not the focus of achieving research goals. Multiple-step synthesis problems should become more of a focus in encouraging students to discover how the reactions, in combination, can and are used to synthesize complex target molecules (Bhattacharyya and Bodner, 2005). Such a focus could help to alleviate the perception that learning chemistry is “an enormous and onerous chore” of memorization (Hendrickson, 1978).
Day-to-Day problems
This last problem type has the most promise for how sophomore-level organic chemistry instruction could be redesigned. The practising organic chemist participants spent the most time working on these types of problems in their research. It must be noted that experimental evidence, such as spectroscopic data, is at the heart of discovering many problems of this type. If problems such as these are to be incorporated into the curriculum, spectroscopy and other data measures (e.g., product distribution ratios) must take a more central role in lecture and be moved toward the beginning of the two-semester (yearlong) instructional sequence. Such a focus on experimental evidence is often found at the graduate level, the last stage of classroom training for future chemists, as shown in several graduate-level textbooks (Boger, 1999; Caruthers and Coldham, 2004; March and Smith, 2001; Norman and Coxon, 1993; Smith, 1984). We believe there is great utility in teaching students, at the beginning of their undergraduate organic chemistry education, the manner in which we come to know what we have synthesized in a given reaction and underlying chemical principles (e.g., resonance, induction, aromaticity). When students have the opportunity to make discoveries and develop their own conclusions from the experimental data, more meaningful learning can occur (Spencer, 1999).
Knowledge of infrared (IR) spectroscopy, mass spectrometry (Mass Spec), and nuclear magnetic resonance (NMR) spectroscopy taught earlier in the course can then serve as a foundation for posing problems where students are to discover the formation of unexpected products or impure starting materials and reagents. For example, Fig. 2 shows a two-step reaction and final product 1H NMR data.
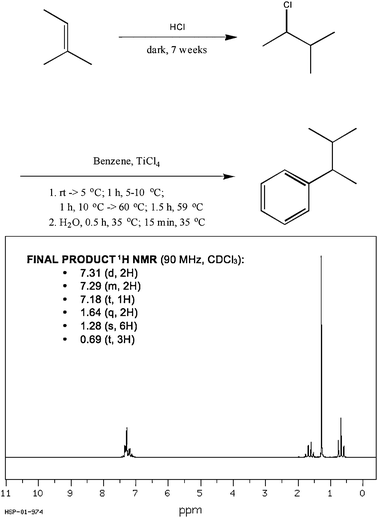 |
| Fig. 2 Sample day-to-day problem for sophomore-level organic chemistry students. Students are asked to consider the reaction scheme, predicted products, and spectroscopic data to determine if the predicted product was obtained. | |
A student would be asked to confirm if the product was or was not obtained. The product shown in Fig. 2 was not obtained. The spectroscopic data (and knowledge of hydrohalogentation reaction mechanisms) should lead a student to conclude that tert-pentylbenzene was the obtained product; in other words, the intended intermediate, 2-chloro-3-methyl-butane, was not obtained. (This problem has been pilot-tested with sophomore-level organic chemistry students who were able to successfully generate solutions.) This type of problem reaffirms the role data has in understanding chemical reactions while providing students with real life experiences of how the chemistry they are taught is utilized.
Summary
The results of this study of eight practising organic chemists provided a foundation for considering how undergraduate organic chemistry instruction can better mirror the practice of science. The three problem types found (i.e., project-level, synthetic planning, and day-to-day) provide a framework for the reform of current instructional problems and practices. Further research is need to explore the impact of new curricular problems on student understanding of the nature of organic chemistry practice and the traditional sophomore-level organic chemistry topics.
Acknowledgements
We would like to thank the eight practising organic chemist participants who took upwards of two hours out of their busy graduate and postdoctoral lives to share their research experiences. In addition, we would like to thank Ms. Nicole Becker, Dr Aaron Bruck, and Mr. Daniel Nicponski for their thoughtful review of this research and subsequent manuscripts.
References
- American Chemical Society, (1972), Report of the organic subcommittee of the curriculum committee, J. Chem. Educ., 49(11), 761–763.
- American Chemical Society, (1976), Report of the organic subcommittee of the curriculum committee, part II, J. Chem. Educ., 53(1), 25–26.
- American Chemical Society, (2008), Undergraduate professional education in chemistry: ACS guidelines and evaluation procedures for bachelor's degree programs. Washington, D.C.: American Chemical Society.
- Anastas P. T. and Warner J. C., (1998), Green Chemistry: Theory and Practice. New York: Oxford University Press.
- Bhattacharyya G. and Bodner G. M., (2005), “It gets me to the product”: How students propose organic mechanisms, J. Chem. Educ., 82(9), 1402–1407.
- Bodner G. M., (1991), A view from chemistry. In M. U. Smith (Ed.), Toward a unified theory of problem solving: Views from the content domains (pp. 21–33). Hillsdale, NJ: Lawrence Erlbaum Associates.
- Bodner G. M. and Domin D. S., (2000), Mental models: The role of representations in problem solving in chemistry. University Chemistry Education, 4(1), 24–30.
- Boger D. L., (1999), Modern organic synthesis: Lecture notes. San Diego, CA: TSRI Press.
- Bowen G. M. and Roth W.-M., (2000), Conducting ecology research: Commonalities of practice in “communities” of elementary students and professional researchers. Paper presented at the Annual Conference of the Canadian Society for the Study of Education, Edmonton, Alberta.
- Bretz S. L., (2001), Novak's theory of education: Human constructivism and meaningful learning, J. Chem. Educ., 78, 1107.
- Cartrette D. P., (2003), Using spectral analysis to probe the continuum of problem solving ability among practicing organic chemists. West Lafayette: Masters of Science, Purdue University.
- Caruthers W. and Coldham I., (2004), Modern methods of organic synthesis. New York: Cambridge University Press.
- Committee on Professional Training, (2002), Undergraduate research ACS-CPT supplement Retrieved January 6, 2010.
- Corey E. J., (1988), Retrosynthetic thinking—Essentials and examples, Chem. Soc. Rev., 17, 111–133.
- Corey E. J., (1991), The logic of chemical synthesis: Multistep synthesis of complex carbodenic molecules, Angew. Chem., Int. Edn. Engl., 30(5), 455–465.
- Corey E. J. and Cheng X., (1995), The logic of chemical synthesis. New York: Wiley.
- Deslongchamps P., (1984), The concept of strategy in organic synthesis, Aldrichim. Acta, 17(3), 59–71.
- Doxsee K. M., (1990), Development of an advanced synthesis laboratory course in Nobel prize winning chemistry, J. Chem. Educ., 67(12), 1057–1060.
- Edelson D. C., (1998), Realising authentic science learning through the adaptation of scientific practice. In B. J. Fraser and K. G. Tobin (Ed.), International Handbook of Science Education (pp. 317–331), Great Britain: Kluwar Academic Publishers.
- Ferguson L. N., (1980), Content and structure of the chemistry course: New approaches, J. Chem. Educ., 57(1), 46–48.
- Fuchs P. L., (2001), Increase in intricacy—A tool for evaluating organic syntheses, Tetrahedron, 57, 6855–6875.
- Gallego M. G. and Sierra M. A., (2004), Organic reaction mechanisms: 40 sovled cases. New York: Springer.
- Harrison A. M., (1989), Medicinal chemistry/pharmacology in sophomore organic chemistry, J. Chem. Educ., 66(10), 825–826.
- Hayes J. R., (1989), The complete problem solver (2nd ed.), Hillsdale, NJ: Lawrence Erlbaum Associates.
- Hendrickson J. B., (1978), A logical characterization of organic chemistry, J. Chem. Educ., 55(4), 216–220.
- Heyworth R. M., (1999), Procedural and conceptual knowledge of expert and novice students for the solving of a basic problem in chemistry, Int. J. Sci. Educ., 21(2), 195–211.
- Hmelo C. E., (1998), Problem-based learning: Effects on the early acquisition of congitive skill in medicine, J. Learn. Sci., 7(2), 173–208.
- Hmelo-Silver C. E., (2004), Problem-based learning: What and how do students learn? Educ. Psychol. Rev., 16(3), 235–266.
- Jacobs M., (2003), Educating chemists for the future, C&E News, 81(31), 34–35.
- Jonassen D. H., (1997), Instructional design models for well-structured and ill-structured problem-solving learning outcomes, Educ. Technol. Res. Dev., 45(1), 65–94.
- Jonassen D. H., (2003), Learning to solve problems: An instructional design guide. San Francisco: Pfeiffer.
- Jonassen D. H. and Hernandez-Serrano J., (2002), Case-based reasoning and instructional design: Using stories to support problem solving, Educ. Technol. Res. Dev., 50(2), 65–77.
- Jonassen D. H. and Land S. M., (2000), Theoretical foundations of learning environments. Mahwah, New Jerseu: Lawrence Erlbaum.
- Jonassen D. H., Strobel J. and Lee C. B., (2006), Everyday problem solving in engineering: Lessons for engineering educators, J. Eng. Educ., 95(2), 139–151.
- Kelley C. and Gaither K. K., (2007), Integrating pharmacology into the organic chemistry course: Understanding the synergy of biology and chemistry, J. Coll. Sci. Teach., 30(7), 450–453.
- Lackner B., Bretterbauer K. and Falk H., (2005), An efficient route to emodic amine and analogous O-methyl protected derivatives starting from emodin, Monatsch. Chem. Chem. Month., 136, 1629–1639.
- Li J. J., (2003), Name reactions: A collection of detailed reaction mechanisms. New York: Springer.
- Lincoln Y. S. and Guba E. G., (1985), Naturalistic Inquiry. Beverly Hills, CA: Sage Publications.
- Lindsay H. A. and McIntosh M. C., (2000), Early exposure of undergraduates to the chemistry research environment: A new model for research universities, J. Chem. Educ., 77(9), 1174–1175.
- Mackie R. K., Smith D. M. and Aiken R. A., (1999), Guidebook to organic synthesis (3rd ed.), New York: Prentice Hall.
- March J. and Smith M. B., (2001), March's advanced organic chemistry: Reactions, mechanisms, and structure (5th ed.). New York: Wiley & Sons.
- Nersessian N. J., (1995), Should physicists preach what they practice? Sci. Educ., 4, 203–226.
- Norman G. R., (1988), Problem-solving skills, solving problems and problem-based learning, Med. Educ., 22, 279–286.
- Norman R. O. C. and Coxon J. M., (1993), Principles of organic synthesis. New York: Blackie Academic & Professional.
- Novak J. D., (1977), A Theory of Education. Ithaca, New York: Cornell University.
- Overton T. and Potter
N., (2008), Solving open-ended problems, and the influence of cognitive factors on students success, Chem. Educ. Res. Pract., 9, 65–69.
- Patton M. Q., (2002), Qualitative research & evaluation methods (3rd ed.). Thousand Oaks, CA: Sage.
- Raker J. R. and Towns M. H., (2010), Benchmarking problems used in second year level oragnic chemistry instruction, Chem. Educ. Res. Pract., 11(1), 25–32.
- Russell C. B. and Weaver G. C., (2011), A comparative study of traditional, inquiry-based, and research-based laboratory curricula: Impacts on understanding of the nature of science, Chem. Educ. Res. Pract., 12(1), 57–67.
- Samarapungaven A., Westby E. L. and Bodner G. M., (2006), Contextual epistemic development in science: A comparison of chemistry students and research chemists, Sci. Educ., 90(3), 468–495.
- Smith M. B., (1984), Organic synthesis. New York: McGraw-Hill.
- Society Committee on Education, (2003), Exploring the molecular vision: American Chemical Society.
- Spencer J. N., (1999), New directions in teaching chemistry: A philosophical and pedagogical basis, J. Chem. Educ., 76(4), 566–569.
- Trost B. M., (1991), The atom economy-A search for synthetic efficiency, Science, 254(5037), 1471–1477.
- Wheatley G. H., (1984), Problem solving in school mathematics. West Lafayette, IN: Purdue University, School Mathematics and Science Center.
- Zoller U., (1987), The fostering of question-asking capability, J. Chem. Educ., 64(6), 510–512.
|
This journal is © The Royal Society of Chemistry 2012 |