DOI:
10.1039/C2RA20811C
(Paper)
RSC Adv., 2012,
2, 8128-8134
Veruclay – a new type of photo-adsorbent active in the visible light range: modification of montmorillonite surface with organic surfactant
Received
6th March 2012
, Accepted 11th July 2012
First published on 12th July 2012
Abstract
Montmorillonite K10 was treated with VeruSOL-3, a biodegradable and food-grade surfactant mixture of coconut oil, castor oil and citrus extracts, to manufacture a benign catalytic adsorbent that is active in the visible light. Veruclay was characterized by SEM, XRD, TGA, UVDRS, and XPS analysis. The catalytic adsorption of cationic dye methylene blue (MB) and halogenated aromatic dichlorophenol (DCP) in the dark and visible light was assessed through the bench scale experiments. It was found that in the presence of visible light, MB degradation was nearly 100% in 45 min, whereas 75% of DCP was removed in 4 h. Demineralization of the solution in terms of TOC degradation of 18, 59, and 74% was achieved with 0.5, 1, and 2 g L−1 of Veruclay, respectively with DCP as a contaminant. Bacterial (Vibrio fischeri) bioluminescence inhibition assay demonstrated the effectiveness of Veruclay to significantly inhibit the microbial growth even at relatively low (50 mg L−1) Veruclay concentrations. It exhibited excellent stability for up to 7 consecutive runs and regained most of its catalytic activity after intermediate calcination.
1 Introduction
Recently, surfactant-intercalated clays have attracted great interest owing to their wide applications in the rheological controlling of paints and greases, in the degradation and sorption of toxic compounds, in the reinforcements in polymer matrix composites and plastics, and as precursors in the synthesis of various nanocomposites.1–4 Such intercalated clays called “organo-clays,” are hybrids formed by the exchange reaction between organic cations (e.g. various surfactants) and the interlayer cations in clay minerals.5
VeruSOL-3 is a benign compound, which combines biodegradable and food-grade surfactant mixtures, such as coconut oil, castor oil, and citrus extracts. It can be effectively utilized as a surfactant to alter surface properties. Also, it can be used to improve the sorption capacity of organic contaminants onto the montmorillonite K10 surface.
Thousands of tones of different dyes, pigments, and halogenated aromatic compounds are produced ever year worldwide, from which approx. 20% are discharged without treatment thus posing a significant threat to the environment or causing aesthetical irritation.1,6 Currently, there are numerous treatment techniques available including biological treatment, coagulation, floatation, oxidation, and nanofiltration; however, adsorption, especially using low cost adsorbents, remains the most effective approach, producing no harmful substances.7
Here we report on the preparation of Veruclay – an effective photocatalytic-adsorbent, which is active in the visible light range. As-prepared Veruclay was characterized by scanning electron microscopy,8 X-ray photoelectron spectroscopy (XPS), X-ray diffraction (XRD), thermogravimetric analysis (TGA), and UV diffuse reflectance spectroscopy (UV DRS). Antibacterial properties of as-prepared, sensitized Veruclay and recycled sensitized Veruclay were evaluated against V. fischeri. The adsorption and photodegradation of the cationic dye methylene blue (MB) and dichlorophenol (DCP) in the presence of Veruclay was found, studied, and discussed. Information presented in this manuscript will be useful in the complete understanding of the photo-adsorption process of organic molecules by the surfactant-modified montmorillonite from aqueous solutions in the presence of visible light.
2 Materials and methods
2.1 Materials
All the chemicals were used without further purification. Montmorillonite K10 (main chemical composition of SiO2 (69%), Al2O3 (14.6%), Fe2O3 (2.9%), MgO (2%) and CaO (1.5%), cation exchange capacity (CEC) of 30 meq/100 g−1 and surface area of 220–270 m2 g−1), methylene blue (MB, analytical grade) and dichlorophenol (DCP) were obtained from Sigma Aldrich (USA). VeruSOL-3 was provided by VeruTEK Technologies Inc. (Bloomfield, CT, USA). Double distilled water was used in all the experiments.
2.2 Preparation of Veruclay
During a typical synthesis, montmorillonite K10 (10 g), dried at 80 °C overnight to remove moisture, was slurried with 1.5 mL of VeruSOL-3. The resulting slurry was magnetically stirred for 12 h at room temperature. Then Veruclay was dried at 60 °C for 12 h. After drying, samples were powdered and stored in closed vessels prior to treatment. To recycle Veruclay, the spent material was subjected to 300 °C for 2 h in air and reused for the subsequent experiments.
2.3 Characterization of Veruclay
X-ray diffraction (XRD) patterns were recorded between 3 and 80° (2θ) at a scanning rate of 1/2° min−1 using an accelerating voltage of 45 kV and current of 40 mA on a X’Pert Pro MPD X-ray diffractometer with a Cu-Kα source. The phases were identified by using Joint Committee on Powder Diffraction Standards (JCPDS).
The morphology of samples was determined using scanning electron microscopy (FEI XL 30 ESEM), operating at 5–15 kV with EDS (energy dispersive X-ray spectroscopy).
X-ray photoelectron spectra (XPS) were recorded on a VG Multilab 2000 spectrometer (Thermo Electron Corporation) with Al Kα radiation as the exciting source (300 W). Binding energies were calibrated versus the carbon signal at 284.64 eV. Prior to the measurements, samples were distributed onto the copper adhesive tape to achieve uniform and complete coverage. All spectra were recorded at a 90° takeoff angle. All binding energies were referenced to the silicon 2s core level of montmorillonite clay at 102.9 eV9 and the resolution was 1.4 eV. The nominal X-ray beam diameter was 600 μm. Since all the samples were non-conductive, the charge binding energy by ∼4–5 eV was adopted. The typical error bar associated to the binding energies was ±0.3 eV.
The UV DRS spectra were recorded on a Shimadzu UV-250IPC instrument in the range 300 to 800 nm. The thermogravimetric analysis (TGA) was performed with a TGA Q5000 (TA instruments, USA) analyzer with a heating range of 10° min−1 in an airflow adopting a ramp method with an increase in temperature from 100 to 1000 °C. Changes in TOC were recorded on a Shimadzu TOC V CPH for Non-Purgeable Organic Carbon analyzer.
2.4 Adsorption equilibrium and degradation
To evaluate the adsorption of methylene blue (MB) and dichlorophenol (DCP), 100–300 mg L−1 of MB and DCP were prepared in separate glass beakers by dissolving the appropriate amounts in double distilled water to reach the required concentrations. The batch adsorption experiments were carried out at room temperature (23 ± 2 °C) in 100 mL closed glass containers in the dark, unless specified otherwise, where 0.05 g of Veruclay (total concentration of 1 g L−1) and 50 mL of MB or DCP were added. At regular 30 min intervals, 5 mL of the aliquot was withdrawn from the suspension and filtered through a 0.45 μm syringe filter to remove Veruclay particles. The concentrations of MB and DCP were determined by a UV spectrophotometer (Hewlett Packard 845X) at characteristic wavelengths.
After the adsorption equilibrium was reached, the supernatant layer of suspension was collected and centrifuged at 300 rpm for 15 min and then analyzed spectrophotometrically to determine the concentration of MB and DCP that remained in the solution.10
The amounts of MB and DCP adsorbed onto Veruclay were calculated from the concentration differences and the amounts of MB and DCP sorbed at equilibrium (Qe MB and Qe DCP, respectively):
| 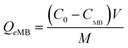 | (1) |
| 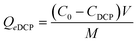 | (2) |
Where Qe MB and Qe DCP were the equilibrium adsorption capacities (mg g−1) C0 and CMB (CDCP) were initial and equilibrium MB and DCP concentrations (mg L−1), V was the volume of the solution, and M was the amount of dry Veruclay used as adsorbent (g).
To further assess the effectiveness of catalytic adsorbent, the typical photo-activity towards DCP was evaluated at solution temperatures of 25 °C, 35 °C, and 45 °C by placing 20 mL of DCP aqueous solution and 0.075 mg (concentration 1 g L−1) of Veruclay into a 25 mL glass reactor equipped with a magnetic stirrer. The photoreactor was irradiated using a 300 W medium pressure metal halogen desk lamp. DCP degradation was monitored by UV spectrophotometry.
2.5 Vibrio fischeri bioluminescence inhibition test
Inhibition of the bacterial growth was evaluated using Microtox toxicity kits (EBPI, Canada) according to the ISO standard.11 It is generally accepted that light production is directly proportional to the metabolic activity of the bacterial population and any inhibition of enzymatic activity causes a corresponding decrease in bioluminescence.12 The detailed testing procedure is described by Lappalainen and co-workers.13 In brief, freeze-dried photobacteria, V fischeri (NRRL B-11177), were rehydrated and stabilized at 4 °C for 1 h and then stabilized at 15 °C for 30 min prior to the measurements. Suspensions containing various concentrations of Veruclay (1, 5, 10, 25, 50, 100, 500, and 1000 mg L−1) were subjected to 30 min ultrasonication and then subsequently stabilized at 15 °C prior to the toxicity measurements.14
According to Alba and coworkers, color and turbidity reduces the light output recorded by the luminometer similar to toxicity,15 thus to avoid the discrepancies, the bacteria was separated by centrifuging from the sample matrix prior to the final luminescence measurements. Thus, 1 mL of centrifuged suspension was transferred to the vial and 200 μL of the bacterial solution was dispensed into the sample. To evaluate the inhibition of the bacterial growth in the presence of as prepared, sensitized, and recycled sensitized Veruclay, the light output was recorded at t0 and t30. All the samples were assayed in duplicates. The control test was carried out by recording the light output of the unstressed bacteria after otherwise identical experimental conditions. The light inhibition percentage (INH%) was calculated according to the standard and kinetic methods:
|  | (3) |
where KF isthe correction factor, LIC
t and LIC
0 are the luminescence intensity of the control after (
t30) and before (
t0) exposition (min), in relative units of luminescence.
16
|
| (4) |
where LICT
t and LICT
o were luminescence intensity of the tested sample after (t
30) and before (t
o) exposition (min), in relative units of luminescence.
3 Results and discussion
3.1 Characterization of Veruclay
3.1.1 SEM analysis.
The morphologies of K10 and Veruclay are shown in Fig. 1. It can be seen that pristine K10 (Fig. 1a) has relatively small particles and a rather non porous surface, which was also observed by Zhou et al.17 However, when the VeruSOL surfactant was introduced, the particles became larger and more agglomerated and a porous surface was formed (Fig. 1b). This indicated the surfactant in fact was incorporated into the K10 structure and formed cavities and/or voids that subsequently allowed MB and DCP into the galleries of the Veruclay.7
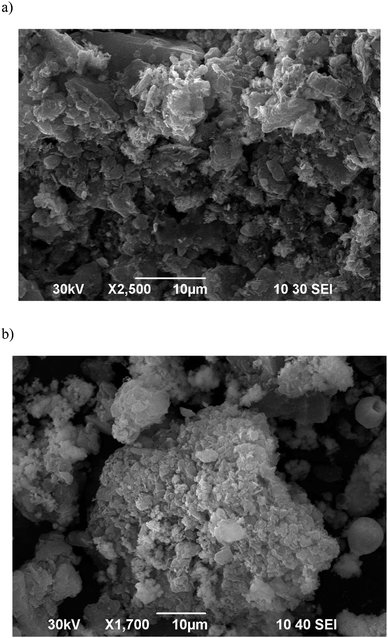 |
| Fig. 1 SEM images of a) pristine montmorillonite K10 and b) Veruclay. | |
3.1.2 XRD analysis.
Fig. 2 depicts X-ray diffraction spectrographs of pristine K10, Veruclay, Veruclay sensitized with MB, and Veruclay sensitized with MB and treated with DCP. Basal spacing values were calculated from the diffraction 2θ values of the peaks in the patterns. Further, the basal spacing (d001) of montmorillonite K10 increased with surfactant loading from ca. 14.2 Å to 18.2 Å indicating that clay treatment with VeruSOL-3 resulted in the structural changes (Fig. 2). Thus, after treatments, the basal plane spacing of pristine K10 was shifted to lower 2θ values, indicating intercalation of VeruSOL surfactant into the K10 gallery. Mishra et al., who researched the surface-modification of montmorillonite with various surfactants, observed similar results.18
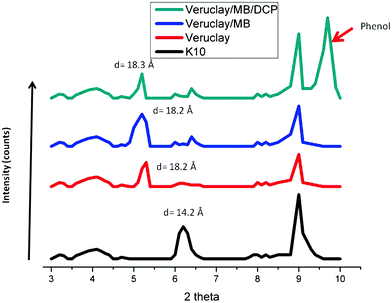 |
| Fig. 2 XRD analysis of pristine montmorillonite K10, Veruclay, Veruclay with intercalated MB and Veruclay with MB and DCP. | |
It was assumed that the relatively small shift in the 2θ values could be attributed to the monolayer type of arrangement for VeruSOL; therefore, a large amount of the surfactant was associated with the external surface of K10 as later confirmed by XPS measurements. The basal spacing of Veruclay/MB was 18.3 Å, which most likely indicated the formation of J-aggregate in the interlayer space.19 The d001 value was slightly larger as well as the diffraction peak observed at 2θ = 9.7° after the visible light treatment of dye-sensitized Veruclay (Fig. 2), indicating the small intercalation of DCP and the possible formation of degradation by-products.
3.1.3 TGA analysis.
Thermogravimetric analysis curves for pristine K10 and Veruclay are presented in Fig. 3. The curves for both, K10 and Veruclay showed about 3 to 5% of weight loss up to 250 °C. This initial loss in weight could be attributed to the loss of adsorbed water.20 In case of Veruclay, there was ca. 8% weight loss between 250 and 400 °C, which could be due to the decomposition of VeruSOL on the surface of K10. Then the last weight loss of ca. 11% and 3% for Verusol and K10, respectively was observed at ca. 400-750 °C due to dehydroxylation21 and/or structural water in the K10 layers as well as the residue of VeruSOL intercalated in K10 galleries.22
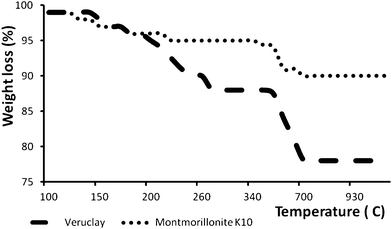 |
| Fig. 3 Thermogravimetric analysis curves of pristine montmorillonite K10 and Veruclay. | |
3.1.4 UV DRS analysis.
UV diffuse reflectance spectra of as-prepared and MB-sensitized Veruclay are shown in Fig. 4. Montmorillonite K10 did not exhibit any visible light activity (data not shown) because the direct band gap of montmorillonite is 5.35 eV23 therefore was not included into the figure and the discussion. Veruclay showed adsorption edges at about 390–410 nm, with the highest absorption peak at 390 nm, which was similar to observations made by Virkutyte and Varma,24 who researched the visible light activity of metal- intercalated montmorillonite. However, when Veruclay was sensitized with MB, a significant absorption in the visible light region up to 600 nm was witnessed, with the highest adsorption peak at 405 nm, indicating the activation of Veruclay via intercalation of cationic azo dye into the K10 galleries.25 The band gaps energy were estimated for the absorption edges using generally acceptable equation: E = 1240/λ26 and were 3.1 eV and 2.2 eV for Veruclay and MB sensitized Veruclay, respectively. Compared with the spectrum of Veruclay, the peaks in MB-sensitized Veruclay were shifted by 15-25 nm to longer wavelengths. The reason for such bathochromic shift may be associated with the adsorption and/or intercalation of MB into the montmorillonite. In the solution, MB molecules were rapidly adsorbed onto the montmorillonite surface via ion exchange and aggregation on the external surfaces of the tactoids.27,28 Then MB molecules migrated to the inter-lamellar region, resulting in the de-aggregation of the dimers and restoring the monomer.29 Therefore, according to Kasha’s molecular excitation theory, these bathochromic shifts could be ascribable to J-aggregates of the intercalated MB that formed an interdigitated monolayer to give a J-aggregate in the interlayer space.25
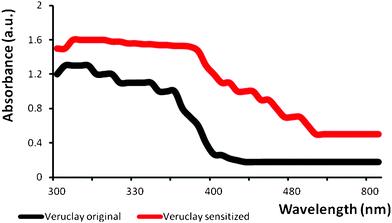 |
| Fig. 4 UV DRS spectra of Veruclay and MB-sensitized Veruclay. | |
3.1.5 XPS analysis.
Fig. 5 shows a low resolution general scan of Veruclay. Importantly, the absolute charge shift was around 2.2 eV for Veruclay. There were two types of material observed: parental montmorillonite that accounted for O (1s), Al (2p), P (2p), and Si (2p) and hydrocarbon (VeruSOL) with three main carbon components. The O (1s), C (1s) and Si (2p) binding energies of Veruclay were 531.8, 284.2 and 102.9, respectively, which closely corresponded to the binding energies of 531.4, 285.0 and 102.2 eV discussed in literature.24,30 According to the binding energies, P, Si and Al formed P–O, Al–O and (Al/P)–Si–O bonds. Furthermore, the binding energy of hydrocarbon was attributed to C–H and C–C type bonding, which can be found in aromatic compounds (e.g. limonene, the main constituent of VeruSOL). Thus, the XPS findings strongly supported the XRD observations that were discussed earlier.
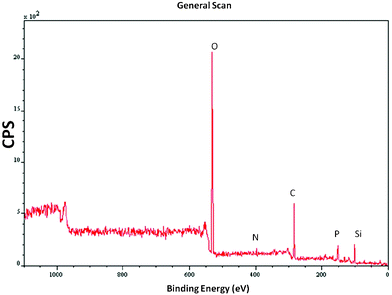 |
| Fig. 5 Low resolution scan of Veruclay. | |
3.2 MB and DCP adsorption onto Veruclay
The hydration of inorganic cations on the exchange sites cause the clay mineral surfaces to be hydrophilic; however, these surfaces are ineffective in the sorption of organic compounds.31 To overcome such a drawback, the montmorillonite surface can be modified with surfactants that convert the hydrophilic silicate surface to an organophilic one.32 To evaluate the effectiveness of Veruclay to remove selected organic contaminants (MB and DCP), a set of experiments was performed to assess the adsorption of MB and DCP on Veruclay in the dark. Fig. 6 shows adsorption isotherms onto Veruclay at room temperature (23 ± 2 °C). As could be seen, Veruclay exhibited a type I (IUPAC classification) isotherm, which indicated the monolayer adsorption of both, MB and DCP onto the Veruclay surface.10,33 In addition, such an isotherm signified high affinity between the clay surface and a contaminant and showed chemisorption.34
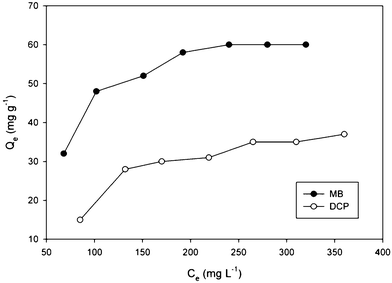 |
| Fig. 6 Adsorption isotherm onto Veruclay. | |
The equilibrium capacity of MB and DCP increased slowly from 32 to 60 and from 15 to 35 mg g−1 for MB and DCP, respectively, with an increase in the equilibrium concentration of both, MB and DCP (Fig. 6), which was consistent with results obtained by Gereli et al.34 According to Ma et al.,27 MB adsorption onto montmorillonite can be divided into i) rapid adsorption on the external surface of the clay by ion-exchange and aggregation and ii) deaggregation of the dimer and the monomer migrating to the interlamellar position of the clay. Consequently, the rate of adsorption decreased with time (data not shown). In addition, higher MB adsorption onto the Veruclay in comparison to that of DCP may be attributed to increased electrostatic attraction and an ion exchange mechanism.35
3.3 MB and DCP degradation in the visible light
Obviously, Veruclay can be used as an adsorbent to partially remove organic contaminants from solutions; however, simultaneous adsorption and degradation of pollutants in the visible light range would offer a significant benefit of using these surfactant-modified clays for environmental remediation. Thus, MB was subjected to the treatment with montmorillonite K10 and Veruclay in the presence of visible light.
According to Fig. 7, a complete removal of MB is attained after 90 and 45 min, respectively indicating a positive effect of the visible light irradiation. The reaction rate constants were 0.05 min−1 and 0.14 min−1 for montmorillonite and Veruclay, respectively, indicating that the intercalation of the surfactant nearly 3 fold increased the photocatalytic adsorption of MB. For comparison, Zhao and colleagues reported the degradation of Rhodamine B on rectorite in the visible light range with the reaction rate of 0.0413 min−1,36 which was significantly lower than the disappearance of MB on Veruclay indicating the superior activity of the as prepared photocatalytic adsorbent. Furthermore, the effectiveness of Veruclay was similar to the one observed by Zhao and colleagues in the direct solar light (0.122 min−1).36
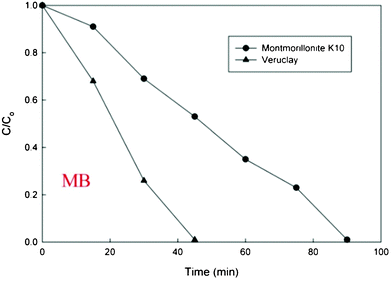 |
| Fig. 7 MB disappearance on the visible light. | |
Unfortunately, as can be seen from Fig. 8, DCP degradation at room temperature was not that effective with a reaction constant of 1.88 × 10−3 min−1. Thus, to test the effect of temperature on DCP removal, it was subjected to the treatment at 45 and 65 °C (± 2 °C). An increase in temperature increased the reaction rates (2.5 × 10−3 min−1 and 2.8 × 10−3 min−1 at 45 and 65 °C, respectively) and DCP removal up to 75% in 4 h, which may be attributed to a positive effect of temperature on the adsorption velocity and equilibrium adsorption capacity.33
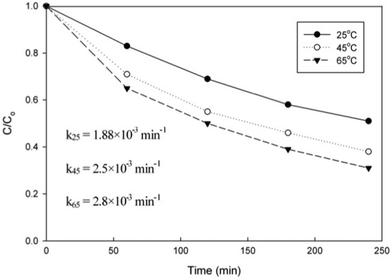 |
| Fig. 8 DCP degradation under the visible light irradiation at various temperatures. | |
Important, demineralization of the solution in terms of TOC degradation of 18, 59, and 74% was achieved with 0.5, 1, and 2 g L−1 of Veruclay, respectively with DCP as a contaminant, which emphasizes the efficiency of Veruclay preparation and relevant reaction conditions.
3.4 Inhibition of bacterial growth
Fig. 9 shows the inhibition of luminescent activity of V. fischeri in the presence of K10, Veruclay, sensitized Veruclay, and sensitized and recycled Veruclay. The lowest bacterial growth inhibition (from 1 to 16.8%) was achieved with the lowest (1 and 5 mg L−1) K10 and Veruclay concentrations.
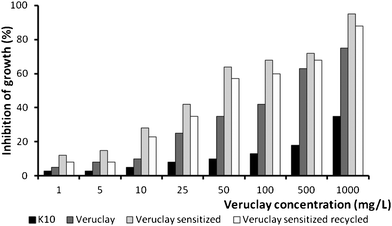 |
| Fig. 9 Antimicrobial activity of Veruclay. | |
However, when the dosage was increased from 10 to 1000 mg L−1, inhibition of the growth was nearly 75% and 98% in the presence of Veruclay and sensitized Veruclay, respectively, indicating that MB may have strengthened the antimicrobial activity of Veruclay.37 In addition, after recycling, sensitized Veruclay exhibited only slightly lower (ca. 7%) antimicrobial activity (Fig. 9), which indicated the stability and efficiency of Veruclay towards bacterial growth inhibition.
3.5 Recyclability of Veruclay
Every synthesized catalyst, adsorbent, or catalytic adsorbent that is relevant to environmental applications must be recyclable and must retain most of its’ activity for practical purposes. To evaluate the recyclability of Veruclay, it was subjected to a simple regeneration procedure, which was modified to lower temperatures (300 °C) due to the fact that VeruSOL decomposes at higher temperatures.28Fig. 10 presents the activity of Veruclay in 7 consecutive runs as well as 5 more consecutive runs with the calcined sample.
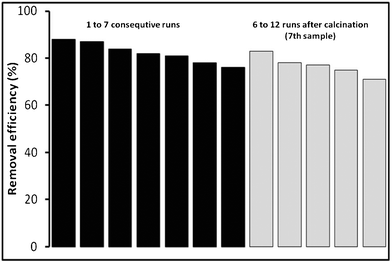 |
| Fig. 10 Effectiveness towards MB disappearance and recyclability of Veruclay. | |
As could be seen in Fig. 10, there was a slight decrease in MB degradation from 88% to 76%. Ramirez et al. and Centi et al. attributed it to the decline in the specific surface area of the adsorbent and poisoning of the active sites due to the interaction with the organic species.38,39
After calcination, however, Veruclay regained most of its activity and was effective in degrading MB for an additional five runs.
4 Conclusions
To be sustainable, it is vital to use benign and abundant precursors to fabricate effective catalytic adsorbents that are applicable to environmental remediation. In this study, a novel Veruclay, which is an organic surfactant-modified montmorillonite, was developed by a simple synthesis procedure. Veruclay showed photo-activity in the visible light range towards adsorption and degradation of MB and DCP in aqueous solutions. Further, it demonstrated excellent stability for up to 7 consecutive runs and an additional 5 runs after a simple calcination step. In addition, Veruclay demonstrated outstanding antibacterial properties against V. fischeri. These properties could be utilized for the simultaneous abatement of chemical and microbial pollution in water bodies. It is anticipated that Veruclay can be used not only for environmental remediation, but in organic transformations and other important fields.
Acknowledgements
This research was performed at the National Risk Management Research Laboratory of U.S. Environmental Protection Agency (EPA), Cincinnati, Ohio. This paper has not been subjected to internal policy review of the U.S. EPA. Therefore, the research results do not necessarily reflect the views of the agency or its policy. Mention of trade names and commercial products does not constitute endorsement or recommendation for use. The CRADA support from VeruTEK Technologies, Inc. is gratefully acknowledged.
References
- C.-C. Wang, L.-C. Juang, C.-K. Lee, T.-C. Hsu, J.-F. Lee and H.-P. Chao, J. Colloid Interface Sci., 2004, 280, 27–35 CrossRef CAS.
- S. Zhu, H. Hou, Y. Xue, N. Wei, Q. Sun and X. Chen, J. Colloid Interface Sci., 2007, 315, 8–12 CrossRef CAS.
- Z. Jianxi, H. Hongping, G. Jiuga, Y. Dan and X. Xiande, Chinese Sci. Bull., 2003, 48, 368–372 Search PubMed.
- B. Chen, L. Zhu, J. Zhu and B. Xing, Environ. Sci. Technol., 2005, 39, 6093–6100 CrossRef CAS.
- H. He, Y. Ma, J. Zhu, P. Yuan and Y. Qing, Appl. Clay Sci., 2010, 48, 67–72 CrossRef CAS.
- A.-H. Chen and S.-M. Chen, J. Hazard. Mater., 2009, 172, 1111–1121 CrossRef CAS.
- L. Wang and A. Wang, J. Hazard. Mater., 2008, 160, 173–180 CrossRef CAS.
- M. Mortimer, K. Kasemets, M. Heinlaan, I. Kurvet and A. Kahru, Toxicol. in Vitro, 2008, 22, 1412–1417 CrossRef CAS.
- T. L. Barr, Appl. Surf. Sci., 1983, 15, 1–35 CrossRef CAS.
- Z. B. Molu and K. Yurdakoç, Microporous Mesoporous Mater., 2010, 127, 50–60 CrossRef CAS.
- ISO 11348-3 2007.
- S. Parvez, C. Venkataraman and S. Mukherji, Environ. Int., 2006, 32, 265–268 CrossRef CAS.
- J. Lappalainen, R. Juvonen, J. Nurmi and M. Karp, Chemosphere, 2001, 45, 635–641 CrossRef CAS.
- N. Strigul, L. Vaccari, C. Galdun, M. Wazne, X. Liu, C. Christodoulatos and K. Jasinkiewicz, Desalination, 2009, 248, 771–782 CrossRef CAS.
- P. Alba, S. Sánchez-Fortún, S. Alvarez-Perez, J. L. Blanco and M. E. García, Toxicon, 2009, 53, 729–733 CrossRef CAS.
- J. Virkutyte and R. S. Varma, RSC Adv., 2012, 2, 1533–1539 RSC.
- L. Zhou, H. Chen, X. Jiang, F. Lu, Y. Zhou, W. Yin and X. Ji, J. Colloid Interface Sci., 2009, 332, 16–21 CrossRef CAS.
- A. K. Mishra, S. Allauddin, R. Narayan, T. M. Aminabhavi and K. V. S. N. Raju, Ceram. Int., 2012, 38, 929–934 CrossRef CAS.
- T. Okada, H. Sakai and M. Ogawa, Appl. Clay Sci., 2008, 40, 187–192 CrossRef CAS.
- M. Sarkar, K. Dana and S. Ghatak, J. Molec. Struct., 2011, 1005, 161–166 CAS.
- R. R. Tiwari, K. C. Khilar and U. Natarajan, Appl. Clay Sci., 2008, 38, 203–208 CrossRef CAS.
- A. A. Yehia, A. M. Akelah, A. Rehab, S. H. El-Sabbagh, D. E. El Nashar and A. A. Koriem, Mater. Des., 2012, 33, 11–19 CrossRef CAS.
- F. Z.-J. He Man-Chao and Ping Zhang, Chin. Phys. B, 2009, 18, 2933–2937 CrossRef.
- J. Virkutyte and R. S. Varma, Sep. Purif. Technol., 2011, 78, 201–207 CAS.
- M. Ogawa, T. Ishii, N. Miyamoto and K. Kuroda, Appl. Clay Sci., 2003, 22, 179–185 CrossRef CAS.
- J. Virkutyte, B. Baruwati and R. S. Varma, Nanoscale, 2010, 2, 1109–1111 RSC.
- Y.-L. Ma, Z.-R. Xu, T. Guo and P. You, J. Colloid Interface Sci., 2004, 280, 283–288 CrossRef CAS.
- J. Virkutyte and R. S. Varma, RSC Adv., 2012, 2, 3416–3422 RSC.
- M. G. Neumann, F. Gessner, C. C. Schmitt and R. Sartori, J. Colloid Interface Sci., 2002, 255, 254–259 CrossRef CAS.
- T. Ebina, T. Iwasaki, A. Chatterjee, M. Katagiri and G. D. Stucky, J. Phys. Chem. B, 1997, 101, 1125–1129 CrossRef CAS.
- W. Liu, Y. Ni and H. Xiao, J. Colloid Interface Sci., 2004, 275, 584–589 CrossRef CAS.
- R. Liu, R. L. Frost, W. N. Martens and Y. Yuan, J. Colloid Interface Sci., 2008, 327, 287–294 CrossRef CAS.
- C. Yan, C. Wang, J. Yao, L. Zhang and X. Liu, Colloids Surf., A, 2009, 333, 115–119 CrossRef CAS.
- G. Gereli, Y. Seki, İ. Murat Kuşoğlu and K. Yurdakoç, J. Colloid Interface Sci., 2006, 299, 155–162 CrossRef CAS.
- F. Franco, J. Environ. Sci., 2010, 22, 467–473 CrossRef.
- X. Zhao, L. Zhu, Y. Zhang, J. Yan, X. Lu, Y. Huang and H. Tang, J. Hazard. Mater., 2012, 215–216, 57–64 CrossRef CAS.
- M. Wainwright, D. A. Phoenix, M. Gaskell and B. Marshall, J. Antimicrob. Chemother., 1999, 44, 823–825 CrossRef CAS.
- G. Centi, S. Perathoner, T. Torre and M. G. Verduna, Catal. Today, 2000, 55, 61–69 CrossRef CAS.
- J. H. Ramirez, C. A. Costa, L. M. Madeira, G. Mata, M. A. Vicente, M. L. Rojas-Cervantes, A. J. López-Peinado and R. M. Martín-Aranda, Appl. Catal., B, 2007, 71, 44–56 CrossRef CAS.
|
This journal is © The Royal Society of Chemistry 2012 |