DOI:
10.1039/C2RA21772D
(Paper)
RSC Adv., 2012,
2, 10564-10574
What causes the low viscosity of ether-functionalized ionic liquids? Its dependence on the increase of free volume
Received
10th August 2012
, Accepted 6th September 2012
First published on 7th September 2012
Abstract
A series of 12 ether-functionalized room temperature ionic liquids (RTILs) and their alkyl counterparts (short of an oxygen atom) were prepared and characterized to investigate the reason for the alkoxy chain effect on decreasing viscosity and how to best apply it. In addition to the ability of the alkoxy chains to decrease viscosity (ηO/η, as low as 0.594) and its activation energy (ΔEη, as low as -4.14 kJ mol−1), they were also found to be able to increase the total free volume (ΔFVO, up to 4.93 mL mol−1), by using density and surface tension. Furthermore, both the obtained ηO/η and ΔEη values for the 12 pairs of RTILs show a strong decreasing dependence on ΔFVO. Accordingly, the reason for the alkoxy chain effect on decreasing viscosity was proposed to be due to the ability of the highly flexible alkoxy chains to increase the total free volume, which offers the convenience of transport for the adjacent molecules. To maximize this effect, the alkoxy groups were supposed to contain a rod-like alkyl tail and a short –CH2– spacer between the ether O atom and the cationic N atom, and be in an environment without hydrogen bond donors. Generally, the ether-functionalized pyrrolidinium and ammonium RTILs possess high conductivity (up to 4.97 mS cm−1 at 25 °C) and wide electrochemical windows (∼5.75 V), indicating their significant promise for electrochemical applications.
Introduction
Over the past three decades, room temperature ionic liquids (RTILs) have been extensively studied and regarded as an environmentally preferable alternative to reaction media,1 supporting electrolytes2 as well as functional materials.3 Since RTILs are composed entirely of ions, they are endowed with some unique features in most cases, such as negligible vapor pressure, non-flammability, high thermal stability, wide liquid range and intrinsic ionic conductivity. These are all considered environmentally friendly characteristics. In addition, the properties of RTILs can be custom-designed by changing either the cation or anion species to meet specific requirements.4 As a result, RTILs have been applied successfully in various fields, such as organic synthesis,1,5 transition metal catalysis,1,6 biotechnology,4,7 ionothermal synthesis,8 nano-materials,8,9 separation techniques,10 lubricants,11 lithium-ion batteries,12 dye-sensitized solar cells,13 supercapacitors,14 fluorescent materials,15 magnetic fluids,16 and many more.
However, RTILs are generally tens and even hundreds of times more viscous than the traditional organic solvents. High viscosity not only decreases the possibility of its applications, such as heterogeneous catalysis and supporting electrolytes, in these fields that require fast mass and ion transport, but also leads to operational difficulties in filtration, extraction and decantation. Therefore, in most cases, the required viscosity of RTILs should be as low as possible. Generally, a low-viscosity RTIL is characteristic of well-delocalized positive and negative charges,17 low-symmetry ions18 and highly flexible side chains.19 These structural features are relevant to weak Coulomb interactions and low effective packing between ions, thereby leading to low viscosity.
Recently, ether groups have been well known for their ability to form low viscosity and high conductivity RTILs,19a,20 as well as low crystallinity and high conductivity polymer electrolytes.21 For example, when the C4H9– group in 1-butyl-1-methylpyrrolidinium CF3BF3− was replaced by an isoelectronic CH3OC2H4– group, its viscosity was reduced from 137 to 87 cP, and its conductivity was increased from 3.3 to 4.3 mS cm−1 at 25 °C.20e Up to now, many ether-functionalized RTIL species have been developed and extensively studied, such as imidazolium cations,20c,22 ammonium cations,20d–g phosphonium cations,20i guanidinium cations,20j,k sulfonium cations,20l pyrazolium cations,20m as well as perfluoroalkylborate anions.20n However, with regard to the mechanism of the alkoxy chain effect on decreasing viscosity, it was often only vaguely related to their high rotational flexibility,20d,e which results from the smaller atomic radius of the O atom compared to a C atom, and that the ether O atom only forms bonds with two atoms rather than four atoms, as is the case for the C atom.23 More recently, molecular dynamics simulations indicated that the more flexible alkoxy chains can cause a less effective assembly, and therefore decrease the viscosity, in comparison with alkyl chains.19a However, the alkoxy chain effect on viscosity is highly variable and strongly depends on the RTIL and alkoxy chain structures. For example, the ability of ether groups to reduce viscosity is much more effective in ammonium RTILs than in imidazolium RTILs,20c–e,20j,20n and is much more effective for the C2H5OC2H4– group than for the CH3OC2H4– group.20g,h These unusual phenomena provide access to conduct an exploratory study on the alkoxy chain effect on viscosity, and to see what exactly causes the effect and how to maximize it.
Recently, hole theory indicated that (1) the ions in RTILs cannot pack closely and lots of holes between ions may come into existence; (2) the bulk volume of a RTIL consists of the inherent volume and the total volume of holes; and (3) a large total hole volume is of advantage to a high rate of mass transport and low viscosity.24 Accordingly, the alkoxy chain effect on viscosity was conceived to be relevant as alkoxy chains can increase the total free volume and provide more available holes for the convenience of mass transport and low viscosity. In order to test this assumption, 12 ether-functionalized RTILs and their alkyl counterparts short of an ether O unit were synthesized and characterized, including viscosity, the activation energy of viscosity, density, molar volume, surface tension and the total free volume, and the alkoxy chain effect on viscosity and its activation energy and the total free volume was also calculated and comparatively studied.
Results
Scheme 1 presents the structures and abbreviations of the RTILs employed in this study. In detail, MPyrr, EDMAm, MIm and Py stand for the cationic species of N-methylpyrrolidinium, N,N-dimethylethanammonium, N-methylimidazolium and pyridinium, respectively; and Pr–, Bu–, MOE–, EOM– and EOE– stand for the side chains of CH3CH2CH2–, CH3CH2CH2CH2–, CH3CH2OCH2–, CH3OCH2CH2– and CH3CH2OCH2CH2–, respectively. 1H-NMR and FT-IR spectra (see Experimental Section for details) were recorded to confirm the structures of the synthesized compounds. Several basic and desired physicochemical properties of the RTILs were characterized and comparatively studied, including viscosity (η), the activation energy of viscosity (Eη), density (ρ) and molar volume (MV), as listed in Table 1. In addition, the group contribution of the incorporated ether O atom to viscosity (ηO/η), its activation energy (ΔEη), molar volume (ΔMVO) and the total free volume (ΔFVO) were also calculated and are summarized in Table 2. The total free volume (∑ν) in the isomeric RTILs, i.e., [MOE-MPyrr]NTf2vs. [EOM-MPyrr]NTf2 and [MOE-EDMAm]NTf2vs. [EOM-EDMAm]NTf2, was calculated by the surface tension method to confirm the rationality of the obtained ΔFVO values, as shown in Table 3. Besides, the high conductivity (up to 4.97 mS cm−1 at 25 °C) and wide electrochemical windows (5.68–5.79 V) of the ether-functionalized pyrrolidinium and ammonium RTILs, as shown in Table 4, imply their promising applications in the field of electrochemistry.
 |
| Scheme 1 The structures and abbreviations of the RTILs employed in this study. | |
Table 1 Some basic physicochemical properties of the alkoxy- and alkyl-substituted RTILs at 25 °C
RTIL |
η
a cP |
E
η
b kJ mol−1 |
ρ
c g mL−1 |
MWd g mol−1 |
MVe mL mol−1 |
Viscosity.
Activation energy of viscosity.
Density.
Molecular weight.
Molar volume.
Literature value, 53 cP.20e
Literature value, 1.4539 g mL−1.20e
Literature value, 76 cP.20e
Literature value, 1.3931 g mL−1.20e
Literature value, 51.0 cP.25
Literature value, 1.4366 g mL−1.25
Literature value, 108.3 cP.26
Literature value, 1.20143 g mL−1.26
|
[Pr-MPyrr]NTf2 |
62.4 |
28.83 |
1.4283 |
408.38 |
285.92 |
[MOE-MPyrr]NTf2 |
54.8f |
27.76 |
1.4546g |
424.38 |
291.75 |
[EOM-MPyrr]NTf2 |
38.7 |
25.29 |
1.4375 |
424.38 |
295.18 |
[Bu-MPyrr]NTf2 |
79.6h |
31.07 |
1.3950i |
422.41 |
302.80 |
[EOE-MPyrr]NTf2 |
51.8 |
28.27 |
1.4104 |
438.41 |
310.84 |
[Pr-EDMAm]NTf2 |
75.1 |
31.53 |
1.4039 |
396.37 |
282.33 |
[MOE-EDMAm]NTf2 |
63.6 |
29.96 |
1.4326 |
412.37 |
287.85 |
[EOM-EDMAm]NTf2 |
44.6 |
27.39 |
1.4134 |
412.37 |
291.76 |
[Bu-EDMAm]NTf2 |
99.3 |
33.49 |
1.3719 |
410.40 |
299.15 |
[EOE-EDMAm]NTf2 |
61.8 |
29.89 |
1.3870 |
426.40 |
307.43 |
[Pr-MIm]NTf2 |
45.3 |
27.68 |
1.4756 |
405.34 |
274.70 |
[MOE-MIm]NTf2 |
49.6 |
29.04 |
1.5088 |
421.34 |
279.26 |
[Bu-MIm]NTf2 |
51.7j |
28.60 |
1.4367k |
419.36 |
291.89l |
[EOE-MIm]NTf2 |
45.1 |
28.07 |
1.4584 |
435.36 |
298.52 |
[Pr-MIm]BF4 |
78.9 |
33.38 |
1.2398 |
212.00 |
171.00 |
[MOE-MIm]BF4 |
90.2 |
35.07 |
1.2996 |
228.00 |
175.44 |
[Bu-MIm]BF4 |
111.3l |
36.56 |
1.2025m |
226.02 |
187.96 |
[EOE-MIm]BF4 |
103.0 |
36.27 |
1.2485 |
242.02 |
193.85 |
[Bu-Py]NTf2 |
61.9 |
30.20 |
1.4490 |
416.36 |
287.34 |
[EOE-Py]NTf2 |
52.3 |
29.34 |
1.4667 |
432.36 |
294.78 |
[Bu-Py]BF4 |
170.7 |
40.77 |
1.2152 |
223.02 |
183.53 |
[EOE-Py]BF4 |
157.5 |
40.56 |
1.2566 |
239.02 |
190.21 |
Table 2 Alkoxy chain effect on η (ηO/η), Eη (ΔEη), MV (ΔMVO) and the total free volume (ΔFVO) of RTILs, by comparing the ether-functionalized RTILs with their alkyl counterparts short of an ether O unit
Alkoxy/alkyl RTIL pair |
η
O/η |
ΔEη kJ mol−1 |
ΔMVO mL mol−1 |
ΔFVO mL mol−1 |
[MOE-MPyrr]NTf2/[Pr-MPyrr]NTf2 |
0.878 |
−1.07 |
5.83 |
1.33 |
[EOM-MPyrr]NTf2/[Pr-MPyrr]NTf2 |
0.620 |
−3.54 |
9.26 |
4.76 |
[EOE-MPyrr]NTf2/[Bu-MPyrr]NTf2 |
0.651 |
−2.80 |
8.04 |
3.54 |
[MOE-EDMA]NTf2/[Pr-EDMA]NTf2 |
0.847 |
−1.57 |
5.52 |
1.02 |
[EOM-EDMA]NTf2/[Pr-EDMA]NTf2 |
0.594 |
−4.14 |
9.43 |
4.93 |
[EOE-EDMA]NTf2/[Bu-EDMA]NTf2 |
0.622 |
−3.60 |
8.28 |
3.78 |
[MOE-MIm]NTf2/[Pr-MIm]NTf2 |
1.095 |
1.36 |
4.56 |
0.06 |
[EOE-MIm]NTf2/[Bu-MIm]NTf2 |
0.872 |
−0.53 |
6.63 |
2.13 |
[MOE-MIm]BF4/[Pr-MIm]BF4 |
1.143 |
1.69 |
4.44 |
−0.06 |
[EOE-MIm]BF4/[Bu-MIm]BF4 |
0.925 |
−0.29 |
5.89 |
1.39 |
[EOE-Py]NTf2/[Bu-Py]NTf2 |
0.845 |
−0.86 |
7.44 |
2.94 |
[EOE-Py]BF4/[Bu-Py]BF4 |
0.923 |
−0.21 |
6.68 |
2.18 |
Table 3 Surface tension and the total free volume of the isomeric RTILs
RTILa |
γ
a mN m−1 |
∑νb mL mol−1 |
Δ∑νc mL mol−1 |
Surface tension at 25 °C.
The total free volume calculated by eqn (3).
The difference of the total free volume between the isomeric RTILs.
|
[MOE-MPyrr]NTf2 |
38.06 |
29.06 |
|
[EOM-MPyrr]NTf2 |
35.51 |
32.25 |
3.19 |
[MOE-EDMAm]NTf2 |
37.04 |
30.27 |
|
[EOM-EDMAm]NTf2 |
34.47 |
33.72 |
3.45 |
Table 4 Electrochemical properties of pyrrolidinium and ammonium RTILs
RTIL |
κ
a mS cm−1 |
Ecathodicb V |
Eanodicc V |
EWd V |
Conductivity at 25 °C.
Cathodic potential limit.
Anodic potential limit.
Electrochemical windows.
Literature value, 3.7 mS cm−1.20e
Literature value, 2.6 mS cm−1.20e
Literature value, 3.1 mS cm−1.20d
|
[Pr-MPyrr]NTf2 |
3.75 |
−3.05 |
2.76 |
5.81 |
[MOE-MPyrr]NTf2 |
3.72e |
−3.06 |
2.71 |
5.77 |
[EOM-MPyrr]NTf2 |
4.97 |
−3.05 |
2.71 |
5.76 |
[Bu-MPyrr]NTf2 |
2.62f |
−3.03 |
2.74 |
5.77 |
[EOE-MPyrr]NTf2 |
3.23 |
−3.05 |
2.65 |
5.70 |
[Pr-EDMA]NTf2 |
2.93 |
−3.07 |
2.76 |
5.83 |
[MOE-EDMA]NTf2 |
3.10g |
−3.05 |
2.74 |
5.79 |
[EOM-EDMA]NTf2 |
4.23 |
−3.08 |
2.71 |
5.79 |
[Bu-EDMA]NTf2 |
1.86 |
−3.05 |
2.75 |
5.80 |
[EOE-EDMA]NTf2 |
2.78 |
−3.01 |
2.67 |
5.68 |
The viscosity of the alkoxy- and alkyl-substituted RTILs was determined using a BROOKFIELD DV-II+ Pro viscometer that is accurate within ±1.0%. The viscosity (η) values obtained at 25 °C fall in the range from 38.7 to 170.7 cP, as listed in Table 1. As can be expected, the addition of an ether O atom into an alkyl side chain exhibits a significant effect on the viscosity of RTILs. For example, by replacing a Pr– group with an EOM– group, the viscosity can be reduced from 62.4 cP of [Pr-MPyrr]NTf2 to 38.7 cP of [EOM-MPyrr]NTf2. In order to quantify the ether O atom's contribution to the viscosity of RTILs, the change rate of viscosity was calculated and expressed as ηO/η in Table 2. For example, the ratio of the viscosity of [EOM-MPyrr]NTf2 (38.7 cP) to that of [Pr-MPyrr]NTf2 (62.4 cP) was calculated to be 38.7/62.4 = 0.620, which represents the contribution of the O atom in the EOM– group to the viscosity of [R-MPyrr]NTf2. The ηO/η values presented in Table 2 are highly variable and range from 1.143 to 0.594. Generally, the ηO/η values (0.878–0.594) in pyrrolidinium- and ammonium-based RTILs are all less than 1, indicating that the alkoxy chains in these RTILs are all helpful to decrease viscosity, especially for the EOM- group (0.620–0.594). However, the ηO/η values less than 1 in imidazolium and pyridinium RTILs are limited to the EOE- group (0.925–0.845), but still larger than those in pyrrolidinium and ammonium RTILs, and the MOE- group in imidazolium RTILs (1.095–1.143) even tends to increase viscosity.
The temperature dependence of the viscosity of the RTILs was also investigated over a temperature range from 10 to 80 °C. As typically shown in Fig. 1, the variation of the viscosity with temperature agrees well with an Arrhenius-type behaviour (eqn (1)),25–27 where η is viscosity (cP), A is a constant, Eη is activation energy (kJ mol−1), R is the universal gas constant (8.314 J K−1 mol−1), and T is the absolute T/K.
![Typical Arrhenius plots of viscosity for two RTILs (●: [Pr-MPyrr]NTf2, ■: [EOM-MPyrr]NTf2).](/image/article/2012/RA/c2ra21772d/c2ra21772d-f1.gif) |
| Fig. 1 Typical Arrhenius plots of viscosity for two RTILs (●: [Pr-MPyrr]NTf2, ■: [EOM-MPyrr]NTf2). | |
According to eqn (1), the Eη values for all of the studied RTILs were calculated and summarized in Table 1, with the linear fitting parameter (R2) larger than 0.995. In addition, the change in the Eη values of the RTILs by incorporating an additional ether O unit was also calculated as ΔEη and summarized in Table 2. For example, the difference of the Eη values between [EOM-MPyrr]NTf2 (25.29 kJ mol−1) and [Pr-MPyrr]NTf2 (28.83 kJ mol−1) was calculated to be 25.29–28.83 = −3.54 kJ mol−1, which represents the contribution of the ether O unit in EOM- group to Eη value of [R-MPyrr]NTf2. In theory, viscosity flow can be treated as a process depending on the activation energy, and the Eη value can be regarded as the energy barrier to be overcome by mass transport.28 As a consequence, the change in viscosity should be consistent with the change in the Eη value. As expected from Fig. 2, the ΔEη value generally decreases with decreasing ηO/η value. As a result, the ΔEη values can also be used, as well as ηO/η, to characterize the effect of an ether O atom on the viscosity of RTILs.
 |
| Fig. 2 The changing relationship between ηO/η and ΔEη. | |
The density (ρ) of the studied RTILs was measured by a DMA 35 portable density meter at 25 °C, and the resulting data in Table 1 fall in the range from 1.2025 to 1.5088 mL mol−1. The corresponding molar volumes (MV) were calculated as their molecular weight divided by density, as listed in Table 1. Theoretically, the molecular volume can be calculated using the group additivity method.29 For example, a mean contribution per methylene to the molecular volume of RTILs was calculated to be 27.8 Å−3 (16.74 mL mol−1) for [CnMIm]alanine series (n = 2, 3, 4, 5, 6)30 and 27.5 Å−3 (16.56 mL mol−1) for [CnMIm]BF4 series (2, 4, 6, 8, 10) and [CnMIm]NTf2 series (1, 2, 4, 6, 8, 10, 12).31 These values are close to our results, i.e., [Bu-MPyrr]NTf2vs. [Pr-MPyrr]NTf2 (302.80–285.92 = 16.88 mL mol−1), [Bu-EDMAm]NTf2vs. [Pr-EDMAm]NTf2 (299.15–282.33 = 16.82 mL mol−1), [Bu-MIm]NTf2vs. [Pr-MIm]NTf2 (291.89–274.70 = 17.19 mL mol−1) and [Bu-MIm]BF4vs. [Pr-MIm]BF4 (187.96–171.00 = 16.96 mL mol−1). It was argued that the contribution per methylene to the molar volume behaves in a regular manner, and should be the same or at least close to a constant.29a,31
Using the same method, the contribution of an additional ether O unit to molar volume was also calculated as ΔMVO (mL mol−1), as listed in Table 2. The ΔMVO values of the 12 pairs of RTILs fall in a wide range 4.44–9.43 mL mol−1, and yield the largest relative difference up to 112% = (9.43–4.44)/4.44. In other words, the ΔMVO value for [EOM-EDMA]NTf2/[Pr-EDMA]NTf2 (9.43 mL mol−1) is even larger than twice that for [MOE-MIm]BF4/[Pr-MIm]BF4 (4.44 mL mol−1). Based on hole theory, the bulk volume of a liquid consists of inherent volume and the total volume of the holes between molecules.24f,g Accordingly, the ΔMVO values should contain excess free volume, especially for the larger ones. Although the inherent volume of the ether O unit is unclear, it should be a constant or at least an approximation, as noted above. Generally, the larger the ΔMVO value is, the larger the excess free volume should be. In fact, the proposed excess free volume is consistent with “the less effective assembly” of the highly flexible alkoxy chains.19a
Actually, the ΔMVO values can be well correlated with the rotational flexibility of the alkoxy chains in different conditions. Fig. 3 shows the increasing dependence of the ΔMVO values on the alkoxy chain rotational flexibility. Generally, around the ether O atom, there are two main rotational models,23 which seem to be affected by hydrogen bonds, the O-terminal alkyl group, and the spacer length between the ether O atom and the cationic nitrogen atom. First of all, either intra- or intermolecular hydrogen bonds tend to restrict the rotational motion and increase the rigidity of the side chains.22,32 As shown in Fig. 3, the [MOE-MIm]NTf2/[Pr-MIm]NTf2 pair (★) exhibits a lower ΔMVO value of 4.56 mL mol−1 than 5.83 mL mol−1 of [MOE-MPyrr]NTf2/[Pr-MPyrr]NTf2 (◆), since the alkoxy chain rotational flexibility in imidazolium RTILs can be weakened by the hydrogen bond between the ether O atom and imidazolium ring hydrogen atom. Similar results could also be observed in pyridinium-based RTILs, and the ΔMVO value of [EOE-Py]NTf2/[Bu-Py]NTf2 pair (7.44 mL mol−1, ▲) is a bit larger than that of [EOE-MIm]NTf2/[Bu-MIm]NTf2 pair (6.63 mL mol−1, ▼), which should be ascribed to the relatively weaker hydrogen-bond donor in the former than that in the latter. Secondly, the ΔMVO values obtained from the MOE-substituted RTILs (e.g. 5.83 mL mol−1 of [MOE-MPyrr]NTf2/[Pr-MPyrr]NTf2, ◆) are always smaller than those from EOE-substituted RTILs (e.g. 8.04 mL mol−1 of [EOE-MPyrr]NTf2/[Bu-MPyrr]NTf2, ●). This result can be related to the structure of the terminal group connected with the ether O atom. In comparison with the rod-like CH2CH3 tail on the EOE- chain, the O-terminal alkyl group on the MOE- chain is the spherical CH3, whose rotation is invalid for the chain flexibility. Thirdly, the EOM- moiety gives the largest ΔMVO value, even larger than the EOE- moiety, for example, 9.26 mL mol−1 of [EOM-MPyrr]NTf2/[Pr-MPyrr]NTf2 (■) vs. 8.04 mL mol−1 of [EOE-MPyrr]NTf2/[Bu-MPyrr]NTf2 (●). The largest ΔMVO value in EOM-substituted RTILs is perhaps due to the short spacer length of only one methylene between the ether O atom and the cationic N atom, and the side chain flexibility can promote the body's molecular motion through the short methylene spacer.
![The proposed alkoxy chain rotational motion for the ΔMVO values. (★: [MOE-MIm]NTf2/[Pr-MIm]NTf2, ◆: [MOE-MPyrr]NTf2/[Pr-MPyrr]NTf2, ▼: [EOE-MIm]NTf2/[Bu-MIm]NTf2, ▲: [EOE-Py]NTf2/[Bu-Py]NTf2, ●: [EOE-MPyrr]NTf2/[Bu-MPyrr]NTf2, ■: [EOM-MPyrr]NTf2/[Pr-MPyrr]NTf2.)](/image/article/2012/RA/c2ra21772d/c2ra21772d-f3.gif) |
| Fig. 3 The proposed alkoxy chain rotational motion for the ΔMVO values. (★: [MOE-MIm]NTf2/[Pr-MIm]NTf2, ◆: [MOE-MPyrr]NTf2/[Pr-MPyrr]NTf2, ▼: [EOE-MIm]NTf2/[Bu-MIm]NTf2, ▲: [EOE-Py]NTf2/[Bu-Py]NTf2, ●: [EOE-MPyrr]NTf2/[Bu-MPyrr]NTf2, ■: [EOM-MPyrr]NTf2/[Pr-MPyrr]NTf2.) | |
According to the above analysis, the rotational flexibility of the MOE- group is totally restricted in imidazolium RTILs, and no excess free volume should come into existence. Consequently, the MOE-substituted imidazolium RTILs possess the lowest ΔMVO values, i.e. 4.44 mL mol−1 of [MOE-MIm]BF4/[Pr-MIm]BF4 and 4.56 mL mol−1 of [MOE-MIm]NTf2/[Pr-MIm]NTf2. As a result, the average of the two ΔMVO values, (4.44 + 4.56)/2 = 4.50 mL mol−1, is approximately considered to be the inherent volume of the ether O unit in RTILs. Since the ΔMVO value consists of the inherent volume of the ether O unit and the total excess free volume, and the inherent volume of the ether O atom was supposed to be 4.50 mL mol−1, the total excess free volume derived from the ether O atom was calculated using eqn (2), and the resulting ΔFVO values are listed in Table 2.
| ΔFVO = ΔMVO − 4.50 mL mol−1 | (2) |
Actually, the total free volume of a liquid can be roughly calculated by surface tension method (eqn (3)),24f,g where ∑v is the total free volume (mL mol−1), N is the Avogadro constant (6.02 × 1023), k is the Boltzmann constant (1.38 × 10−23 J K−1), T is the absolute T/K, and γ is surface tension (N m−1).
| Σv = 2N × 0.6791(kT/γ)3/2 | (3) |
However, this method is not applicable as the side chain length increases.24b,33 In order to confirm the rationality of the above obtained ΔFVO values, the surface tension method was used to calculate the total free volume (∑v) of two pairs of the isomeric RTILs, and the corresponding results are listed in Table 3. The surface tension of these RTILs was observed between 34.47 and 38.06 mN m−1, which is close to the common NTf2-based imidazolium RTILs.34 Using the γ values, the ∑v values were calculated to be between 29.06 and 33.72 mL mol−1. Generally, the major materials exhibit a 10–15% free volume fraction.24f The free volume fraction of the studied RTILs falls within the expected range, for example, 32.25/295.18 = 10.9% for [EOM-MPyrr]NTf2. In comparison, the surface tension of the lower-viscosity MOE-substituted RTILs is generally smaller than their analogous EOM-substituted RTILs, and the total free volume of the former is larger than the latter. The difference of the total free volume between the isomeric RTILs is 32.25–29.06 = 3.19 mL mol−1 for [EOM-MPyrr]NTf2/[MOE-MPyrr]NTf2, and 33.72–30.27 = 3.45 mL mol−1 for [EOM-EDMAm]NTf2/[MOE-EDMAm]NTf2. According to the ΔMVO values, the corresponding two values were calculated to be 3.43 mL mol−1 (4.76–1.33) and 3.91 mL mol−1 (4.93–1.02), respectively. The approximation between 3.19 and 3.43 mL mol−1 or between 3.45 and 3.91 mL mol−1 defends the rationality of the ΔFVO values in Table 2.
Discussion
The changes in the free volume and viscosity as a result of the incorporation of an oxygen atom in an alkyl side chain of RTILs were measured and recorded as ΔFVO (mL mol−1) and ηO/η or ΔEη (kJ mol−1), respectively. According to Fig. 4 and 5, both ηO/η and ΔEη exhibit a nearly linear dependence on the ΔFVO values. This dependence is consistent with the hole theory, where viscosity involves an exchange of position between a hole and its adjacent molecule.24b,35 In detail, a larger ΔFVO value means the alkoxy chain can provide more available hole locations to promote mass transport and to decrease viscosity, i.e., smaller ηO/η (<1) or ΔEη (<0) values. It can be seen from Fig. 4 and 5 that both ηO/η and ΔEη generally decrease with the increase of ΔFVO values. The two observed trends clearly indicate that the increased free volume (ΔFVO) plays a decisive role in the viscosity change of the ether-substituted RTILs (ηO/η or ΔEη). According to the fitted linear trend in Fig. 4, when ηO/η = 1.00, where the alkoxy chain shows no effect on viscosity, the ΔFVO value was found to be 0.71 mL mol−1. Analogously, the corresponding ΔFVO value for ΔEη = 0.00 kJ mol−1 is 1.07 mL mol−1 as shown in Fig. 5. The two fitted values yield an average of 0.89 mL mol−1, which could be taken as the key point of the ΔFVO value for the alkoxy chain effect on viscosity. Generally, when ΔFVO > 0.89 mL mol−1, the addition of an ether O unit will decrease or increase viscosity. For ΔMVO, the corresponding key point is (4.50 + 0.89) = 5.39 mL mol−1.
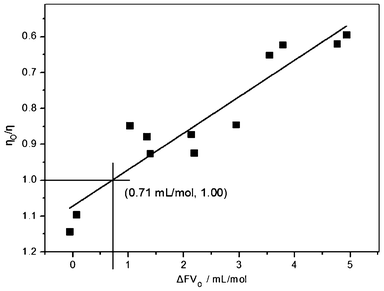 |
| Fig. 4 The changing relationship between ηO/η and ΔFVO. | |
 |
| Fig. 5 The changing relationship between ΔEη and ΔFVO. | |
Is it reasonable for the quantitative relationship between ΔFVO and ηO/η or ΔEη values shown in Fig. 4 and 5? An alternative method is to decrease the free volume and increase viscosity of the RTILs by an external pressure.25–27 For example, at 25 °C, when the pressure was raised from 0.1 to 24.3 MPa, [Bu-MIm]NTf2 would be compressed by 3.44 mL mol−1 from 291.90 to 288.46 mL mol−1, and its viscosity would be increased from 51.0 to 68.1 (0.749 times).25 According to the hole theory,24b,24f,35 3.44 mL mol−1 variation in free volume is responsible for the viscosity change of 0.749. Although this characterization cannot be conducted in our laboratory, the available literature data25–27 can be used for comparative study. For the isomeric RTILs, i.e., [MOE-MPyrr]NTf2vs. [EOM-MPyrr]NTf2 and [MOE-EDMAm]NTf2vs. [EOM-EDMAm]NTf2, there are great differences in viscosity and free volume between them. Generally, the EOM-substituted RTILs have larger free volume and lower viscosity than their isomeric MOE-RTILs, for example, [MOE-MPyrr]NTf2 (29.06 mL mol−1, 54.8 cP) vs. [EOM-MPyrr]NTf2 (32.25 mL mol−1, 38.7 cP), and [MOE-EDMAm]NTf2 (30.27 mL mol−1, 63.6 cP) vs. [EOM-EDMAm]NTf2 (33.72 mL mol−1, 44.6 cP). If [MOE-MPyrr]NTf2 and [EOM-MPyrr]NTf2 were taken as two states of a liquid under different pressure, 3.19 mL mol−1 (32.25–29.06) change in the total free volume should be responsible for the viscosity change of 0.706 (38.7/54.8), as well as 3.45 mL mol−1 free volume (291.76–287.85) for 0.701 (44.6/63.6) viscosity change in the pair of [MOE-EDMAm]NTf2 and [EOM-EDMAm]NTf2. These change relationships between free volume and viscosity in the isomers are close to the previously reported value 3.44 mL mol−1 free volume change for the viscosity change of 0.749 for [Bu-MIm]NTf2.25 As a result, the quantitative relationships between ΔFVO and ηO/η or ΔEη values shown in Fig. 4 and 5 were confirmed to be appropriate.
Actually, the ηO/η and ΔEη values could be correlated with the alkoxy chain rotational flexibility via the ΔFVO values. Generally, the highly flexible alkoxy chains trend to cause the less efficient packing between bulk ions19a and some excess free volume come into existence, resulting in decreasing viscosity. In order to maximize the alkoxy chain effect on decreasing the viscosity of RTILs, three factors need to be considered, i.e. hydrogen bond, the terminal alkyl group in the ether, and the spacer length between the ether O atom and the cationic nitrogen atom, analogous to ΔFVO (Fig. 3). First of all, the ηO/η and ΔEη values in imidazolium and pyridinium RTILs are larger than those in pyrrolidinium and ammonium RTILs, in agreement with the reported literature,20c–e,20j,20ne.g., [EOE-MIm]NTf2/[Bu-MIm]NTf2 (ηO/η = 0.872, ΔEη = −0.53 kJ mol−1) vs. [EOE-MPyrr]NTf2/[Bu-MPyrr]NTf2 (0.651, −2.80 kJ mol−1). The hydrogen bonds between the ether O atom and the aromatic ring hydrogen in imidazolium and pyridinium RTILs should be responsible for the low efficiency of the alkoxy chain effect on viscosity, via restraining the rotational motion of the alkoxy chains. Secondly, the spherical CH3 terminal group reduces the rotational flexibility of the MOE-ether chain, compared with its analogous EOE-ether, whose terminal group is the rod-like CH2CH3 and effective in increasing the chain flexibility via rotation. As a result, the ηO/η and ΔEη values obtained from MOE-substituted RTILs are larger than those from EOE-RTILs, e.g., [MOE-EDMA]NTf2/[Pr-EDMA]NTf2 (0.847, −1.57 kJ mol−1) vs. [EOE-EDMA]NTf2/[Bu-EDMA]NTf2 (0.622, −3.60 kJ mol−1). In addition, the EOE-substituted RTILs can be even less viscous than their MOE-substituted counterparts, e.g. at 25 °C, [EOE-MPyrr]NTf2 (51.8 cP) vs. [MOE-MPyrr]NTf2 (54.8 cP), consistent with the reported literature.20g,h It is argued that the EOE- group is longer but more flexible than the MOE- group, and flexibility dominates over length in reducing the viscosity of the ether derivatives. Thirdly, the short methylene spacer between the ether O atom and the cationic N atom in the EOM- group seems to be able to promote the structural molecular motion (flexibility), and can lead to the largest ΔFVO value and the lowest ηO/η and ΔEη values, in comparison with MOE- and EOE- groups, e.g., [EOM-MPyrr]NTf2/[Pr-MPyrr]NTf2 (0.620, −3.54 mL mol−1) vs. [EOE-MPyrr]NTf2/[Bu-MPyrr]NTf2 (0.651, −2.80 mL mol−1) and [MOE-MPyrr]NTf2/[Pr-MPyrr]NTf2 (0.878, −1.07 mL mol−1).
Consequently, it could be argued that the alkoxy chain effect on decreasing viscosity is strongly dependent on the amount of the increased total free volume, which results from the alkoxy chain rotational flexibility and can facilitate mass transport. According to the above results, in order to maximize the alkoxy chain effect on decreasing viscosity, both the ether and cation structures should be considered. First of all, the tail group should not be the spherical methyl; secondly, the spacer length between the cationic core and the ether O atom should be as short as only one methylene; thirdly, there should not be the factors that weaken the rotational flexibility, such as hydrogen bonds.
Low viscosity allows high conductivity for RTILs to be better applied as electrolytes in electrochemical devices. Another major factor on the ionic conductivity of RTILs is the ion sizes.36,37 The conductivity (κ) of the alkyl- and alkoxy-substituted pyrrolidinium and ammonium RTILs was determined, ranging from 1.86 to 4.97 mS cm−1 at 25 °C, as shown in Table 4. As can be summarized, the obtained conductivity values show a significant dependence on the side chains, and generally increase in an order of Bu- < EOE- < Pr- ≈ MOE- < EOM-. Although the incorporation of an ether O unit tends to increase the cation volume and decrease conductivity, the rotational flexibility of the alkoxy chains dominates over the increase in the cation volume, and leads to high conductivity of the ether derivatives, e.g., [Bu-EDMA]NTf2 (1.86 mS cm−1) vs. [EOE-EDMA]NTf2 (2.78 mS cm−1). For the isomeric RTILs, viscosity is the only decisive factor and should be reciprocally dependent on the conductivity, e.g., [EOM-MPyrr]NTf2 (4.97 mS cm−1, 38.7 cP) and [MOE-MPyrr]NTf2 (3.72 mS cm−1, 54.8 cP).
A wide electrochemical window is also required for RTILs to be used as electrolytes in electrochemical devices with high energy and power density, such as supercapacitor and battery systems.12,13,20f The electrochemical windows of the pyrrolidinium and ammonium RTILs were evaluated by cyclic voltammetry on a glassy-carbon electrode, as typically illustrated in Fig. 6. Table 4 summarizes the test results for the respective cathodic (Ecathodic) and anodic (Eanodic) limits, and electrochemical windows (EW = Eanodic − Ecathodic). Evidently, all of the measured RTILs possess wide electrochemical windows, ranging up to 5.68–5.83 V. Unlike some previous literature, where the alkoxy group sometimes can cause more than 0.5 V reduction in EW,20d,e the alkoxy group in this study shows no significant negative effect on the electrochemical windows, which are larger than 5.68 V. Both the wide electrochemical windows and high conductivity of the ether-functionalized RTILs indicate their significant promise for electrochemical applications.
Conclusion
An attempt to understand the reason for the low viscosity of the ether-functionalized RTILs was performed in an experimental method, based on a comparative study between 12 ether-substituted RTILs and their alkyl counterparts short of an ether O atom. The properties of the RTILs, including viscosity, the activation energy of viscosity, density, molar volume, surface tension, the total free volume, conductivity and electrochemical window, were investigated for this study. Replacing an alkyl chain by an alkoxy chain with an additional oxygen unit in RTILs shows a significant effect on viscosity (ηO/η) and its activation energy (ΔEη). Both the obtained ηO/η and ΔEη values for the 12 pairs of RTILs are highly variable and strongly dependent on the structures of the cation and ether moiety, and range 1.143–0.594 and 1.69–−4.14 kJ mol−1, respectively. For the first time, the ether groups were found to be able to increase the total free volume of RTILs, with ΔFVO ranging up to 4.93 mL mol−1. For the 12 pairs of RTILs, both the ηO/η and ΔEη values generally decrease with increasing ΔFVO values, indicating that the increased free volume plays a positive key role in decreasing viscosity. Consequently, the alkoxy chain effect on decreasing viscosity was ascribed to the increased free volume, which results from the high conformational flexibility of the ether moieties and provides more available holes for the convenience of mass transport and low viscosity. In these RTILs, the alkoxy chain flexibility is generally governed by three factors. In order to better exert the alkoxy chain effect on decreasing viscosity and increasing the free volume, the alkoxy groups were supposed to contain a rod-like alkyl tail and a –CH2– spacer between the ether O atom and the cationic N atom, and be in an environment without hydrogen bond donors. As a result of their low viscosity, the ether-substituted RTILs possess high conductivity up to 4.97 mS cm−1 at 25 °C, and meanwhile the alkoxy chains can maintain the high electrochemical stability of pyrrolidinium and ammonium RTILs with electrochemical windows wider than 5.68 V, indicating significant promise for electrochemical applications. The results in this work should be very helpful to design and synthesize novel functionalized liquid materials, in particular those with highly flexible side chains to form low viscosity solvents and high conductivity electrolytes.
Experimental section
Materials and characterization
All the chemicals and solvents were reagent grade quality and obtained commercially and used without further purification, except for CH3CH2OCH2Cl, which was redistilled under anhydrous conditions before use. 1H-NMR spectra (ppm, Δ) were recorded on a BRUKER NMR spectrometer (300 MHz), with CD3COCD3 as solution and TMS as an internal reference. FT-IR spectra (cm−1) were measured using diamond smart orbit ATR on a Nicolet 6700 FT- IR instrument.
Property testing
Prior to each test, the RTIL samples would be vacuum dried for 20 h at 80 °C and 10−2–10−3 mbar. The water content in RTILs could be reduced to be less than 150 ppm,20h after such a vacuum drying process. The density (g mL−1) of the RTILs was determined at 25 °C using a DMA 35 portable density meter, which was calibrated with ultrapure water (0.99704 g mL−1 at 25 °C). Each density value represents the average of three measurements, with standard deviation less than 0.0002 g mL−1. The viscosity (cP) was recorded with a BROOKFIELD DV-II+ Pro viscometer at nine temperatures ranging from 10 to 80 °C. The viscosity measurement was performed in a glove box with moisture concentrations below 1 ppm, and each value is based on the average of three measurements with standard deviation less than 2 cP. The surface tension (mN m−1) was measured by a Kibron EZ-Pi plus Tensiometer using the du Noüy technique at 25 °C, and averaged by 6 tests (±0.05 mN m−1). The conductivity (mS cm−1) was obtained by using a JENCO EC330 (B) conductivity meter, and averaged by three tests (±0.05 mS cm−1). The cyclic voltammetry testing was carried out on a CHI 660D electrochemical work station with a glassy carbon working electrode and an Ag/AgCl reference electrode at room temperature. The temperature for the measurements was maintained at ±0.1 °C by means of an external temperature controller.
General procedure for the synthesis of [Pr-MPyrr]Br, [Bu-MPyrr]Br, [MOE-MPyrr]Br, [EOE-MPyrr]Br, [Pr-EDMAm]Br, [Bu-EDMAm]Br, [MOE-EDMAm]Br, [EOE-EDMAm]Br, [Pr-MIm]Br, [Bu-MIm]Br, [MOE-MIm]Br, [Bu-Py]Br and [EOE-Py]Br
In a typical procedure, equimolar amounts (0.40 mol) of an amine and of an alkyl or alkoxyalkyl bromide were added into 80 mL anhydrous ethanol. After stirring at room temperature for 10 h and then at 50 °C for 48 h, the solvent was removed under vacuum at 80 °C, leaving a colourless or pale yellow solid. After twice recrystallization from a mixture of acetone and anhydrous diethyl ether, a white solid was obtained in a yield of about 80%.
General procedure for the synthesis of [EOM-MPyrr]Cl and [EOM-EDMAm]Cl
In a typical procedure, 0.40 mol CH3CH2OCH2Cl was added dropwise into a mixture of 60 mL anhydrous dichloromethane and an amine (0.40 mol equiv.) in a round bottom flask fitted with a drying tube at 0 °C. After stirring for 4 h at 30 °C, the solvent was evaporated under vacuum at 50 °C, affording a pale yellow solid. After twice recrystallization from the mixture of acetone and anhydrous diethyl ether, a white solid was obtained in a yield of about 80%.
Synthesis of [EOE-MIm]Br
A mixture of 0.48 mol CH3CH2OCH2CH2Br, 0.40 mol N-methylimidazole and 80 ml anhydrous ethanol was vigorously stirred for 4 d at 50 °C. After the reaction, the solvent was removed under vacuum at 80 °C, leaving a colourless liquid. After extraction with anhydrous diethyl ether (3 × 50 mL) and drying under vacuum, a colourless liquid was obtained in a yield of 88%.
General procedure for the synthesis of NTf2-based RTILs
In a typical procedure, an ammonium halide (0.10 mol) and lithium bis(trifluoromethylsulfonyl)imide (LiNTf2) (0.11 mol) were added into 40 mL ultrapure water and then stirred for 4 h at room temperature. After the reaction, the upper water phase was decanted off. The bottom IL phase was washed with 0.1 M aqueous LiNTf2 solution (20 mL) and ultrapure water (3 × 20 mL) in a separating funnel. Concentration under vacuum at 80 °C afforded a colorless or pale yellow liquid, with a yield of about 90%. By AgNO3 test and halide titration with 0.5M AgNO3 using 0.2 g of the ionic liquid, no halide content was detected (halide content < 200 ppm).38
General procedure for the synthesis of BF4-based RTILs
The anion exchange reaction was performed by using triethyloxonium tetrafluoroborate [Et3O]BF4, and in a glove box with moisture concentrations below 1 ppm. In a typical procedure, an ammonium halide (0.10 mol) and equimolar [Et3O]BF4 were added to 40 mL anhydrous dichloromethane in a round bottom flask. After stirring for 2 h at room temperature, the mixture was concentrated in vacuo at 80 °C to remove the solvent, yielding a colorless or pale yellow liquid. The halide content was evaluated by AgNO3 test to be less than 200 ppm.
[Pr-MPyrr]NTf2
1H-NMR (CD3COCD3, 300 MHz, ppm): 3.726 (m, 4H), 3.504 (m, 2H), 3.256 (s, 3H), 2.328 (m, 4H), 1.959 (m, 2H), 1.109 (t, 3H); IR (cm−1): 2980.64, 2947.26, 2888.93, 1472.23, 1347.14, 1328.94, 1225.78, 1173.45, 1131.65, 1049.81, 970.30, 938.77, 788.57, 761.91, 739.55, 653.80, 611.84, 599.63, 568.62, 509.44.
[Bu-MPyrr]NTf2
1H-NMR (CD3COCD3, 300 MHz, ppm): 3.727 (m, 4H), 3.548 (m, 2H), 3.263 (s, 3H), 2.328 (m, 4H), 1.916 (m, 2H), 1.440 (m, 2H), 0.984 (t, 3H); IR (cm−1): 2969.37, 2943.26, 2880.85, 1467.06, 1347.03, 1329.10, 1225.71, 1173.96, 1131.91, 1050.19, 964.88, 928.14, 788.42, 762.07, 739.38, 653.72, 612.32, 599.59, 568.69, 509.67.
[MOE-MPyrr]NTf2
1H-NMR (CD3COCD3, 300 MHz, ppm): 3.948 (m, 2H), 3.783 (m, 6H), 3.400 (s, 3H), 3.301 (s, 3H), 2.321 (m, 4H); IR (cm−1): 2991.65, 2942.42, 2902.29, 2825.53, 1475.20, 1463.33, 1347.29, 1328.80, 1226.08, 1174.44, 1130.49, 1050.15, 996.65, 933.40, 788.56, 762.25, 739.68, 653.79, 611.83, 599.70, 568.72, 509.17.
[EOE-MPyrr]NTf2
1H-NMR (CD3COCD3, 300 MHz, ppm): 3.990 (m, 2H), 3.792 (m, 6H), 3.584 (q, 2H), 3.315(s, 3H), 2.325 (m, 4H), 1.193 (t, 3H); IR (cm−1): 2981.90, 2938.53, 2885.34, 1474.61, 1462.78, 1347.60, 1329.11, 1226.01, 1175.07, 1130.54, 1050.11, 998.47, 935.29, 788.43, 762.14, 739.60, 653.71, 612.48, 599.68, 568.78, 509.39.
[EOM-MPyrr]NTf2
1H-NMR (CD3COCD3, 300 MHz, ppm): 4.866 (s, 2H), 3.960 (q, 2H), 3.769 (m, 2H), 3.628(m, 2H), 3.274 (s, 3H), 2.313 (m, 4H) 1.288 (t, 3H); IR (cm−1): 2986.90, 2940.15, 2903.54, 1464.10, 1391.98, 1347.52, 1329.13, 1225.88, 1174.23, 1131.03, 1050.34, 998.61, 927.07, 788.49, 762.24, 739.67, 653.86, 612.11, 599.61, 568.69, 509.36.
[Pr-EDMAm]NTf2
1H-NMR (CD3COCD3, 300 MHz, ppm): 3.607 (q, 2H), 3.454 (m, 2H), 3.262 (s, 6H), 1.934 (m, 2H), 1.466 (m, 2H), 1.019 (t, 3H); IR (cm−1): 3049.95, 2982.40, 2949.71, 2888.72, 1487.73, 1462.82, 1422.98, 1346.78, 1328.30, 1226.16, 1174.44, 1131.84, 1050.22, 976.34, 950.43, 788.85, 761.92, 739.85, 653.99, 612.36, 599.97, 568.86, 509.72.
[Bu-EDMAm]NTf2
1H-NMR (CD3COCD3, 300 MHz, ppm): 3.609 (q, 2H), 3.494 (m, 2H), 3.265 (s, 6H), 1.880 (m, 2H), 1.459 (m, 5H), 0.989 (t, 3H); IR (cm−1): 3050.48, 2969.54, 2942.50, 2881.87, 1487.84, 1464.15, 1422.78, 1346.67, 1328.46, 1225.99, 1174.87, 1132.02, 1050.43, 983.09, 955.74, 920.14, 788.71, 762.20, 739.70, 653.90, 612.58, 599.91, 568.88, 510.05.
[MOE-EDMAm]NTf2
1H-NMR (CD3COCD3, 300 MHz, ppm): 3.950 (m, 2H), 3.749 (t, 2H), 3.678 (q, 2H), 3.394 (s, 3H), 3.314 (s, 4H), 1.474 (m, 3H); IR (cm−1): 3048.85, 2997.09, 2941.45, 2894.82, 2831.37, 1484.11, 1473.31, 1458.08, 1346.77, 1328.26, 1226.22, 1175.01, 1131.95, 1050.42, 960.88, 918.31, 788.88, 762.42, 739.90, 653.93, 612.32, 599.99, 568.85, 510.01.
[EOE-EDMAm]NTf2
1H-NMR (CD3COCD3, 300 MHz, ppm): 3.983 (m, 2H), 3.736 (t, 2H), 3.678 (q, 2H), 3.576(q, 2H), 3.314 (s, 4H), 1.473 (m, 3H), 1.188 (t, 3H); IR (cm−1): 3048.77, 2983.02, 2936.81, 2882.65, 1486.63, 1470.48, 1347.19, 1328.64, 1226.13, 1175.81, 1131.92, 1050.46, 960.37, 914.72, 788.70, 762.25, 739.80, 653.97, 612.82, 600.08, 568.93, 510.41.
[EOM-EDMAm]NTf2
1H-NMR (CD3COCD3, 300 MHz, ppm): 4.860 (s, 2H), 3.977 (q, 2H), 3.585 (q, 2H), 3.213(s, 6H), 1.461 (m, 3H), 1.294 (t, 3H); IR (cm−1): 3047.79, 2989.48, 2939.74, 2906.19, 1473.93, 1452.94, 1347.31, 1328.63, 1226.16, 1175.55, 1132.12, 1050.46, 981.91, 862.68, 788.90, 762.40, 739.91, 654.16, 612.36, 600.02, 568.91, 510.22.
[Pr-MIm]NTf2
1H-NMR (CD3COCD3, 300 MHz, ppm): 9.022 (s, 1H), 7.768 (t, 1H), 7.721 (t, 1H), 4.336 (t, 2H), 4.072 (s, 3H), 1.976 (m, 2H), 0.966 (t, 3H); IR (cm−1): 3157.29, 3121.85, 2975.01, 2943.75, 2885.26, 1573.24, 1459.42, 1346.55, 1329.09, 1169.06, 1131.80, 1050.16, 843.23, 788.95, 739.40, 651.98, 611.10, 598.71, 568.50, 508.64.
[Bu-MIm]NTf2
1H-NMR (CD3COCD3, 300 MHz, ppm): 9.062 (s, 1H), 7.791 (t, 1H), 7.735 (t, 1H), 4.384 (t, 2H), 4.079 (s, 3H), 1.940 (m, 2H), 1.391 (m, 2H), 0.949 (t, 3H); IR (cm−1): 3156.43, 3121.11, 2966.71, 2940.25, 2879.33, 1573.07, 1466.69, 1346.72, 1329.19, 1176.16, 1131.91, 1050.45, 843.02, 788.82, 739.35, 652.32, 611.36, 598.76, 568.55, 508.97.
[MOE-MIm]NTf2
1H-NMR (CD3COCD3, 300 MHz, ppm): 9.001 (s, 1H), 7.734 (t, 1H), 7.701 (t, 1H), 4.536 (t, 2H), 4.080 (s, 3H), 3.815 (t, 2H), 3.353 (s, 3H); IR (cm−1): 3159.31, 3122.12, 2943.12, 2902.05, 2840.13, 1566.93, 1453.35, 1346.57, 1329.05, 1174.34, 1131.83, 1050.22, 835.08, 788.91, 761.92, 739.57, 652.38, 611.35, 598.53, 568.50, 508.10.
[EOE-MIm]NTf2
1H-NMR (CD3COCD3, 300 MHz, ppm): 9.013 (s, 1H), 7.746 (t, 1H), 7.702 (t, 1H), 4.532 (t, 2H), 4.083 (s, 3H), 3.854 (t, 2H), 3.532 (q, 2H), 1.136 (t, 3H); IR (cm−1): 3158.11, 3122.62, 2980.72, 2937.76, 2876.07, 1566.90, 1450.78, 1347.18, 1329.42, 1175.40, 1131.39, 1050.48, 831.58, 788.81, 761.84, 739.53, 652.27, 611.79, 598.68, 568.65, 508.05.
[Pr-MIm]BF4
1H-NMR (CD3COCD3, 300 MHz, ppm): 8.987 (s, 1H), 7.752 (t, 1H), 7.706 (t, 1H), 4.315 (t, 2H), 4.044 (s, 3H), 1.959 (m, 2H), 0.955 (t, 3H); IR (cm−1): 3161.46, 3121.73, 2971.68, 2942.52, 2883.47, 1574.29, 1460.75, 1431.64, 1389.97, 1347.71, 1338.78, 1285.31, 1171.08, 1044.91, 1030.62, 1016.40, 846.57, 754.93, 649.64, 622.65, 520.21.
[Bu-MIm]BF4
1H-NMR (CD3COCD3, 300 MHz, ppm): 8.987 (s, 1H), 7.754 (t, 1H), 7.699 (t, 1H), 4.349 (t, 2H), 4.038 (s, 3H), 1.921 (m, 2H), 1.383 (m, 2H), 0.944 (t, 3H); IR (cm−1): 3161.03, 3121.41, 2963.67, 2938.17, 2876.77, 1573.75, 1466.54, 1431.73, 1385.07, 1339.08, 1285.07, 1169.32, 1044.99, 1032.56, 1015.72, 847.41, 753.54, 651.04, 622.68, 520.26.
[MOE-MIm]BF4
1H-NMR (CD3COCD3, 300 MHz, ppm): 8.961 (s, 1H), 7.715 (t, 1H), 7.686 (t, 1H), 4.512 (t, 2H), 4.052 (s, 3H), 3.802 (t, 2H), 3.345 (s, 3H); IR (cm−1): 3161.94, 3122.15, 2942.41, 2902.30, 2837.07, 1574.94, 1567.15, 1453.93, 1431.16, 1385.29, 1357.10, 1339.88, 1285.89, 1224.93, 1171.10, 1113.52, 1045.10, 1028.26, 1011.45, 832.24, 753.26, 703.41, 652.61, 622.87, 520.20.
[EOE-MIm]BF4
1H-NMR (CD3COCD3, 300 MHz, ppm): 8.968 (s, 1H), 7.724 (t, 1H), 7.687 (t, 1H), 4.504 (t, 2H), 4.052 (s, 3H), 3.841 (t, 2H), 3.526 (q, 2H), 1.131 (t, 3H); IR (cm−1): 3162.14, 3122.12, 2977.05, 2934.68, 2874.18, 1574.74, 1567.24, 1451.46, 1431.39, 1382.25, 1352.27, 1339.80, 1285.13, 1216.36, 1171.51, 1114.05, 1044.59, 1028.32, 1014.87, 846.89, 754.12, 703.02, 652.43, 622.92, 520.20.
[Bu-Py]NTf2
1H-NMR (CD3COCD3, 300 MHz, ppm): 9.191 (d, 2H), 8.753 (t, 1H), 8.293 (t, 2H), 4.861 (t, 2H), 2.129 (m, 2H), 1.463 (m, 2H), 0.982 (t, 3H); IR (cm−1): 3138.32, 3094.50, 3073.79, 2969.06, 2940.56, 2880.26, 1636.24, 1503.33, 1489.34, 1468.75, 1346.49, 1329.60, 1226.10, 1174.22, 1030.85, 1050.15, 956.07, 787.48, 769.11, 739.20, 682.89, 653.93, 611.48, 598.99, 568.49, 508.96.
[EOE-Py]NTf2
1H-NMR (CD3COCD3, 300 MHz, ppm): 9.146 (d, 2H), 8.778 (t, 1H), 8.295 (t, 2H), 5.024 (t, 2H), 4.041 (t, 2H), 3.527 (q, 2H), 1.093 (t, 3H); IR (cm−1): 3140.77, 3095.29, 3072.50, 2981.96, 2936.37, 2877.27, 1636.82, 1501.86, 1489.82, 1448.59, 1347.04, 1329.61, 1226.20, 1174.73, 1030.21, 1050.34, 929.35, 788.23, 774.52, 739.70, 682.12, 653.92, 611.67, 599.25, 568.52, 507.94.
[Bu-Py]BF4
1H-NMR (CD3COCD3, 300 MHz, ppm): 9.163 (d, 2H), 8.731 (t, 1H), 8.263 (t, 2H), 4.832, (t, 2H), 2.102 (m, 2H), 1.451 (m, 2H), 0.972 (t, 3H); IR (cm−1): 3139.76, 3096.64, 3075.23, 2963.96, 2937.70, 2876.97, 1635.96, 1503.72, 1489.15, 1468.04, 1386.37, 1322.87, 1285.25, 1221.43, 1173.24, 1044.03, 1031.06, 1020.92, 770.83, 737.18, 683.09, 520.17.
[EOE-Py]BF4
1H-NMR (CD3COCD3, 300 MHz, ppm): 9.109 (d, 2H), 8.757 (t, 1H), 8.265 (t, 2H), 4.986 (t, 2H), 4.016 (t, 2H), 3.519 (q, 2H), 1.085 (t, 3H); IR (cm−1): 3141.10, 3097.34, 3074.15, 2978.47, 2934.43, 2874.72, 1636.41, 1501.47, 1489.15, 1448.95, 1382.19, 1352.67, 1285.29, 1219.28, 1178.93, 1043.56, 1019.83, 928.81, 824.06, 776.51, 682.45, 520.22.
Acknowledgements
This work is supported by Startup Grant of Nanyang Technological University and Academic Research Fund (RG21/09) of Ministry of Education in Singapore.
References
-
(a) J. P. Hallett and T. Welton, Chem. Rev., 2011, 111, 3508–3576 CrossRef CAS;
(b) R. D. Rogers and K. R. Seddon, Science, 2003, 302, 792–793 CrossRef.
-
(a) M. Armand, F. Endres, D. R. MacFarlane, H. Ohno and B. Scrosati, Nat. Mater., 2009, 8, 621–629 CrossRef CAS;
(b) B. A. Rosen, A. Salehi-Khojin, M. R. Thorson, W. Zhu, D. T. Whipple, P. J. A. Kenis and R. I. Masel, Science, 2011, 334, 643–644 CrossRef CAS.
-
(a) T. Torimoto, T. Tsuda, K. Okazaki and S. Kuwabata, Adv. Mater., 2010, 22, 1196–1221 CrossRef CAS;
(b) J. Le Bideau, L. Viau and A. Vioux, Chem. Soc. Rev., 2011, 40, 907–925 RSC.
- K. Fujita, K. Murata, M. Masuda, N. Nakamura and H. Ohno, RSC Adv., 2012, 2, 4018–4030 RSC.
- A. D. Sawant, D. G. Raut, N. B. Darvatkar and M. M. Salunkhe, Green Chem. Lett. Rev., 2011, 4, 41–54 CrossRef CAS.
- J. D. Scholten, B. C. Leal and J. Dupont, ACS Catal., 2012, 2, 184–200 CrossRef CAS.
- H. Tadesse and R. Luque, Energy Environ. Sci., 2011, 4, 3913–3929 CAS.
- D. Freudenmann, S. Wolf, M. Wolff and C. Feldmann, Angew. Chem., Int. Ed., 2011, 50, 11050–11060 CrossRef CAS.
- Z. H. Li, Z. Jia, Y. X. Luan and T. C. Mu, Curr. Opin. Solid State Mater. Sci., 2008, 12, 1–8 CrossRef CAS.
- M. D. Joshi and J. L. Anderson, RSC Adv., 2012, 2, 5470–5484 RSC.
- F. Zhou, Y. M. Liang and W. M. Liu, Chem. Soc. Rev., 2009, 38, 2590–2599 RSC.
- G. T. Kim, S. S. Jeong, M. Z. Xue, A. Balducci, M. Winter, S. Passerini, F. Alessandrini and G. B. Appetecchi, J. Power Sources, 2012, 199, 239–246 CrossRef CAS.
- J. Jeon, H. Kim, W. A. Goddard, T. A. Pascal, G. I. Lee and J. K. Kang, J. Phys. Chem. Lett., 2012, 3, 556–559 CrossRef CAS.
- L. Wei, M. Sevilla, A. B. Fuertes, R. Mokaya and G. Yushin, Adv. Funct. Mater., 2012, 22, 827–834 CrossRef CAS.
- A. J. Boydston, P. D. Vu, O. L. Dykhno, V. Chang, A. R. Wyatt, A. S. Stockett, E. T. Ritschdbrff, J. B. Shear and C. W. Bielawski, J. Am. Chem. Soc., 2008, 130, 3143–3156 CrossRef CAS.
- T. Peppel, M. Kockerling, M. Geppert-Rybczynska, R. V. Ralys, J. K. Lehmann, S. P. Verevkin and A. Heintz, Angew. Chem., Int. Ed., 2010, 49, 7116–7119 CrossRef CAS.
- Y. Fukaya, Y. Iizuka, K. Sekikawa and H. Ohno, Green Chem., 2007, 9, 1155–1157 RSC.
- O. J. Curnow, D. R. MacFarlane and K. J. Walst, Chem. Commun., 2011, 47, 10248–10250 RSC.
-
(a) L. J. A. Siqueira and M. C. C. Ribeiro, J. Phys. Chem. B, 2009, 113, 1074–1079 CrossRef CAS;
(b) C. Rey-Castro, A. L. Tormo and L. F. Vega, Fluid Phase Equilib., 2007, 256, 62–69 CrossRef CAS;
(c) S. Tsuzuki, H. Matsumoto, W. Shinoda and M. Mikami, Phys. Chem. Chem. Phys., 2011, 13, 5987–5993 RSC;
(d) H. Shirota and E. W. Castner, J. Phys. Chem. B, 2005, 109, 21576–21585 CrossRef CAS.
-
(a) S. K. Tang, G. A. Baker and H. Zhao, Chem. Soc. Rev., 2012, 41, 4030–4066 RSC;
(b) S. Y. Hong, J. Im, J. Palgunadi, S. D. Lee, J. S. Lee, H. S. Kim, M. Cheong and K. D. Jung, Energy Environ. Sci., 2011, 4, 1802–1806 RSC;
(c) Z. B. Zhou, H. Matsumoto and K. Tatsumi, Chem.–Eur. J., 2004, 10, 6581–6591 CrossRef CAS;
(d) Z. B. Zhou, H. Matsumoto and K. Tatsumi, Chem.–Eur. J., 2005, 11, 752–766 CrossRef CAS;
(e) Z. B. Zhou, H. Matsumoto and K. Tatsumi, Chem.–Eur. J., 2006, 12, 2196–2212 CrossRef CAS;
(f) T. Sato, G. Masuda and K. Takagi, Electrochim. Acta, 2004, 49, 3603–3611 CrossRef CAS;
(g) Z. B. Zhou, H. Matsumoto and K. Tatsumi, Chem. Lett., 2004, 33, 1636–1637 CrossRef CAS;
(h) Z. J. Chen, S. M. Liu, Z. P. Li, Q. H. Zhang and Y. Q. Deng, New J. Chem., 2011, 35, 1596–1606 RSC;
(i) H. Matsumoto, H. Sakaebe and K. Tatsumi, J. Power Sources, 2005, 146, 45–50 CrossRef CAS;
(j) S. H. Fang, L. Yang, J. X. Wang, M. T. Li, K. Tachibana and K. Kamijima, Electrochim. Acta, 2009, 54, 4269–4273 CrossRef CAS;
(k) S. H. Fang, Y. F. Tang, X. Y. Tai, L. Yang, K. Tachibana and K. Kamijima, J. Power Sources, 2011, 196, 1433–1441 CrossRef CAS;
(l) H. B. Han, J. Nie, K. Liu, W. K. Li, W. F. Feng, M. Armand, H. Matsumoto and Z. B. Zhou, Electrochim. Acta, 2010, 55, 1221–1226 CrossRef CAS;
(m) M. Chai, Y. D. Jin, S. H. Fang, L. Yang, S. Hirano and K. Tachibana, Electrochim. Acta, 2012, 66, 67–74 CrossRef CAS;
(n) N. Terasawa, S. Tsuzuki, T. Umecky, Y. Saito and H. Matsumoto, Chem. Commun., 2010, 46, 1730–1732 RSC.
-
(a) A. Nishimoto, M. Watanabe, Y. Ikeda and S. Kohjiya, Electrochim. Acta, 1998, 43, 1177–1184 CrossRef CAS;
(b) K. Yoshida, M. Nakamura, Y. Kazue, N. Tachikawa, S. Tsuzuki, S. Seki, K. Dokko and M. Watanabe, J. Am. Chem. Soc., 2011, 133, 13121–13129 CrossRef CAS;
(c) J. Gong, K. Sumathy and J. Liang, Renewable Energy, 2012, 39, 419–423 CrossRef CAS;
(d) M. Watanabe, T. Endo, A. Nishimoto, K. Miura and M. Yanagida, J. Power Sources, 1999, 81–82, 786–789 CrossRef CAS.
-
(a) Z. F. Fei, W. H. Ang, D. B. Zhao, R. Scopelliti, E. E. Zvereva, S. A. Katsyuba and P. J. Dyson, J. Phys. Chem. B, 2007, 111, 10095–10108 CrossRef CAS;
(b) J. D. Holbrey, A. E. Visser, S. K. Spear, W. M. Reichert, R. P. Swatloski, G. A. Broker and R. D. Rogers, Green Chem., 2003, 5, 129–135 RSC;
(c) V. Kempter and B. Kirchner, J. Mol. Struct., 2010, 972, 22–34 CrossRef CAS.
-
S. Lampman, Characterization and failure analysis of plastics, ASM International, 2003, pp. 1-45 Search PubMed.
-
(a) A. P. Abbott, D. Boothby, G. Capper, D. L. Davies and R. K. Rasheed, J. Am. Chem. Soc., 2004, 126, 9142–9147 CrossRef CAS;
(b) A. P. Abbott, ChemPhysChem, 2004, 5, 1242–1246 CrossRef CAS;
(c) A. P. Abbott, ChemPhysChem, 2005, 6, 2502–2505 CrossRef CAS;
(d) A. P. Abbott, R. C. Harris and K. S. Ryder, J. Phys. Chem. B, 2007, 111, 4910–4913 CrossRef CAS;
(e) A. R. Abbott, G. Capper and S. Gray, ChemPhysChem, 2006, 7, 803–806 CrossRef CAS;
(f) J. Z. Yang, X. M. Lu, J. S. Gui and W. G. Xu, Green Chem., 2004, 6, 541–543 RSC;
(g) Q. A. Wang, H. Wang, F. S. Guo, Y. Liu, D. W. Fang and S. L. Zang, Fluid Phase Equilib., 2010, 299, 300–303 CrossRef CAS;
(h) H. Zhao, Z. C. Liang and F. Li, J. Mol. Liq., 2009, 149, 55–59 CrossRef CAS;
(i) C. E. Woodward and K. R. Harris, Phys. Chem. Chem. Phys., 2010, 12, 1172–1176 RSC;
(j) J. A. P. Coutinho, P. J. Carvalho and N. M. C. Oliveira, RSC Adv., 2012, 2, 7322–7346 RSC;
(k) C. Larriba, Y. Yoshida and J. F. de la Mora, J. Phys. Chem. B, 2008, 112, 12401–12407 CrossRef CAS.
- K. R. Harris, M. Kanakubo and L. A. Woolf, J. Chem. Eng. Data, 2007, 52, 1080–1085 CrossRef CAS.
- K. R. Harris, M. Kanakubo and L. A. Woolf, J. Chem. Eng. Data, 2007, 52, 2425–2430 CrossRef CAS.
-
(a) K. R. Harris, M. Kanakubo and L. A. Woolf, J. Chem. Eng. Data, 2006, 51, 1161–1167 CrossRef CAS;
(b) K. R. Harris, L. A. Woolf and M. Kanakubo, J. Chem. Eng. Data, 2005, 50, 1777–1782 CrossRef CAS;
(c) S. Bulut, P. Eiden, W. Beichel, J. M. Slattery, T. F. Beyersdorff, T. J. S. Schubert and I. Krossing, ChemPhysChem, 2011, 12, 2296–2310 CrossRef CAS.
- N. Ramesh, P. K. Davis, J. M. Zielinski, R. P. Danner and J. L. Duda, J. Polym. Sci., Part B: Polym. Phys., 2011, 49, 1629–1644 CrossRef CAS.
-
(a) C. F. Ye and J. M. Shreeve, J. Phys. Chem. A, 2007, 111, 1456–1461 CrossRef CAS;
(b) H. D. B. Jenkins, H. K. Roobottom, J. Passmore and L. Glasser, Inorg. Chem., 1999, 38, 3609–3620 CrossRef CAS.
- D. W. Fang, W. Guan, J. Tong, Z. W. Wang and J. Z. Yang, J. Phys. Chem. B, 2008, 112, 7499–7505 CrossRef CAS.
- L. Glasser, Thermochim. Acta, 2004, 421, 87–93 CrossRef CAS.
-
(a) P. Song, A. H. Gao, P. W. Zhou and T. S. Chu, J. Phys. Chem. A, 2012, 116, 5392–5397 CrossRef CAS;
(b) F. A. S. Chipem, A. Mishra and G. Krishnamoorthy, Phys. Chem. Chem. Phys., 2012, 14, 8775–8790 RSC.
- M. H. Ghatee, M. Zare, A. R. Zolghadr and F. Moosavi, Fluid Phase Equilib., 2010, 291, 188–194 CrossRef CAS.
- M. Tariq, M. G. Freire, B. Saramago, J. A. P. Coutinho, J. N. C. Lopes and L. P. N. Rebelo, Chem. Soc. Rev., 2012, 41, 829–868 RSC.
- N. Hirai and H. Eyring, J. Appl. Phys., 1958, 29, 810–816 CrossRef CAS.
- P. Bonhote, A. P. Dias, N. Papageorgiou, K. Kalyanasundaram and M. Gratzel, Inorg. Chem., 1996, 35, 1168–1178 CrossRef CAS.
- D. D. Patel and J.-M. Lee, Chem. Rec., 2012, 12, 329–355 CrossRef CAS.
- D. Reinhardt, F. Ilgen, D. Kralisch, B. Konig and G. Kreisel, Green Chem., 2008, 10, 1170–1181 RSC.
|
This journal is © The Royal Society of Chemistry 2012 |