DOI:
10.1039/C2RA21583G
(Paper)
RSC Adv., 2012,
2, 12255-12262
Self photostabilizing UV-durable MWCNT/polymer nanocomposites†
Received
26th July 2012
, Accepted 5th October 2012
First published on 8th October 2012
Abstract
A potentially active hindered amine light stabilizer (HALS) was successfully anchored onto multiwalled carbon nanotubes (MWCNTs) and used as a light-stabilizing yet reinforcing multifunctional nanofiller to obtain UV-durable polymer nanocomposites. The influence of such light stabilizing MWCNTs on the photo-oxidation behaviour and structure-properties of polypropylene (PP) was studied. The composites were prepared by solution mixing of MWCNTs followed by melt compounding with polypropylene (PP). The resulting composite exhibits excellent UV-durability showing an almost 20 fold increase in the induction period of photo-oxidation. Moreover, the hydrophobic HALS was found to be compatibilizing enough to achieve homogeneous dispersion of exfoliated nanotubes into a polymer matrix. The rheological characterizations predict the formation of a percolated network structure. The obtained nanocomposites present markedly improved mechanical properties which underline the reinforcing ability of functionalized MWCNTs. Overall combination of HALS and MWCNTs offers an attractive route to combine multifunctionality into new hybrid UV-durable polymer nanocomposites. Such materials may possess great potential for outdoors high performance applications.
1 Introduction
The prospect of developing novel and new hybrid carbon-based materials and their polymer composites has excited immense attention among researchers. Today we stand at a similar threshold in the realm of polymer nanocomposites with the promise of strong, durable, multifunctional materials with low nanofiller content. However, the potential for new multifunctional materials lies in the versatility of both the polymer and the chemistry of the filler that can be exploited in the material synthesis. With emerging availability of nanometer featured materials such as carbon nanotubes, fibres, plates, and particles, in combination with a growing ability to characterize and manipulate systems at this scale, nano-engineered multifunctional materials could hold the key for future development and use of advanced super-performing composites for several advanced applications.1–5 Carbon nanotubes (CNTs) are one of the most promising candidates for the design of the novel high strength polymer composites. It is believed that the high aspect ratio, mechanical strength, and thermo-electric conductivity of CNTs make them an ideal reinforcing material for polymers and many other engineering applications.6–9 Amongst, polypropylene/CNT composites have been widely prepared and investigated for various applications because of their easy processability, and the resulting shaped plastic articles have a perfect surface appearance over polymer composites using conventional carbon or glass fibers.10–12 Hence, such composites represent an attractive material for fabricating light weight high strength materials for advanced applications. However, real time properties like durability under specific conditions i.e. outdoor performance have been strongly considered as an important prerequisite for such nanocomposite materials to find potential applications. It is well known that organic polymers often undergo degradation when exposed to UV-light in the presence of air, and a question arises whether the addition of nano fillers accelerates oxidative processes or shows any impediment in the time alteration. In MWCNT based polymer nanocomposites, few reports describe the effect of nanotubes toward the photodegradation of polymers and reveal that nanotubes can act as weak antioxidants.13–18 However, a realistic stabilization is still needed to achieve assured long term outdoor durability and to date no reports that shed light on detailed photo-stabilization studies on such hybrid materials exist.
In order to control the photo-oxidation processes, light stabilizers like hindered amine light stabilizers (HALS) are often added to the polymeric materials as light protecting additives to extend their service life.19–21 However, such organic stabilizers are known to be readily lost through migration and volatilization which effectively results in a serious problem since the physical loss of stabilizers accelerates the ageing of polymers more than thermal-oxidation or photo-oxidation.22 Consequently, high retention of the additives is needed to achieve long term stability. Hence, covalent functionalization of HALS onto the polymer backbone is often practiced to achieve longer outdoor performance.23 In a similar line we have proposed that the grafting of HALS moieties onto carbon nanotubes via chemical fixation and the mixing of such multifunctional nanotubes is expected to result in more durable polymer nanocomposites.
Moreover, the high strength polymer nanocomposites demand uniform dispersion of the nanofiller without agglomeration. The advantages of carbon nanotubes have not been fully realized due to difficulties in producing their polymer composites with well-dispersed CNTs.24–31 One of the best methods for achieving the homogeneous dispersion of nanotubes in a polymer matrix is their chemical functionalization. Moreover, functionalization with moieties that are structurally close to the matrix polymer can ensure good compatibility which limits any microscopic phase separation in the resulting nanocomposites.32 Such functional nanotubes exhibit improved dispersion in solvents and polymers. Furthermore, covalent functionalization of active molecules can provide the means to engineer the CNTs/polymer interface to optimize the properties of the composite.33–35 A similar approach has been employed here in order to render MWCNTs more active and compatible by functionalizing highly hydrophobic hindered amine light stabilizers (HALS).
Here we have exploited the covalent grafting approach in order to anchor the active HALS moieties onto MWCNTs (denoted as MWCNTs-f-HALS). The highly hydrophobic character of HALS has been employed as a compatibilizer and to direct the uniform nanotube dispersion into the polymer matrix. The grafting was confirmed by Fourier transform infrared and Raman spectroscopy supported by transmission electron microscopy (TEM). The MWCNTs dispersion into the polymer matrix was observed by TEM morphological analysis. The MWCNTs/PP nanocomposites were fabricated by solution mixing followed by a melt extrusion process. The photo-stabilizing efficacy of MWCNTs-f-HALS was assessed under accelerated UV weathering and monitored by FT-IR spectroscopy. Furthermore, mechanical and viscoelastic properties of the resulting nanohybrids were also studied.
2 Experimental
2.1 Materials
The MWCNTs used in this work were obtained from Nanocyl S.A. (Nanocyl™ NC 7000), a hindered amine 1,2,2,6,6-pentamethyl-4-piperidinol and thionyl chloride (SOCl2) were obtained from Aldrich and were used after further purification. Isotactic PP (average Mw 250
000, MFI-4.5 g/10 min) was obtained from ExxonMobil.
2.2 Chemical oxidation of MWCNTs
Typically, 1.0 g of crude MWCNTs were added to 150 mL of HNO3
:
H2SO4 (1
:
3, v/v) and sonicated for 4 h in an ultrasonic bath (40 kHz); the resulting mixture was then transferred into a 500 mL flask equipped with a condenser and was refluxed with vigorous stirring at 90 °C for 9 h. After cooling to room temperature the mixture was subjected to vacuum filtration using a 0.2 μm Millipore membrane filter that was then washed several times with distilled water until the pH of the filtrate was 7.0. The filtered solid was vacuum dried for 24 h at 60 °C to give MWCNTs functionalized with carboxylic acid (MWCNTs–COOH).
MWCNTs–COOH (200 mg), synthesized as described above, was reacted with 100 mL of SOCl2 at 70 °C for 24 h under reflux to convert the surface-bound carboxylic acid groups into acyl chloride groups. Any residual SOCl2 was removed by rotary evaporation, and the solids that were subsequently obtained were filtered and washed with anhydrous THF. Lastly, the filtrate was dried under vacuum at room temperature for 4 h to give acyl chloride functionalized MWCNTs (MWCNTs–COCl).
2.4 Synthesis of MWCNTs functionalized HALS
MWCNTs functionalized HALS were obtained by reacting MWCNTs–COCl with hindered amines under reflux in THF solvent at 60 °C for 48 h under a nitrogen atmosphere. The resulting product (MWCNTs-f-HALS, Scheme 1) was then separated by centrifugation, washed well with methanol, and dried under vacuum at room temperature.
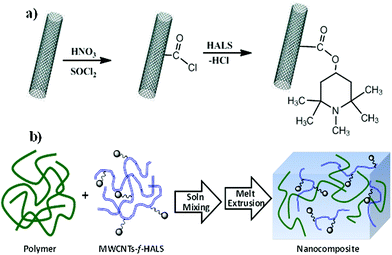 |
| Scheme 1 Synthetic routes used for the preparation of (a) MWCNTs-f-HALS and (b) MWCNTs-f-HALS/PP nanocomposite. | |
2.5 Preparation of the MWCNTs-f-HALS/PP nanohybrids
In order to achieve a better dispersion of functionalized MWCNTs, first ultrasonic treatment in xylene was carried out and later mixed with the designated amount of PP pre-dissolved in xylene. This process also aids the removal of any additives from the commercial polymer. The polymer solution was vigorously stirred for 1 h to obtain a homogeneous dispersion and later co-precipitated in cold methanol. The obtained composites were filtered and dried under vacuum overnight. In the next step, the composites were further subjected to melt extrusion using a DSM microcompounder (5 cc) at 180 °C, 100 rpm for 4 min. The extruded samples were compression molded using a hot press (Carver Inc.) at 170 °C, forming 100 μm films, which were rapidly quenched in a water bath at ambient temperature.
Composites with final concentrations of MWCNTs-f-HALS of 0, 1, 3, 5 wt% were obtained and designated as PP, PP-HCNT1, PP-HCNT3 and PP-HCNT5, respectively. For comparison, PP/MWCNT nanocomposites with 3 wt% pristine MWCNT and PP/HALS with a HALS concentration corresponding to PP-HCNT3 (based on TGA analysis) were prepared and labeled as PP-CNT3 and PP-HAS3, respectively.
2.6 UV-irradiation
UV-irradiations of nanocomposite films were carried out in air in a SEPAP 12/24 unit (ATLAS) at λ > 300 nm and 60 °C. This apparatus is equipped with four medium pressure mercury lamps with a borosilicate envelope which filters wavelengths below 300 nm and it is designed for the study of accelerated artificial photodegradation, in conditions that are relevant to natural outdoor weathering.36
2.7 Characterization techniques
Fourier transform infrared (FT-IR) spectra used to unveil HALS grafting onto the MWNTs and to monitor the photo-oxidation behavior in PP nanocomposites films were recorded using a Perkin-Elmer Spectrum GX infrared spectrometer. Spectra were obtained using 32 scans and a 4 cm−1 resolution. PP photo-oxidation was followed by the 1713 cm−1 intensity band which was plotted as a function of time, in order to avoid differences due to film thickness absorption was normalized to the IR absorption band at 2723 cm−1 (νC–H vibration stretching band of PP). The photo-oxidation was followed using the area of the carbonyl band (area between 1850–1600 cm−1 maximum at 1715 cm−1) normalized by the area of the rocking vibration bands of CH2 (area between 969 and 757 cm−1).
The Raman spectra were obtained by using a Renishaw Raman system Model 3000 spectrometer equipped with an integral microscope (Olympus BH2-UMA). Radiation from a He–Ne laser (633 nm) was used as the excitation source. Raman scattering was detected with 180° geometry by using a Peltier-cooled (−70 °C) charge-coupled device camera (400 × 600 pixels).
Transmission electron microscopy (TEM) images were obtained using a TEM 2000 EX-II microscope (JEOL, Tokyo, Japan) operated at a 100 kV accelerating voltage to observe the nanoscale structures of the functionalized MWCNTs. The specimen for the TEM observations was prepared by placing one drop of the sample on a copper grid coated with carbon. For polymer nanocomposites, ultrathin sections were cut at ambient temperature with a Leica Reichert FCS microtome and collected on a 300 mesh copper grid. The micrographs were taken using an energy filter in zero loss mode for an optimal contrast of the nanotubes.
The thermal behaviors of the MWCNTs were studied by using a TA instruments Q500 TGA at a heating rate of 10 °C min−1 under inert atmosphere.
The tensile properties of the films were determined with an Instron mechanical tester (Model 5564) at a crosshead speed of 5 mm min−1. The specimens were prepared by cutting strips with dimensions of 5 × 70 mm2. The reported data are the averages of at least 5 individual determinations.
Rheological properties were assessed using a Rheometric Scientific ARES rheometer and a parallel plate geometry of 25 mm diameter was used. The specimen thickness was about 0.5 mm. The storage modulus (G′) and complex viscosity (η*) were measured in frequency sweep experiments performed at 180 °C over the frequency range 0.1–100 rad s−1. The strain value was fixed at 5% ensuring that the measurements were carried out in the linear region.
3 Results and discussion
The changes in surface functionalities were depicted from FTIR spectroscopy and presented in Fig. 1. A distinct difference between the oxidized and functionalized MWCNTs can be observed. The HNO3–H2SO4 treatment produced carboxyl groups on the surface of MWCNTs because of oxidation, as indicated by the presence of characteristic peaks at 3435 and 1711 cm−1 for stretching vibrations of O–H and C
O of the carboxyl group, respectively (Fig. 1a). Functionalization of HALS resulted in the formation of ester linkages on the MWCNTs due to characteristic peak at 1730 cm−1 (Fig. 1b). Moreover, the vibrational bands at 2930–2850 and 1115 cm−1 which correspond to the characteristic C–H stretching of alkyl groups and C–N bond in the piperidine ring further confirm the covalent attachment between HALS and MWCNTs.
3.2 XPS study
The HALS bound MWCNTs have also been confirmed further by XPS (Fig. 2). A new peak is observed at 400 eV corresponding to the N1s also the peak of O1s (533 eV) for MWCNTs-f-HALS became significantly weaker in the XPS survey scan spectrum as compared with that of MWCNTs–COOH (Fig. S1, ESI†). In contrast, the C1s (285 eV) peak became much stronger. The narrow scan C1s XPS spectrum (inset Fig. 2) of MWCNTs-f-HALS shows characteristic signals at ca. 284.9 and 285.4, which accounts for C–C and C–O functionalities. Moreover, there are two additional absorbance peaks that appear at 286.3 and 287.5 eV corresponding to the carbon in the C–N and C
O bonds, respectively.37 These results suggest that the MWCNTs have been functionalized well by HALS through ester linkages. The % elemental composition of MWCNTs before and after functionalization has been tabulated in the supporting information (Table S1†).
3.3 Raman spectroscopy
The covalent functionalization was also demonstrated by Raman scattering spectroscopy. Fig. 3 shows the Raman spectra of MWCNTs before and after the modifications. Compared with the pristine MWCNTs, the D bands of the MWNTs–COOH and MWCNTs-f-HALS have significantly increased, indicating the damage to the side walls of nanotubes or the formation of fragments caused by the functionalization. Furthermore, the G band has shifted by ca. 10 cm−1 from 1563 cm−1 to 1579 and 1574 cm−1. This is a typical feature of chemical charge-transfer and provides direct evidence of the changes occurring in the graphite structures of the MWCNTs due to functionalization.38,39
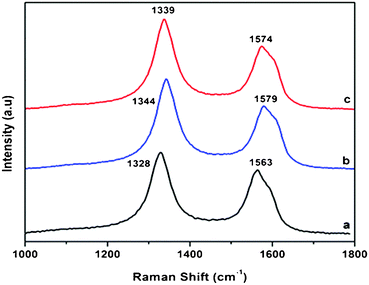 |
| Fig. 3 Raman spectra of a) pristine MWCNTs, b) MWCNT–COOH, c) MWCNTs-f-HALS. | |
3.4 Thermogravimetric analysis
The amount of HALS attached to the MWCNTs and the comparison of mass losses between pristine and functionalized nanotubes upon heating is determined by TGA as shown in Fig. 4. Pristine nanotubes show a single step decomposition at 637 °C and completely oxidize around 700 °C (Fig. S2†). Upon functionalization, a gradual mass loss starting from 150 °C, with a major loss at around 645 °C (Fig. 4). The first decomposition observed at 259 °C was attributed to the HALS wrapped around the nanotube and second attributed to the nanotube oxidation. The degree of functionalization from the gradual mass loss of the MWCNTs-f-HALS was estimated to be around 16.7 mass percent. As a result, this decomposition behavior confirms the existence of organo-inorganic hybrids.
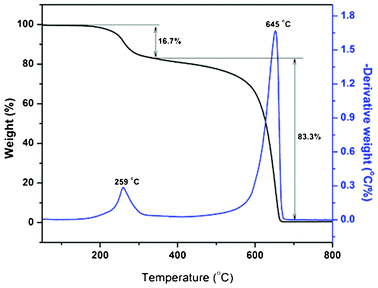 |
| Fig. 4 TGA/DTA curves of MWCNTs-f-HALS. | |
3.5 TEM analysis
More evidence on the HALS grafting has been collected from the morphologies by TEM images as shown in Fig. 5. In the TEM images of the pristine MWCNTs (Fig. 5a), the individual tubes were obviously separated from each other. The nanotube wall is relatively smooth and clean and does not appear covered with any extra phase. In contrast, TEM images of the MWCNTs-f-HALS (Fig. 5b) after many washings with dry THF under sonication, appear stained with HALS and they are glued together at the intersection points. This indicates that the covalent grafting reaction takes place not only at the tips but also on the whole MWNT surfaces. Moreover, post functionalization, the carbon nanotubes become more hydrophobic and stable dispersions in THF can be obtained after 24 h as shown in the inset images of Fig. 5.
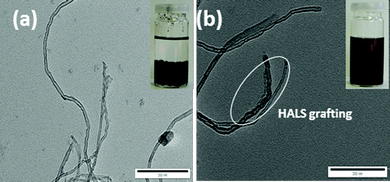 |
| Fig. 5 TEM micrographs of a) pristine MWCNTs and b) HALS grafted MWCNTs (inset images – dispersion in THF after 24 h), scale-200 nm. | |
3.6 Nanocomposite morphology
It is of interest to investigate the degree of dispersion of functionalized MWCNTs within MWCNTs/PP nanocomposites as examined by TEM. The nanotube content in the MWCNTs/PP nanocomposites was kept at 3 wt% in each sample. As shown in Fig. 6a, the pristine MWCNTs are not well dispersed in the matrix. One part of the nanotubes is dispersed individually while the other part is dispersed as agglomerates of different sizes. By comparison, the MWCNTs-f-HALS nanocomposites possess better homogeneity and relatively good dispersion in the matrix as shown in Fig. 6b, conceivably owing to the high compatibility of functionalized MWCNTs toward the polymer matrix. The functionalized nanotubes were embedded in the PP matrix and interconnected to networks, rather than being pulled out. This suggests that MWCNTs-f-HALS are held tightly to the matrix, demonstrating a strong interaction between the PP matrix and functionalized nanotubes.
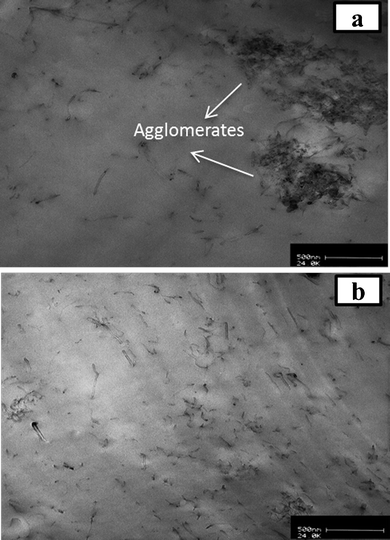 |
| Fig. 6 TEM micrographs of PP/MWCNTs-f-HALS nanocomposites a) PP-CNT3 and b) PP-HCNT3 (scale-500 nm). | |
3.7 Photostabilization of nanocomposites
In order to assess the photostabilizing efficacy of HALS grafted carbon nanotubes, the composites containing MWCNTs were subjected to accelerated photo-ageing (Fig. 7b). The photo-oxidation behavior of PP/MWCNT hybrids was monitored by FT-IR spectra. The photo-oxidation mechanism of isotactic PP and its nanocomposites have been reported numerous times in the literature.40–42 The main product of the combined action of UV-radiation and oxygen is the formation of the tertiary hydroperoxide. The oxidation continues up to the production of carboxylic acids, esters, peresters lactones and other oxidized species. The infrared analysis of PP photo-oxidation shows the formation of a band with a maximum at 1713 cm−1 (Fig. 7a). These modifications indicate the formation of a carbonylic band from the oxidated species. In the hydroxyl region, a broad absorption band is observed with a maximum at 3400 cm−1 that corresponds to products such as hydroperoxides and alcohols. In the case of the samples bands, the relative intensities of each carbonyl and hydroxyl band are the same as in the case of pure polypropylene. This indicates that the PP/MWCNT nanocomposites and PP develop similar photoproducts. Thus in this case the nanotube presence does not change the photo-oxidation mechanism.43
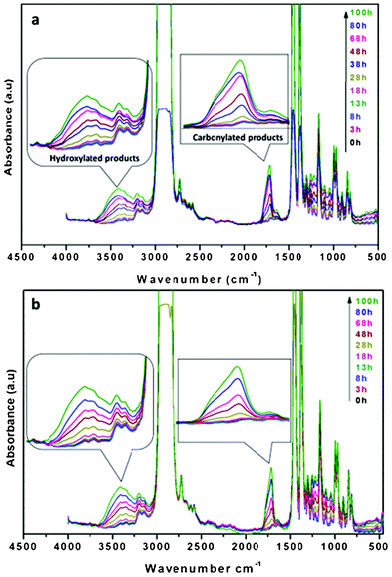 |
| Fig. 7 FTIR spectra of a) pure PP and b) PP-HCNT3 nanocomposite films photo-oxidized at λ > 300 nm, 60 °C. | |
The rates of photo-oxidation were determined from the kinetics of photo-oxidation and displayed in Fig. 8. The kinetic curves presented here were determined by plotting the variation of absorbance in the carbonyl region at 1713 cm−1 (Fig. 8a) and hydroxyl region at 3400 cm−1 (Fig. 8b), characteristic bands of the acids and alcohols coming from the oxidation of PP, respectively. The most relevant analysis on this set of curves is based on the comparison between the times necessary before the oxidation starts i.e.induction time. During this induction period where no oxidation of the polymer is detected, it is generally admitted that the additive plays its stabilizing role.44 The comparison of the oxidation rates in the different samples described above shows that the presence of the light stabilizer has a significant effect on the induction time, whereas the length of this induction period is dramatically reduced in the presence of unmodified MWCNTs.
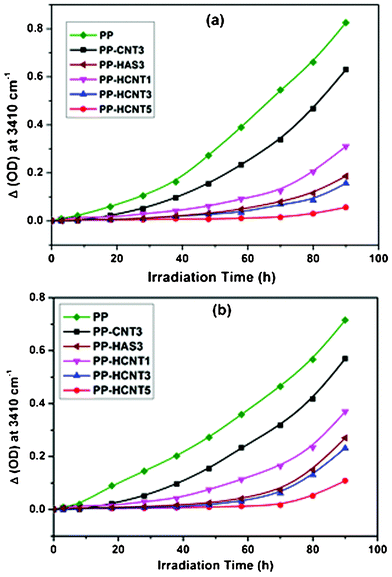 |
| Fig. 8 Evolution of absorbance at a) 1713 cm−1 and b) 3400 cm−1 as a function of irradiation time for PP and PP/MWCNT nanocomposite films photo-oxidized at λ > 300 nm, 60 °C. | |
In the cases of PP and PP/CNT3, the oxidation starts after approximately 3 h and 18 h, respectively. A 67 h shift in the carbonyl domain was observed for PP-HCNT5 with an induction time around 70 h. A similar trend has been observed in case of hydroxyl photoproducts. These findings underline that HALS grafted nanotubes add a significant photostabilizing effect to the polymer. This is because hindered amines are known to form stable nitroxyl radicals which later can trap the polymer free radicals generated during photolysis and not allowing the further photo-oxidation reactions. Moreover, further evolution of peroxide radicals can also be arrested via the cyclic stabilizing mechanism of HALS as shown in Scheme 2. Also, the specimen stabilized with HALS (PP-HAS3) without nanotubes shows a trivial increase in photo-oxidation compared to PP-HCNT3. This observation underlines the fact that MWCNTs in conjunction with HALS add a synergistic effect towards polymer photostabilization. Hence, such a dramatic influence of the MWCNT-f-HALS on the induction period suggests an increase in stabilizing activity offering considerable photo-oxidative durability to the resulting PP/MWCNT nanocomposites.
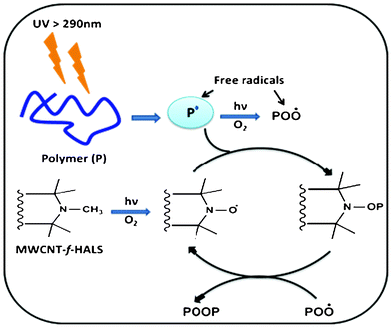 |
| Scheme 2 Photostabilizing action of HALS. | |
3.8 Rheological and mechanical characterization
The reinforcing ability of functionalized MWCNTs in PP was confirmed by studying rheo-mechanical properties. Understanding the rheological properties of the nanocomposites is of prime importance from the perspective of processability and structure–property relationships. Moreover, it provides an idea of the global state of dispersion in the composites, information about the percolated network structure, and the interaction between the filler and polymer matrix.45 Due to the nanometric size and the high aspect ratio of the nanoparticles, the specific surface area is high and thus responsible for strong interparticle interactions which make the dispersion in the matrix difficult. In the case of PP/MWCNT nanocomposites the weak nanofiller/matrix interaction and the entanglement of very long nanotubes increase the difficulty to obtain a well-defined network structure. So as to evaluate the influence of functionalized MWCNTs on the network structure of the elaborated nanocomposites, rheological measurements have been done. Fig. 9 represents the typical features of the linear viscoelastic response and the frequency dependence of the storage modulus (G′) and complex viscosity (η*) for the PP and MWNT/PP nanocomposites melt containing varying contents of MWCNTs-f-HALS measured at 180 °C. It is apparent that the storage moduli (Fig. 8a) of the nanocomposites increases with the increasing MWNTs loading, due to the reinforcing effect of the nanotubes. Specifically in the case of PP and PP-HCNT1, at low frequencies PP chains are fully relaxed and exhibit typical homopolymer like terminal behaviour. However, with increasing MWCNTs loading, the dependence of G′ on ω in the low frequency region weakens gradually, as the relaxations of the polymer chains in PP/MWCNT nanocomposites are effectively restrained by MWCNTs. This behaviour can be attributed to the formation of a network structure, which hinders the motion of polymer chains on the chain length scale.46 When the loading of MWCNTs is 3 wt% or higher, a great enhancement of G′ is observed in the low frequency region. At high frequencies, the relaxations of the polymer chains on the segment scale, such as an entanglement strand, are not significantly influenced by the existence of the network structure, so the effect of the MWCNTs on the rheological responses is relatively weak. To show the effect of surface characteristics of MWCNTs, the dynamic storage moduli of the two groups of nanocomposites at 0.01 Hz are compared. It can be found that G′ of PP-HCNT3 is higher than that of composites having pristine MWCNTs at 3 wt% loading (PP-CNT3). This phenomenon can be attributed to the presence of the highly hydrophobic groups of HALS, that is to say, the functionalized MWCNTs are more compatible with the polymer, resulting in stronger interfacial interactions between the MWCNTs and PP matrix (Fig. 9a).
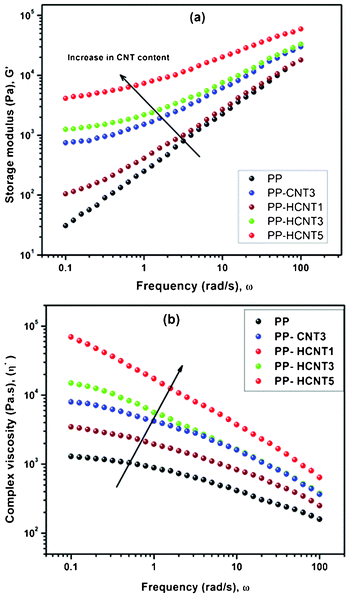 |
| Fig. 9 Rheological behavior a) storage modulus (G′) versus frequency (ω) and b) complex viscosity (η*) versus frequency (ω) of PP/MWCNT nanocomposites. | |
In Fig. 9b the dependence of complex viscosity (η*) on frequency for the PP/MWCNT nanocomposites is presented. It can be seen that with the frequency increasing, the complex viscosity decreased. The addition of the MWCNTs causes a dramatic enhancement of the shear viscosity, especially at low shear rate. It can be found that η* of PP-HCNT3 (PP/MWCNTs-f-HALS) is much higher than that of PP-CNT3 (PP/MWCNTs). It is believed that this difference in the contribution of the two kinds of MWCNTs to the rheological properties is mainly caused by the different surface characteristics of MWCNTs, or more exactly, the different interactions between PP and the MWCNTs. This observation again underlines the possible interactions between the polymer and functionalized MWCNTs which are considerably weaker for pristine MWCNTs.
The mechanical properties of MWCNTs-f-HALS reinforced PP nanocomposites were investigated through typical stress–strain curves as shown in Fig. 10. It is observed that the PP nanocomposites exhibit higher mechanical performance. It can be seen that the tensile strength of neat PP is 28.5 MPa which was gradually increased with increasing functionalized MWCNTs. This confirms that partial tensile strain can be transferred to the MWCNTs embedded in the PP matrix under tensile stress. The sample containing pristine nanotubes (PP-CNT3) experienced a slight decrease in tensile strength as compared to functionalized nanotubes (PP-HCNT3) and such behaviour might be attributed to poor dispersion of the pristine MWCNTs. The pronounced reinforcing effect of the functionalized MWCNTs is due to a good dispersion within polymer matrix which is also evidenced by the morphological analysis.
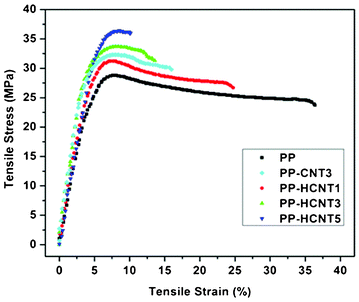 |
| Fig. 10 Stress–strain curves for pure PP and PP/MWCNT nanocomposites. | |
Conclusion
In this study, novel light stabilizer functionalized MWCNTs have been prepared and successfully employed as a multifunctional filler to obtain more UV-durable PP nanocomposites. The microscopic and spectroscopic characterization evidences the covalent attachment of HALS on the nanotube surface. The TEM micrographs show that the functionalized MWCNTs possess a better dispersion in the PP matrix in comparison to pristine MWCNTs. Accelerated UV-aging studies confirmed that the MWCNTs-f-HALS offer a better photostabilization effect to the resulting composites. Moreover, modification of the MWCNTs with grafted hydrophobic hindered amines was found to be a useful method for producing reinforced and durable MWCNTs/PP nanocomposites. Rheological characterization shows that the dynamic moduli and viscosity were increased and a fluid to solid transition takes place with the addition of functionalized MWNTs into PP resulting in a percolated network structure in the material. The mechanical strength was improved effectively by enhancing the nanotube dispersion to form MWCNT–MWCNT networks or by modulating the interface with the polymer matrix, due to higher polymer compatibility and a good chain interaction with the bulk polymer. The overall results show that the MWCNTs-f-HALS/PP nanocomposites fabricated in this work may perform remarkably in outdoor applications.
Acknowledgements
Dr S. P. Lonkar is thankful to the Director, IPF and Prof. Heinrich for providing the guest researcher grant and necessary research and characterization facilities.
References
- E. P. Giannelis, Adv. Mater., 1996, 8, 29–35 CrossRef CAS.
- D. Cai and M. Song, J. Mater. Chem., 2010, 20, 7906–7915 RSC.
- A. D. Pomogailo, Russ. Chem. Rev., 2000, 69, 53–80 CrossRef CAS.
- H. Althues, J. Henle and S. Kaskel, Chem. Soc. Rev., 2007, 36, 1454–1465 RSC.
- Y. Lin, M. J. Meziani and Y-P. Sun, J. Mater. Chem., 2007, 17, 1143–1148 RSC.
- M. Naffakh, M. Remškar, C. Marco, M. A. Gómez-Fatou and I. Jiménez, J. Mater. Chem., 2011, 21, 3574–3578 RSC.
- N. G. Sahoo, S. Rana, J. W. Cho, L. Li and S. H. Chan, Prog. Polym. Sci., 2010, 35, 837–867 CrossRef CAS.
- F. Beguin and P. Ehrburger, Carbon, 2002, 40, 1619 CrossRef.
- S. Subramoney, Adv. Mater., 1998, 10, 1157–1173 CrossRef CAS.
- M. Moniruzzaman and K. I. Winey, Macromolecules, 2006, 39, 5194–5205 CrossRef CAS.
- A. A. Kovalchuk, A. N. Shchegolikhin, V. G. Shevchenko, P. M. Nedorezova, A. N. Klyamkina and A. M. Aladyshev, Macromolecules, 2008, 41, 3149–3156 CrossRef CAS.
- M. C. Hsiao, S. H. Liao, Y.-F. Lin, C.-C. Weng, H. M. Tsai, C.-C. M. Ma, S.-H. Lee, M.-Y. Yen and P.-I. Liu, Energy Environ. Sci., 2011, 4, 543–550 CAS.
- P. C. P. Watts, P. K. Fearon, W. K. Hsu, N. C. Billingham, H. W. Kroto and D. R. M. Walton, J. Mater. Chem., 2003, 13, 491–495 RSC.
- L. Guadagno, C. Naddeo, M. Raimondo, G. Gorrasi and V. Vittoria, Polym. Degrad. Stab., 2010, 95, 1614–1626 CrossRef CAS.
- X. Shi, B. Jiang, J. Wang and Y. Yang, Carbon, 2012, 50, 1005–1013 CrossRef CAS.
- S. Morlat-Therias, E. Fanton, J.-L. Gardette, S. Peeterbroek, M. Alexandre and Ph. Dubois, Polym. Degrad. Stab., 2007, 92, 1873–1882 CrossRef CAS.
- S. Bocchini, A.-D. Blasio and A. Frache, Macromol. Symp., 2011, 301, 16–22 CrossRef CAS.
- Y. Lian, H. M. Wang and D. W. Huang, Adv. Mater. Res., 2011, 189–193 Search PubMed.
- F. Gugumus, Polym. Degrad. Stab., 1989, 24, 289–301 CrossRef CAS.
- K. Schwetlick and W. D. Habicher, Polym. Degrad. Stab., 2002, 78, 35–40 CrossRef CAS.
- M. Kaci, G. Hebal, N. Touati, A. Rabouhi, L. Zaidi and H. Djidjelli, Macromol. Mater. Eng., 2004, 289, 681–687 CrossRef CAS.
- M. Scoponi, S. Cimmino and M. Kaci, Polymer, 2000, 41, 7969–7980 CrossRef CAS.
- M. Auer, R. Nicolas, A. Vesterinen, H. Luttikhedde and C.-E. Wilén, J. Polym. Sci., Part A: Polym. Chem., 2004, 42, 1350–1355 CrossRef CAS.
- J. Gao, M. E. Itkis, A. Yu, E. Bekyarova, B. Zhao and R. C. Haddon, J. Am. Chem. Soc., 2005, 127, 3847–3854 CrossRef CAS.
- Y. Wang, J. Wu and F. Wei, Carbon, 2003, 41, 2939–2948 CrossRef CAS.
- C. Gao, C. D. Vo, Y. Z. Jin, W. Li and S. P. Armes, Macromolecules, 2005, 38, 8634–8648 CrossRef CAS.
- H. Kong, C. Gao and D. Yan, J. Am. Chem. Soc., 2004, 126, 412–413 CrossRef CAS.
- Y. P. Sun, W. Huang, Y. Lin, A. Kitaygorodskiy, L. A. Riddle, Y. J. Yu and D. L. Carroll, Chem. Mater., 2001, 13, 2864–2869 CrossRef CAS.
- Y. Liu, Z. Yao and A. Adronov, Macromolecules, 2005, 38, 1172–1179 CrossRef CAS.
- G. Guo, D. Yang, C. Wang and S. Yang, Macromolecules, 2006, 39, 9035–9040 CrossRef CAS.
- K. Mylvaganam and L. C. Zhang, J. Phys. Chem. B, 2004, 108, 15009–15026 CrossRef CAS.
- Y. Lin, M. J. Meziani and Y.-P. Sun, J. Mater. Chem., 2007, 17, 1143–1148 RSC.
- A. Star, Y. Liu, K. Grant, L. Ridvan, J. F. Stoddart, D. W. Steuerman, M. R. Diehl, A. Boukai and J. R. Heath, Macromolecules, 2003, 36, 553–560 CrossRef CAS.
- R. J. Chen, Y. Zhang, D. Wang and H. Dai, J. Am. Chem. Soc., 2001, 123, 3838–3839 CrossRef CAS.
- D. Bonduel, M. Mainil, M. Alexandre, F. Monteverde and Ph. Dubois, Chem. Commun., 2005, 781–783 RSC.
- L. Philippart, C. Sinturel and J.-L. Gardette, Polym. Degrad. Stab., 1997, 58, 261–268 CrossRef.
- D. S. Everhart and C. N. Reilley, Anal. Chem., 1981, 53, 665–676 CrossRef CAS.
- M. Holzinger, J. Abraham, P. Whelan, R. Graupner, L. Ley, F. Hennrich, M. Kappes and A. Hirsch, J. Am. Chem. Soc., 2003, 125, 8566–8580 CrossRef CAS.
- M. F. Islam, E. Rojas, D. M. Bergey, A. T. Johnson and A. G. Yodh, Nano Lett., 2003, 3, 269–273 CrossRef CAS.
- J. Lacoste, D. Vaillant and J. Carlsson, J. Polym. Sci., Part A: Polym. Chem., 1993, 31, 715–722 CrossRef CAS.
- J. L. Philippart, F. Posada and J. L. Gardette, Polym. Degrad. Stab., 1995, 49, 285–290 CrossRef CAS.
- S. P. Lonkar, S. Therias, N. Caperaa, F. Leroux and J.-L. Gardette, Eur. Polym. J., 2010, 46, 1456–1464 CrossRef CAS.
- S. Bocchini, A. D. Blasio and A. Frache, Macromol. Symp., 2011, 30, 16–22 CrossRef.
- C. Sinturel, J. L. Philippart, J. Lemaire and J.-L. Gardette, Eur. Polym. J., 1999, 35, 1773–1790 CrossRef CAS.
- M. K. Seo and S. J. Park, Chem. Phys. Lett., 2004, 395, 44–48 CrossRef CAS.
- F. M. Du, R. C. Scogna, W. Zhou, S. Brand, J. E. Fischer and K. I. Winey, Macromolecules, 2004, 37, 9048–9055 CrossRef CAS.
Footnotes |
† Electronic supplementary information (ESI) available: XPS scan spectra of pristine MWCNTs, TGA/DTA curves for MWCNTs–COOH and XPS elemental composition data. See DOI: 10.1039/c2ra21583g |
‡ Present address: Laboratory of Polymers and Composite Materials, University of Mons, 7000 Mons, Belgium. Tel: +32(0)65553374; Fax: +32(0)65373484 |
|
This journal is © The Royal Society of Chemistry 2012 |
Click here to see how this site uses Cookies. View our privacy policy here.