DOI:
10.1039/C2RA20687K
(Paper)
RSC Adv., 2012,
2, 8410-8415
Controlled self-assemblies of clay silicate platelets by organic salt modifier†
Received
17th April 2012
, Accepted 7th July 2012
First published on 10th July 2012
Abstract
We observed unique cubic microstructures and hierarchical dendrite formation from clay silicate platelet self-piling. The fundamental units of silicate platelets with dimensions of ca. 80 × 80 × 1 nm were previously prepared from natural clay stacks. By ionic exchange with the hydrochloride salt of diethylene glycolamine, the platelets could be connected with polar organic moieties. The self-piling of these silicate platelets generated cubic arrays of 1–4 μm in size that differed from the rod-like microstructures of 10–60 μm in length and 0.5–1.5 μm in diameter for non-modified platelets. The cubic self-assemblages were characterized to be hollow in structure by energy dispersive X-ray spectrometry for elemental analysis, scanning electron microscopy, and transmission electron microscopy. Dendrite arrays were further observed over a large area of a millimeter square, indicating high regularity of the cube unit interconnection. Both charge attraction and organic interaction had shifted the platelet piling directions, favoring 3-D cubes and further facilitating the formation of hierarchical dendrites.
1. Introduction
Self-assembly of surfactants and amphiphilic copolymers is well recognized for the formation of ordered structures in various morphologies and sizes. Bottom-up processes may be developed for synthesizing nanometer-sized organic structures1–4 for templating inorganic particles5–8 into nanomaterials. It is further demonstrated that the self-assemblages with dimensions of 1 to 100 nm could give rise to new physical and chemical properties.9–12 In the past, the nanomaterials with different shapes such as nano-sphere,13 -cube,14 -wire,15 -tube,16 -fiber,17 -ring,18 -plates19etc. have been widely reported. These self-assembling occurrences are mechanistically rationalized by the equilibrium of non-covalent bonding forces such as ionic charge interaction,20 hydrogen bonding,21 aromatic π–π stacking,22 van der Waals force,23 and hydrophobic effect.24
The natural phyllosilicate clays are crystalline aluminosilicates and characterized as the multilayered structure in the primary stacks. Among many varieties of natural inorganic minerals, the smectite clays commonly comprise two tetrahedral sheets and a sandwiched octahedral sheet as an edge-sharing 2
:
1 structure. The plate-like clays have various practical use such as catalysts,25 adsorbents,26 metal chelating agents,27 and polymer/clay nanocomposites.28–30 For the montmorillonite clay, the multilayered structure consists of 8–10 platelets on average in one stack, with platelet units of polydispersed dimensions of 80 × 80 × 1 nm. The individual platelets are tightly bonded together through the counter metal ions in one primary stack.31–33 Previously, it was reported that organically intercalated clay stacks at a proper organic/silicate ratio may undergo a self-assembling process to generate highly ordered microstructures.8 The self-assembly was attributed to the presence of the non-covalent bonding forces involving silicate ionic charges and organic interactions in the primary units of clay stacks. However, randomized silicate platelets after removing the organic exfoliating agents34–38 were also reported for the ability of self-assembling behavior.39 Since the individual platelets were isomeric structures from the original multilayered clay stacks, the ionic charge interaction was realized to the predominant parameter for these self-assemblies. It is further noted that the exfoliated platelets in aqueous suspension have their complete exposure of surface area, expressing high surface area and ca. 18
000 ionic charges per platelet.40,41 The organic-free platelets have demonstrated their high tendency for self-piling via two different directions, platelet face-to-face vertical and edge-to-edge horizontal growths into the ordered microstructures. With respect to an organic modification for the clay platelets, it was separately reported that poly(N-isopropylacrylamide) (PNiPAAm) covalently connected platelets tended to self-assemble into 3D nanodomain networks.42 In forming such networks, the PNiPAAm organic attachments had a significant influence on the platelet self-piling through the factor of lower critical solution temperature or LCST of polymer interaction with water.
In order to understand the clay self-assembling behavior, we have modified the platelet surface by organic amine-salts through an ionic exchange reaction and explored the effect of organic salts in replacing the sodium counter ions in clay. By varying the amount of organic salts to sodium counter ions in the platelets, the self-assembly has led to a different formation of cubic-shape arrays from the original rod-like morphology. The morphological change through modifying the fundamental unit structure by exchanging with organic amine-salts allows further understanding of the self-piling mechanism. The morphological arrays were characterized by energy dispersive X-ray spectrometry (EDS), scanning electronic microscopy (SEM) and transmission electron microscopy (TEM). The mechanism involving clay platelet unit piling and surface organic interaction is proposed to account for the clay self-assembling behavior.
2. Experimental
2.1 Materials
Sodium montmorillonite (Na+-MMT), a layered silicate clay with a cationic exchange capacity (CEC) of 120 mequiv/100 g, was supplied from Nanocor Co., USA. The 2/1 type of the natural smectite clays is aggregates from unit stacks of approximately 8–10 platelets in an average stack.40,41 Each layer of aluminosilicates typically constitutes two tetrahedron sheets sandwiching with an edge-shared octahedral sheet. The individual layer units are polydispersed with dimensions of ca. 80 × 80 × 1 nm. Diethylene glycolamine or diethanolamine (HOCH2CH2OCH2CH2NH2) as the common name (DGA, Mw 105 g mol−1) was purchased from Aldrich Chemical Co.
2.2 Exfoliation of layered Na+-MMT into random platelets in water suspension
The preparation of random nanosilicate platelets (NSP) from the exfoliation of Na+-MMT by using polyamine quaternary salts has been reported previously.34–38 The exfoliated platelets were isolated by toluene/NaOH phase extraction several times to remove organic amines. The NSP was dispersible in methanol/water at 60 °C and settled into two layers with toluene additions. The aqueous phase containing the silicate platelet suspension was separated and purified. The isolated NSP material, with ionic charges of 18
000 ions per plate in the form of
SiO−Na+, were hydrophilic and gelling in water, and estimated to be ca. 80 × 80 × 1 nm. The measurement of zeta potentials demonstrated its ionic characteristics depending on pH environment and an isoelectric point at pH 6.4.35 Atomic force microscopy analysis from a high dilution of a dispersion sample had shown that the NSP was individually separated in one platelet unit or two platelets associated together in a lamellar structure. The fine dispersion of NSP in aqueous medium was subjected to controlled evaporation to remove water, the sample was in aggregates as powder and analyzed by EDS, SEM, and TEM.
2.3 Preparation of self-assemblies from the random silicate platelet anchored with polar organic amine salts
The solution of diethylene glycolamine (DGA) was dissolved in water and aqueous hydrogen chloride added in 1
:
1 ratio. The DGA salt was then added to the NSP dispersion in ratios of 1
:
0.2, 1
:
0.5, and 1
:
1, based on the clay CEC. The solution of 1 wt% of NSP/DGA complex was thinly coated on glass and then dried at 80 °C evaporation in an oven for 8 h. Viscous semi-solid material was obtained and analyzed.
2.4 Characterization and instruments
The surface tension was measured by the Wilhelmy method using a Kruss-K10 digital tensiometer equipped with a spherical ring. The thermal analyses were analyzed by using a thermal gravimetric analyzer (TGA), on a Perkin-Elmer Pyris 1 model. The organic weight was estimated from the weight losses by ramping the temperature from 100 to 850 °C at a rate of 10 °C min−1 in air. Scanning electron microscopy (SEM) was performed on a JEOL JSM-5600 SEM system and operated at 15 kV. The samples were prepared by spreading onto a glass plate surface and evaporating to dryness in an oven at 80 °C for 8 h. The samples were coated with Au before the SEM measurements. The surface element analysis was performed by energy dispersive X-ray spectroscopy (EDS, OXFORD INCA ENERGY 400). Transmission electron microscopy (TEM) was performed on a Zeiss EM 902A operated at 80 kV. The NSP/DGA sample was cut into a wedge shape and then embedded in a polyethylene mold using epoxy resin. The specimen was trimmed to a trapezoid shape of approximately 80 nm in thickness, microtomed at room temperature using a diamond knife on a Leica Ultracut UCT6 system, and collected on a 200-mesh carbon-coated Cu grid for analysis. A ZetaPlus zetameter (Brookhaven Instrument Corp., NJ) was used for characterizing the ionic properties of the NSP. The zeta potentials of the NSP and the NSP/DGA complex in aqueous suspension of 0.01 wt% concentration were measured.
3. Results and discussion
3.1 Modifying NSP with organic salt through an ionic exchange reaction
Nanoscale silicate platelets (NSP), the self-assembling primary units, were prepared according to the procedures reported previously.34,35 The pristine clay stacks could be exfoliated by a polyamine-salt to generate randomized platelets and further isolated by a biphase toluene/water extraction process to remove the organic agent. The overall process is conceptually described in Scheme 1a. The purified NSP were characterized to be silicate compositions with thin platelet geometric shapes and ionic charges (
SiO−Na+ functionalities) at cationic exchange capacity (CEC) of 120 mequiv/100 g. It was estimated that NSP have a high surface area of 720 m2 g−1, ionic character of 18
000 ions per platelet or 0.9 nm2 area per ion, and 4 × 1010 platelets per gram.8 With an averaged dimension of 80 × 80 × 1 nm, the extremely thin NSP have the tendency to interact with polar organics and water to form gel products.43 Furthermore, the presence of counter ions rendered the platelets self-assembling during water evaporation and forming rod-like or fibrous arrays. However, the occurrence of self-assembled microstructures was difficult to control and less reproducible. In order to understand the self-assembling behavior, we investigated the use of low-molecular-weight amine-salts for modifying the ability of the NSP self-piling. The water-soluble amine-salt or the hydrogen chloride treated diethylene glycolamine (abbreviated DGA or HOCH2CH2OCH2CH2NH3+Cl−) was selected as the organic modifier. The DGA salts were simply generated by treating the amine with aqueous hydrogen chloride solution at an equal equivalent. The replacement of sodium counter ions with the DGA salts will divert the platelet self-assembling behavior because of the changes in the surface attracting force. The ionic exchange reaction is generally fast for DGA salt to interact with the NSP surface counter ions. The Na+ ions of representative
SiO−Na+ functionalities are exchangeable and allowed anchoring of the organic moiety. The amount of DGA salts for the replacement is screened by varying the equivalents of NSP ions up to a maximum value of the clay exchange capacity of 120 mequiv/100 g. The ionic complexes of NSP and the DGA salts were prepared in three different molar ratios of 1
:
0.2, 1
:
0.5, and 1
:
1 (Table 1). The generated NSP/DGA complexes possessed amphiphilic surface properties of lowering the air/water interface tension. At a concentration of 0.1 wt%, the aqueous solution of NSP/DGA at 1
:
0.2 molar ratio was capable of lowering the surface tension from 70.1 to 40.1 mN m−1, as shown in Fig. 1. For comparison, the increase in DGA ratios from 0.2 to 0.5, and then 1.0 equivalent rendered to the complexes a smaller degree of lowering the surface tension. The demonstration of changes in the surface tension implies tendency for aggregation by themselves at the air/water interface. However, the solution behavior had an implication for the ability of NSP self-assemblies under the conditions of water evaporation into dryness.
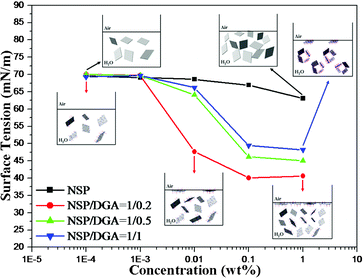 |
| Fig. 1 Surface tension energy changes of adding the NSP/DGA complex in water. | |
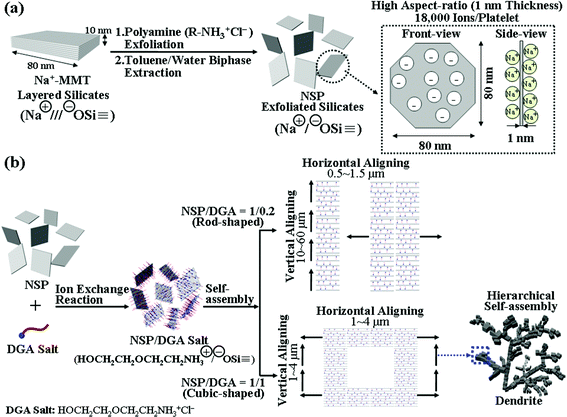 |
| Scheme 1 (a) Schematic view of the exfoliation of a clay layered structure into randomized silicate platelets (NSP) and description of the surface ionic charges on the thin geometric shape unit. (b) Conceptual illustration of NSP/DGA self-piling routes of vertical versus horizontal growth by varying the DGA amount. | |
Table 1 Occurrences of self-assembling from the NSP and DGA promoter by ionic exchange reaction at different equivalent ratios
|
Ion equivalent ratio |
Weight fraction (w/w)b |
Zeta potential (mV)c |
Observed self-assemblages by SEM |
Diethylene glycolamine-hydrochloride salt or HOCH2CH2OCH2CH2NH3+Cl−.
Weight fraction of silicates/organics, corresponding to the ionic charge ratio based on the clay counter ions or cationic exchange capacity (CEC, 120 mequiv/100 g).
NSP/DGA complex (0.01 wt%) in water.
|
NSP/DGAa |
1/0.2 |
96/4 |
−46.2 |
Rod-like arrays (10–60 μm in length and 0.5–1.5 μm in width) |
|
1/0.5 |
85/15 |
−43.2 |
Cubes (1–4 μm in three dimensions of length, width, and height) |
|
1/1 |
70/30 |
−42.0 |
Cubes (1–4 μm in three dimensions of length, width, and height) and large-scale hierarchical dendrite arrays through cube unit interconnection) |
NSP |
1/0 |
100/0 |
−46.6 |
Rod-like arrays (0.85–3.5 μm in length and 0.1–1 μm in width) |
DGA salt |
0/1 |
0/100 |
– |
None |
Na+-MMT |
1/0 |
100/0 |
−25.9 |
None |
3.2 Diverted self-assembling from the formation of lengthy rods to hollow cubes, and hierarchical cube-united dendrites
The NSP/DGA complexes were easily dispersed in water at a concentration of 1 wt%. The self-assemblages were obtained after coating the solution on a glass substrate and heating to 80 °C until dryness. Based on SEM analyses as shown in Fig. 2, directional and ordered rod-like arrays were observed when the NSP/DGA equivalent ratio was adjusted to 1
:
0.2 that corresponded the the DGA organic composition of 4 wt% based on NSP. The morphology of lengthy rods up to 60 μm was observed and implied a strong platelet face-to-face directional stacking propensity or a 2D directional growth. The less straight rod-like arrays or more growth in width were derived from another direction of horizontal platelet edge-to-edge alignment.
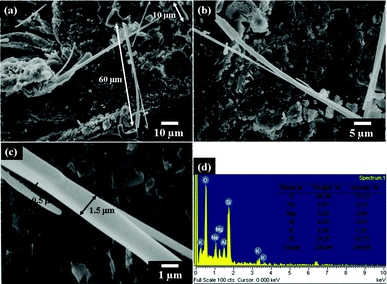 |
| Fig. 2 (a–c) SEM micrographs of lengthy rods from the self-piling of NSP/DGA (equivalent ratio 1 : 0.2) at different magnifications. (d) EDS analysis of the rods. | |
The rod width was in the range of 0.5–1.5 μm in diameter. With increasing the amount of DGA to NSP at two equivalent ratios of 1
:
0.5 and 1
:
1 or DGA organics of 15 and 30 wt%, respectively, the cubic-shape morphology appeared as major self-assemblages (Fig. 3 and Fig. S1, ESI†). The self-assembling route was altered from the formation of lengthy rods at a tenth of micrometer to the cubes at 1–4 μm for three dimensions of length, width, and height. This occurrence implies change of the mechanism from the major face-to-face unit piling directions owing to the presence of DGA tethering on the NSP surface. The addition of DGA salts could largely affect the platelet face-to-face attraction through changes in the ionic character and organic interaction. Further, the existence of secondary hierarchical structures was detected. The SEM observations at different magnifications allow a large-scale view of the cube unit interconnection in forming dendrite arrays (Fig. 3a and b). The formation is resembling the morphology of naturally occurring fern-like plants. The continuous branches had extended to a large area over 1.0 mm which was visible by the naked eye. The large-scale array formation is attributed to the strong aggregation force for interconnection of the cubic-shaped units, presumably by the DGA organic hydrophilic affinity besides the NSP self-piling force. The presence of DGA organics is essential since the control experiments demonstrated irregular aggregation for the aqueous suspension of pristine Na+-MMT (Fig. 4a and b) and rod-like morphology rather than the cubic shape for the NSP self-piling (Fig. 4c and d).
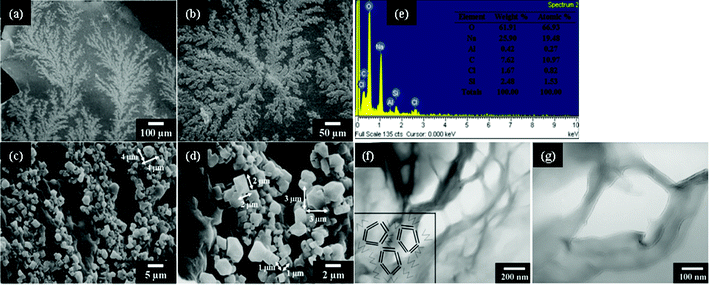 |
| Fig. 3 SEM micrographs (at different magnifications): (a–d) cubes from the self-piling of NSP/DGA (equivalent ratio 1 : 1); (e) EDS analysis of NSP/DGA cubes. (f and g) TEM cross-section micrograph of the hollow cubes from NSP/DGA. The inserted diagram in Fig. 3f is a conceptual illustration of the hollow cubes from the cross-sectional view. | |
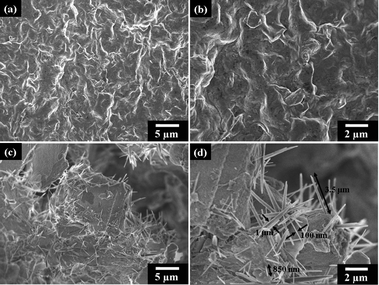 |
| Fig. 4 SEM micrographs of the controlled self-assembled morphologies; (a and b) none of the ordered structure from the pristine Na+-MMT under self-assembling conditions and (c and d) rod-like microstructures from the NSP (without DGA modification) self-piling. All samples were prepared by the same method of controlled water evaporation on glass. | |
Elemental analysis by energy dispersive X-ray spectroscopy (EDS) had indicated distributions of oxygen 72.13%, sodium 4.14%, aluminum 3.07%, magnesium 2.80%, potassium 1.11%, and silicon 16.75% for the rods (Fig. 2d) and oxygen 66.93%, sodium 19.48%, aluminum 0.27%, carbon 10.97%, chlorine 0.82%, silicon 1.53% for the cubes (Fig. 3e). This is consistent with the theoretical calculations for the NSP/DGA at 1
:
0.2 and 1
:
1 equivalent ratio compositions of oxygen 52.21%, sodium 3.08%, aluminum 11.85%, magnesium 1.58%, potassium 0.03%, silicon 28.46%, carbon 1.83%, hydrogen 0.42%, nitrogen 0.54% for the rods and oxygen 46.28%, sodium 2.24%, aluminum 8.65%, magnesium 1.15%, potassium 0.02%, silicon 20.75%, carbon 13.71%, hydrogen 3.15%, and nitrogen 4.05% for the cubes. It is noted that the sodium content is higher than the calculated values, indicating the sodium salt accumulation on the surface. Furthermore, hollow cube microstructures were observed on a TEM cross-section micrograph (Fig. 3f and g), showing the image of silicate platelets surrounding the empty cages. The hollow cubic-shaped arrays of 200 nm dimensions in a TEM micrograph are smaller than 1 μm in a SEM image, perhaps due to shrinking or collapse of the cubic cages during the TEM sample preparation involving epoxy resin during the encapsulation process. Recently, the formation of a hollow structure has been similarly reported in the case of organoclays that were prepared from the intercalation of organic ions into a montmorillonite clay layered structure.44 It was shown that the organoclays enabled to form self-aligned hollow microspheres of 3–9 μm in diameter during the spray drying process. It appeared that the silicate clays with proper organic involvement had a tendency for self-aligning into the plate-to-plate overlapping arrangement.
The thin thickness of geometric shape and the ionic character on the surface are two dominant factors for favoring the silicate platelet self-piling the vertical direction. The presence of DGA modification on NSP could divert the platelet piling directions. Conceptually demonstrated are the two piling directions for generating rod versus cube morphologies (Scheme 1b). The vertical growth is mainly controlled by the interaction of neighboring platelet sodium counter ions (
SiO−Na+), estimated to be 18
000 ions for each platelet. Through the exchange with the DGA salts, the NSP surface was tethered by the polar organics and demonstrated different zeta potentials (Table 1 and Fig. S2, ESI†). It favors the edge-to-edge horizontal growth by DGA organic interaction, ultimately leading to the formation of 3-D cube-shape units. The organic presence further contributed to the hierarchical dendrite arrays on a large scale. Although the driving force is not well understood, the cube-shape units appeared to organize themselves in a manner minimizing the excess surface energy of the cubes that could form a hierarchical dendrite array.45–50 From the EDS elemental analysis, it could be seen that the cube surface appeared to have a high composition of sodium chloride (sodium 19.48% and chloride 0.82% for the cubes vs. sodium 4.14% and chloride 0% for the rods). In considering the potential chelating nature of DGA, the tethered organic moiety of HOCH2CH2OCH2CH2NH3+ in complexing with NSP surface (
SiO−) could attract sodium ions in the proximity and facilitate the platelet edge-to-edge piling direction.
4. Conclusion
The self-assembly of the exfoliated clay platelets into either lengthy fibrous or 3D cubic arrays was controllable by adding an organic amine-salt modifier. The original self-assembling route involving the platelet face-to-face self-piling mechanism was altered through the organic involvement for the platelet surface interaction. The platelets with surface-tethered organic salts favored an edge-to-edge self-piling into 3D cubic arrays owing to the presence of organic salts on the platelet surface. The organic presence further affected the secondarily hierarchical formation of dendrites from the cubic units. The addition of amine-salt organics offers a method of controlling the clay platelet piling and a new design for self-assembling microstructures.
Acknowledgements
We acknowledge financial support from the National Taiwan University of Science and Technology, the Ministry of Economic Affairs, and the National Science Council (NSC) of Taiwan.
References
- G. M. Whitesides and B. Grzybowski, Science, 2002, 295, 2418–2421 CrossRef CAS.
- J. K. Kim, S. Y. Yang, Y. Lee and Y. Kim, Prog. Polym. Sci., 2010, 35, 1325–1349 CrossRef CAS.
- S. B. Darling, Prog. Polym. Sci., 2007, 32, 1152–1204 CrossRef CAS.
- R. M. Ho, Y. W. Chiang, S. C. Lin and C. K. Chen, Prog. Polym. Sci., 2011, 36, 376–453 CrossRef CAS.
- Y. Kondo and K. Takayangi, Science, 2000, 289, 606–608 CrossRef CAS.
- Y. Zhang, K. Suenaga, C. Colliex and S. Iijima, Science, 1998, 281, 973–975 CrossRef CAS.
- D. Yu and V. W. W. Yam, J. Am. Chem. Soc., 2004, 126, 13200–13201 CrossRef CAS.
- C. W. Chiu and J. J. Lin, Prog. Polym. Sci., 2012, 37, 406–444 CrossRef CAS.
-
K. L. Chopra, Thin Film Phenomena, McGraw-Hill, New York, 1969 Search PubMed.
- Y. Cui, M. T. Bjork, J. A. Liddle, C. Sonnichsen, B. Boussert and A. P. Alivisatos, Nano Lett., 2004, 4, 1093–1098 CrossRef CAS.
- A. Mikrajuddin, F. Iskandar and K. Okuyama, Adv. Mater., 2002, 14, 930–933 CrossRef.
- C. W. Chiu, P. D. Hong and J. J. Lin, Langmuir, 2011, 27, 11690–11696 CrossRef CAS.
- R. Gref, Y. Minamitake, M. T. Peracchia, V. Trubetskoy, V. Torchilin and R. Langerll, Science, 1994, 263, 1600–1603 CAS.
- A. S. Edelstein, G. M. Chow, E. I. Altman, R. J. Colton and D. M. Hwang, Science, 1991, 251, 1590–1592 CAS.
- C. W. Na, H. S. Woo and J. H. Lee, RSC Adv., 2012, 2, 414–417 RSC.
- J. Luo, L. Gao, J. Sun and Y. Liu, RSC Adv., 2012, 2, 1884–1889 RSC.
- J. D. Hartgerink, E. Beniash and S. I. Stupp, Science, 2001, 294, 1684–1688 CrossRef CAS.
- S. A. Jenekhe and X. L. Chen, Science, 1999, 283, 372–375 CrossRef CAS.
- R. Jin, G. Chen, J. Pei, H. Xu and Z. S. Lv, RSC Adv., 2012, 2, 1450–1456 RSC.
- L. E. Euliss, S. G. Grancharov, S. O'Brien, T. J. Deming, G. D. Stucky, C. B. Murray and G. A. Held, Nano Lett., 2003, 3, 1489–1493 CrossRef CAS.
- W. D. Xiao, Y. H. Jiang, K. Ait-Mansour, P. Ruffieux, H. J. Gao and R. Fasel, J. Phys. Chem. C, 2010, 114, 6646–6649 CAS.
- M. Li, H. J. Xu, K. Deng, Z. Shen, X. Z. You, Q. D. Zeng and C. Wang, J. Phys. Chem. C, 2010, 114, 1881–1884 CAS.
- T. D. Lazzara, T. G. M. van de Ven and M. A. T. Whitehead, Macromolecules, 2008, 41, 6747–6751 CrossRef CAS.
- L. Hua, R. Zangi and B. J. Berne, J. Phys. Chem. C, 2009, 113, 5244–5253 CAS.
- T. J. Pinnavaia, Science, 1983, 220, 365–371 CAS.
- R. Celis, M. C. Hermosin, M. J. Carrizosa and J. Cornejo, J. Agric. Food Chem., 2002, 50, 2324–2330 CrossRef CAS.
- Z. Kiraly, B. Veisz, A. Mastalir and G. Kofarago, Langmuir, 2001, 17, 5381–5387 CrossRef CAS.
- S. S. Ray and M. Okamoto, Prog. Polym. Sci., 2003, 28, 1539–1641 CrossRef CAS.
- S. Pavlidou and C. D. Papaspyrides, Prog. Polym. Sci., 2008, 33, 1119–1198 CrossRef CAS.
- P. Kiliaris and C. D. Papaspyrides, Prog. Polym. Sci., 2010, 35, 902–958 CrossRef CAS.
- M. Alexandre and P. Dubois, Mater. Sci. Eng., R, 2000, 28, 1–63 CrossRef.
- A. Usuki, N. Hasegawa, H. Kadoura and T. Okamoto, Nano Lett., 2001, 1, 271–272 CrossRef CAS.
- L. A. Utracki, M. Sepehr and E. Boccaleri, Polym. Adv. Technol., 2007, 18, 1–37 CrossRef CAS.
- C. C. Chu, M. L. Chiang, C. M. Tsai and J. J. Lin, Macromolecules, 2005, 38, 6240–6243 CrossRef CAS.
- J. J. Lin, C. C. Chu, M. L. Chiang and W. C. Tsai, J. Phys. Chem. B, 2006, 110, 18115–18120 CrossRef CAS.
- C. W. Chiu, C. C. Chu, W. T. Cheng and J. J. Lin, Eur. Polym. J., 2008, 44, 628–636 CrossRef CAS.
- J. J. Lin, C. C. Chu, M. L. Chiang and W. C. Tsai, Adv. Mater., 2006, 18, 3248–3252 CrossRef CAS.
- C. W. Chiu, C. C. Chu, S. A. Dai and J. J. Lin, J. Phys. Chem. C, 2008, 112, 17940–17944 CAS.
- J. J. Lin, C. C. Chu, C. C. Chou and F. S. Shieu, Adv. Mater., 2005, 17, 301–304 CrossRef CAS.
-
H. V. Olphen, Clay Colloid Chemistry, 2nd ed., John Wiley & Sons, New York, 1997 Search PubMed.
-
B. K. G. Theng, The Chemistry of Clay-Organic Reactions, 2nd ed., John Wiley & Sons, New York, 1974 Search PubMed.
- Y. M. Chen, H. C. Lin, R. S. Hsu, B. Z. Hsieh, Y. A. Su, Y. J. Sheng and J. J. Lin, Chem. Mater., 2009, 21, 4071–4079 CrossRef CAS.
- J. C. Wei, Y. T. Yen, H. L. Su and J. J. Lin, J. Phys. Chem. C, 2011, 115, 18770–18775 CAS.
- X. Du, Z. Jiang, X. Meng, Z. Wang, H. Yu, M. Li and T. Tang, J. Phys. Chem. C, 2008, 112, 6638–6642 CAS.
- D. Wang, B. Tejerina, I. Lagzi, B. Kowalczyk and B. A. Grzybowski, ACS Nano, 2011, 5, 530–536 CrossRef CAS.
- D. A. Walker, B. Kowalczyk, M. O. Cruz and B. A. Grzybowski, Nanoscale, 2011, 3, 1316–1344 RSC.
- A. M. Kalsin, B. Kowalczyk, S. K. Smoukov, R. Klajn and B. A. Grzybowski, J. Am. Chem. Soc., 2006, 128, 15046–15047 CrossRef CAS.
- A. M. Kalsin and B. A. Grzybowski, Nano Lett., 2007, 7, 1018–1021 CrossRef CAS.
- A. M. Kalsin, M. Fialkowski, M. Paszewski, S. K. Smoukov, K. J. M. Bishop and B. A. Grzybowski, Science, 2006, 312, 420–424 CrossRef CAS.
- K. J. M. Bishop, C. E. Wilmer, S. Soh and B. A. Grzybowski, Small, 2009, 5, 1600–1630 CrossRef CAS.
Footnote |
† Electronic supplementary information (ESI) available: SEM micrographs and zeta potentials of NSP/DGA complex are available in the supplemental information. See DOI: 10.1039/c2ra20687k/ |
|
This journal is © The Royal Society of Chemistry 2012 |