DOI:
10.1039/C2RA20618H
(Paper)
RSC Adv., 2012,
2, 6306-6314
Efficient and selective enzymatic synthesis of N-acetyl-lactosamine in ionic liquid: a rational explanation†
Received
3rd April 2012
, Accepted 12th April 2012
First published on 14th June 2012
Abstract
Room temperature ionic liquids (ILs) can affect enzyme activity in some enzyme-catalyzed reactions, however the effects of these cosolvents on the enzymes are not clearly understood. Using β-galactosidase from Thermus thermophilus HB27 (TTP0042), we found an important change from the classical regioselectivity of the transglycosylation reaction with this enzyme. The enzyme increases N-acetyl-D-lactosamine synthesis (Galβ[1→4]GlcNAc) when RTILs are used instead of the traditional self-condensated products. To understand the possible effect of these liquids on the synthetic behavior of the enzyme, we performed a molecular interaction study by surface plasmon resonance. The KD value obtained for this interaction could mean that ILs bind to β-galactosidase through non specific interactions characterized by very fast kinetics and millimolar affinity. Then, several reactions were performed, increasing the concentration of the IL. As a result, a dependence on the IL's concentration was found for transglycosylation products. We hypothesize that ILs might induce conformational changes in the enzyme, which would modify the enzymatic activity and regioselectivity. These structural modifications were confirmed in the secondary and tertiary structures of the protein by circular dichroism and fluorescence studies, respectively. Molecular modeling confirms this hypothesis and shows that the enzyme becomes more flexible in an IL–water mixture and that it allows stabilization of the GlcNAc molecule in the active centre of the enzyme, in order to develop a new product according to the original regioselectivity of the reaction.
Introduction
The use of enzymes and whole cells for catalysis in the chemical industry is of great interest, as environmentally friendly alternative syntheses are able, in many cases, to compete economically with well-established chemical processes.1,2 The combination of biocatalysts with the appropriate solvents and/or type of reactor is key to the successful construction of a bioprocess. As many enzymes can catalyze reactions in organic solvents, there is much interest on environmental grounds in the use of ILs as (co)solvents to create novel reaction media for biocatalytic processes. ILs have emerged as a potentially attractive recyclable alternative to environmentally harmful organic solvents;3 they are not intrinsically green solvents, but careful design in the synthesis of ILs is required to reduce their toxicity.4,5
ILs are composed entirely of ions, generally consisting of organic cations and either organic or inorganic anions, and they have attracted extensive attention due to their special properties; they are liquids at room temperature,6 thermally stable,7 highly polar,8 relatively inert,9 have negligible vapour pressure,10 non-flammablility,11 good ionic conductivity,12 low melting temperature13 and wide electrochemical windows.14 So far, ILs have been used as solvents in various reactions catalyzed by enzymes, such as lipases,15 proteases,16 oxidoreductases,17 peroxidases,18 and whole cells.19 Nevertheless, studies of the use of ILs in transglycosylation catalyzed by glycosidases are scarce.20,21
The use of enzymes in ILs has proved to have many advantages, such as high conversion rates, high enantioselectivity and good recoverability and recyclability.22 These properties must be fully understood before enzyme technology can be applied in industrial chemical processes. Several authors have explained this behaviour in terms of enzyme–IL molecular interactions, but to our knowledge there are no reports quantifying such interactions. At the same time, surface plasmon resonance (SPR) has been developed as an analytical method to measure the interactions. SPR allows us to study the interactions between a ligand immobilized on a sensor chip and an analyte in solution that flows across the chip surface.23 Spectrophotometry techniques such as circular dichroism and fluorescence emission are two specific methods to analyze conformational changes in the secondary and tertiary structures of proteins.24–26 These approaches could be used to understand the effects of ILs on protein structure.
Thermus thermophilus HB27 is an extremely thermophilic bacteria with decoded genoma;27 its TTP0042 gene encodes a β-galactosidase that can catalyze the synthesis of disaccharides with β[1→3] glycosidic linkages.28–30 Actually, the synthetic activities of this β-galactosidase from cellobiose-induced cell extracts, and of the purified his6tag enzyme, were shown to catalyze the production of N-acetyl-D-lactosamine (Galβ[1→4]GlcNAc),31 with some amount of substrate self condensation (Gal-β[1→3]Gal-β-pNP) (Scheme 1).31,32
![Synthesis of Galβ[1→4]GlcNAc catalyzed by T. thermophilus β-galactosidase.](/image/article/2012/RA/c2ra20618h/c2ra20618h-s1.gif) |
| Scheme 1 Synthesis of Galβ[1→4]GlcNAc catalyzed by T. thermophilus β-galactosidase. | |
In this paper, we have studied for the first time the influence of ILs as co-solvents in the enzymatic synthesis mediated by a thermostable β-galactosidase from Thermus thermophilus HB27 (TTP0042). To understand the effect of these liquids over the synthetic behavior of the enzyme, we performed a molecular interaction study using SPR, a conformational study by circular dichroism and fluorescence techniques between TTP0042 and ILs, and finally we confirmed these interactions by molecular modeling.
Results and discussion
In a recent work we have demonstrated that TTP0042 is the main β-galactosidase from Thermus thermophilus HB27 that can catalyze the synthesis of N-acetyl-D-lactosamine (LacNAc or Galβ[1→4]GlcNAc), with some amount of donor self condensation (Gal-β[1→3]Gal-β-pNP and Gal-β[1→6]Gal-β-pNP) (Scheme 1).31 In the present work, we have extended the use of this enzyme to the synthesis of carbohydrates in the presence of different ILs. Tables 1 and 2 show the results obtained using cell extracts and pure enzyme as catalysts, using four different ILs (1-butyl-3-methylimidazolium hexafluorophosphate ([Bmim][PF6]), 1-octyl-3-methylimidazolium hexafluorophosphate ([Omim][PF6]), cocosalkyl pentaethoxy methyl ammonium methosulfate ([CPMA][MS]) and trioctylmethylammonium bistriflimide ([Troma][NTf2])) as co-solvents (Scheme 2).
Table 1 Synthesis of Gal-β[1→4]-GlcNAc (% yields) using induced cell extracts from HB27 as the catalyst
Media |
Gal-β[1→3]-Gal-β-pNP |
Gal-β[1→6]-Gal-β-pNP |
Galactose |
Gal-β[1→4]-GlcNAc |
Gal-β[1→6]-GlcNAc |
Buffer |
40 |
17 |
– |
41 |
2 |
[Bmim][PF6] |
– |
11 |
4 |
63 |
22 |
[Omim][PF6] |
– |
– |
– |
71 |
29 |
[CPMA] [MS] |
– |
– |
– |
82 |
18 |
[Troma][NTf2] |
13 |
14 |
– |
70 |
3 |
Table 2 Synthesis of Gal-β[1→4]-GlcNAc (% yields) using T. thermophilus β-galactosidase (TTP0042) as the catalyst
Media |
Gal-β[1→3]-Gal-β-pNP |
Gal-β[1→6]-Gal-β-pNP |
Galactose |
Gal-β[1→4]-GlcNAc |
Gal-β[1→6]-GlcNAc |
Buffer |
54 |
6 |
3 |
34 |
3 |
[Bmim][PF6] |
– |
4 |
19 |
69 |
8 |
[Omim][PF6] |
– |
8 |
5 |
79 |
8 |
[CPMA] [MS] |
– |
2 |
33 |
10 |
55 |
[Troma][NTf2] |
1 |
11 |
4 |
77 |
7 |
As can be seen, important changes in the enzyme regioselectivity were observed with both catalysts, compared to the usual all-water buffered media. All the ILs employed shifted the regioselectivity of this reaction towards a clear reduction, and even total disappearance, of self condensation products, with the concomitant enhancement of the yields of both the β[1→4] and β[1→6] disaccharides. Comparatively, the best results were obtained for [Bmim][PF6], [Omim][PF6] and [Troma][NTf2]. They show the advantage of not being miscible with water, whereas [CPMA][MS] is fully miscible. This water immiscibility makes them good candidates to be used in scaled up reactions, because it will allow solvent recovery from the reaction media, i.e. by simple centrifugation.
Firstly, it is noticeable how important the decrease of self-condensation products is (Gal-β[1→3]Gal-β-pNP and Gal-β[1→6]Gal-β-pNP), observed when ILs are used as co-solvents, in comparison with reactions performed in all-water buffer media. Secondly, when [Bmim][PF6], [Omim][PF6] and [Troma][NTf2] were employed, the reaction equilibrium was modified towards Galβ[1→4]GlcNAc synthesis. Thirdly, [CPMA][MS] improves Galβ[1→6]GlcNAc synthesis, while the same IL does not produce such an effect for reactions catalyzed by HB27 cell extracts and pure enzyme (Table 1 and Table 2).
Ionic liquid–enzyme molecular interactions by SPR
The influence that ILs exert on the activity and regioselectivity of this enzyme might be due more to direct interactions with the enzyme than to any other effect at the level of the substrate. To explore this possibility, we have used SPR to monitor interactions between the ILs and the enzyme. For this, the solubility of the ILs in water was investigated. It was found that the maximum solubility in water of the different ILs tested was: 33.5 Mm for [Bmim][PF6] (0.98%), 5.94 Mm for [Omim][PF6] (0.2%), 3.86 Mm for [Troma][NTf2] (0.25%) and 600 Mm for [CPMA][MS] (31%). Initially, we selected two ILs ([Bmim][PF6] and [Omim][PF6]), because they provided important results in disaccharide synthesis. Unfortunately they are slightly soluble in water. Both ILs were prepared in buffer solutions until their maximum solubility in water was reached.
Then, the β-galactosidase TTP0042 from Thermus thermophilus was covalently attached to a sensor chip and ILs [Bmim][PF6] and [Omim][PF6] were injected over the surface at various concentrations. The response was monitored as a function of time, to permit calculation of the rate constants. Due to the difficulties in the determination of kinetic rate constants, the concentration dependence of steady-state values (Req) was used to estimate apparent affinity (KD values). The apparent KD values obtained for [Bmim][PF6] and [Omim][PF6] were 16.5 mM and 8.73 mM respectively (see ESI† for details). These experiments were carried out at different concentrations from the enzymatic reaction due to solubility problems.
We next injected several high concentrations of [CPMA][MS] (10%) onto the immobilized enzyme sensor surface to examine binding under the same experimental conditions as the enzymatic reactions. This IL exhibited a strong nonspecific binding between the control surface and the IL. The response observed in the control cell was higher than in the cell where the enzymes were immobilized (data not shown).
The SPR interaction study described above shows that both ILs bind to the enzyme through non-specific interactions, characterized by very fast kinetics and millimolar affinity. Based on these data, we hypothesize that [Bmim][PF6] and [Omim][PF6] in the reaction media might induce conformational changes in the enzyme that would result in increased enzymatic activity and change in regioselectivity.
Effect of concentration on enzyme activity
One of the drawbacks of the molecular interaction measurements performed is the low solubility in water of some of the ILs used in this study. Both liquids tested in the SPR, [Bmim][PF6] and [Omim][PF6], displayed a limited solubility in water, making it difficult to achieve saturation of the enzyme. The same problem would happen if [Troma][NTf2] was used. One of the alternatives to consider in this case is the effect of increasing the concentrations of ionic liquid in the media. Based on this, we decided to repeat the transglycosylation reactions under different concentrations of IL: 0%, 5%, 10%, 20%, 30% (Fig. 1).
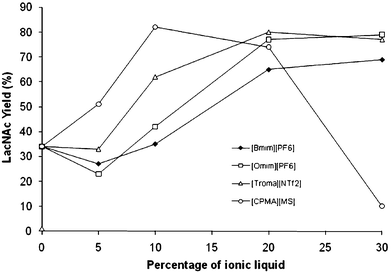 |
| Fig. 1 Effect of concentration of ILs on the enzymatic synthesis of LacNAc using TTP0042 as a catalyst. | |
According to the results, the effect of ILs on enzyme activity in transglycosylation reactions seems to be related to their concentration. If the concentration affects the enzyme behavior in spite of their solubility, this phenomenon could be related to the interface between water and the IL. During reactions the magnetic stirring disrupted the IL's surface, allowing the formation of small IL particles, and this effect may be enhanced at higher concentrations of ionic liquids, producing higher interface areas, improving their effects over the enzyme behaviour and also improving the synthesis.
Most of the ILs show a maximum synthesis of LacNAc when the reaction is performed at 30% IL, but when lower concentrations are used, the effects are not visible. At 5% IL the effect is almost null, this confirms that the molecular interaction, measured by SPR, between the enzyme and the IL is a very low interaction and may be improved by increasing the concentration of the IL. In this case, the effect of the interaction will only be in the buffer–IL interphase. Particular results were seen using [CPMA][MS] as the co-solvent. The effect is very significant at 5% IL and reaches a maximum activity at 10%. Then, the synthesis shifts from the β[1→4] disaccharide to the β[1→6]. At 30% [CPMA][MS] the enzyme produces 55% of the β[1→6] isomer and only 10% of the β[1→4] isomer.
Conformational effects of IL on enzyme structure measured by circular dichroism
Considering that the enzyme is affected by the concentration of the IL, we explore the possibility of studying the effects of conformational changes on the enzyme structure, using circular dichroism (CD) at higher concentrations of these substances. First, we selected the water-soluble IL [CPMA][MS], then we checked the absorbance of that liquid in the UV range (260–196 nm). At 10% m/v, it was not possible to measure because the liquid signal makes too much noise in the baseline (data not shown), but we were able to measure up to 5% m/v of this liquid. This is a good concentration, because the enzyme synthesized more than 50% LacNAc under these conditions (see Fig. 1).
The far UV CD spectrum of TTP0042 was recorded with the enzyme in buffer solution. Then, small amounts of [CPMA][MS] were added to the cuvette and several CD spectra were taken (Fig. 2). As a result, the enzyme presented an α-helix as the main structure and two valleys at 208 and 218 nm were identified. The addition of [CPMA][MS] did not affect the position of either valley, but changed the magnitude of the mean residue ellipticity (MRE, Fig. 3). A great loss of the α-helix structure of the enzyme was found when low concentrations of IL (0.08%, 0.15% and 0.5% m/v) were prepared. The enzyme retained the general structure, but the signal for the α-helix decreased.
![Effect of [CPMA][MS] concentration over negative ellipticity bands at 208 nm and 218 nm.](/image/article/2012/RA/c2ra20618h/c2ra20618h-f3.gif) |
| Fig. 3 Effect of [CPMA][MS] concentration over negative ellipticity bands at 208 nm and 218 nm. | |
At higher concentrations (from 1.5% to 5% m/v) the enzyme increased its α-helix structure and reached more stable forms. CD values at 3.0% and 5.0% showed an identical spectrum, which could mean a steady state of the enzyme structure in presence of this IL. However, the protein did not recover its original secondary structure. This conformational change when the enzyme is dissolved in the presence of the IL could explain the result obtained in the transglycosylation reactions in the presence of ILs.
Fluorescence study
If the enzyme structure is affected by the presence of the IL, this effect could also be seen in its tertiary structure. For this, we used [CPMA][MS] to perform a fluorescence study. The enzyme was first dissolved in 10 mM sodium phosphate buffer (pH 6.00), to measure a native emission spectrum, then some aliquots of IL were added. As a result, we saw a red shift of the maximum peak; the enzyme in the buffer medium displays a maximum emission wavelength of 334 nm; when the enzyme is placed in 5% m/v IL, the maximum fluorescence emission is shifted to 346 nm (Fig. 4).
The result suggests a modification of the chemical environment for the main fluorescence residue: tryptophan.33 Tryptophan is highly hydrophobic and its location is related to the internal regions of the enzyme. This suggests that the IL affects every region of the protein, which in this case, also means a modification of the tertiary structure of the enzyme and points out the ionic liquid as being responsible for the enzyme structure modification. Conformational changes in protein structure found in CD and fluorescence studies did not affect the enzyme activity. As proof, we analyzed the thermal stability under the reaction conditions (data not shown). As a result, the enzyme displays a very similar deactivation rate in the buffer and [CPMA][MS] media, confirming no denaturalization due to this IL. These data lead us to conclude that modifications of the enzyme secondary and tertiary structures are the most important effects seen in the enzyme behaviour in this study, and also help to explain the cause of the great regioselectivity change found during the transglycosylation reactions.
Molecular modeling
In order to explain the kind of conformational change that might occur due to the interaction and its nature, we have used molecular modelling. With this aim, two different types of simulations were carried out. First, short-time simulations, in the picosecond range, with a very small time step were performed to analyze the kinetics and thermodynamics of the hydrogen bonds between the solvents and the enzymes. Second, longer-time simulations, in the nanosecond range, were used to check a possible conformational change of the system due to the interaction between the IL and the enzyme. For both cases, molecular dynamics simulations were based on the use of a structural model based on the β-glucosidase of T. thermophilus HB8 (PDB code 1UG6). This enzyme only differs from that of β-galactosidase TTP0042v of T. thermophilus HB27 in a single external residue. This change was included in a structural model that was subjected to the molecular dynamics experiments.
The enzyme was simulated with three solvent mixtures ([Bmim][PF6]–water, [Omim][PF6]–water and water) with the aim of getting an insight into the possible structural changes involved in the interactions observed in the SPR experiments. The root mean squared deviation (RMSD) of the carbon alpha atoms for the three assay systems (Fig. 5) during the simulation confirmed the stability of the systems in those media.
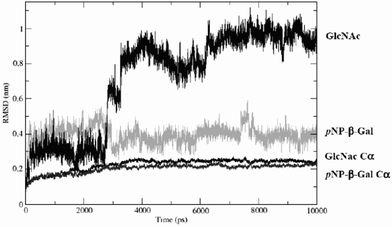 |
| Fig. 5 Comparative RMSD of pNP-β-Gal and GlcNAc in water. RMSD of Cα with the first ligand and with the second one. | |
A slight difference was found between IL-containing systems and water. In order to explain the ligand selectivity towards the different substrates for which the enzyme has an affinity, glycosil–enzyme complexes were simulated by automatic docking of a pNP-β-Gal molecule into the active centre, and further formation of a covalent link between the Glu-164 position of the enzyme and the best position of the galactose molecule. This structure was again used to dock substrates pNP-β-Gal and GlcNAc.
Finally, these complexes were solvated with the three solvent systems ([Bmim][PF6]–water, [Omim][PF6]–water and water) in the case of GlcNAc, and with water only in the case of pNP-β-Gal. The trajectories generated were analyzed by measuring the RMSD of the ligand and by principal component analysis (PCA), to try to identify large amplitude movements that could shed some light on the experimental behaviour of the enzyme. These analyses revealed that simulations were able to reproduce the experimental selectivity of the enzyme.
As can be seen in Fig. 6, the RMSD of GlcNAc in the water-only system raised movements to more than 10 Å, before re-stabilizing, which resulted in movement of the ligand from the active centre to the solvent after 2.7 ns. In contrast, pNP-β-Gal remained stable in the active centre during the whole simulation period. A possible explanation of these differences between different ligands arose from the PCA results. The largest amplitude movement corresponds to a unique displacement of the loop between residues Arg-285 and Pro-299 from the active center of the protein when GlcNAc was used in the water system (Fig. 6).
This kind of high range movement was not observed under the other conditions simulated. Actually, a small displacement of this same region in the opposite direction was found in the ligand-free systems when ILs were present, thus supporting the opening of the active centre under these conditions.
Simulations also suggest that this opening of the active centre could be triggered by certain kinds of ligands under normal conditions, as is the case for GlcNAc, for which the increase in the RMSD of the ligand appears to be correlated with the displacement of this region of the protein. This trigger mechanism could be based on the ability of the ligands to fill the pocket (Fig. 7).
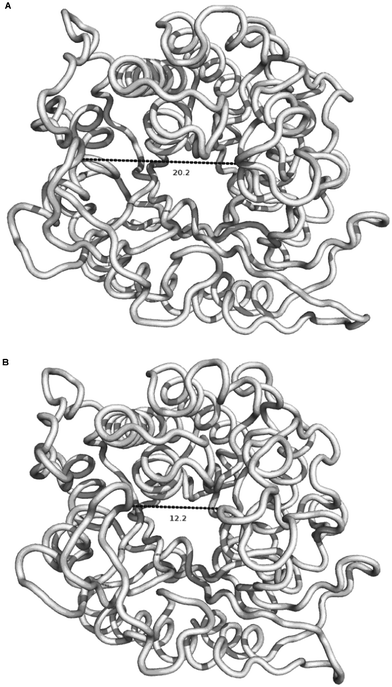 |
| Fig. 7 Displacement of residues between Arg-285 and Pro-299 from the active centre in the GlcNAc–water simulation. | |
For example, in the case of pNP-β-Gal, the pNP group interacts with the zone near the loop, in some way preventing the displacement of these residues into the cavity and the ultimate displacement of the ligand from the active center. On the other hand, the mixtures of IL and water could modify this behaviour, stabilizing the GlcNAc molecule in the active center and allowing the reaction to occur. As other authors34 have pointed out, ILs can kinetically trap proteins which may be the cause of a change in the flexibility of this loop next to the active center, explaining the different behaviours of these solvents.
To characterize the interaction between the solvents and the protein, we have analyzed the kinetic and thermodynamic properties of the hydrogen bonds (HBs) between them. HBs were defined based on a geometric criteria of a donor–acceptor distance of 3.5 Å and an donor–hydrogen–acceptor angle of 30°. HB properties were calculated as described in the van der Spoel et al. method35 using a modified version of the g_hbond tool of the GROMACS suite to account for the acceptor character of the fluor atoms in the solvent.
In this analysis technique, hydrogen bond breaking is interpreted as an Eyring process, for which the Gibbs energy of activation ΔG can be determined from the HB lifetime. Short trajectories of 40 ps were employed with a time step of 0.5 fs with no constraints, saving the coordinates every 2 fs, providing high time resolution to determine the lifetime of the hydrogen bonds and their activation energies.36
A comparison of the values for water, [Omim][PF6] and [Bmim][PF6] is presented in Table 3. As can be seen, in both ILs the required energy to break a hydrogen bond is higher than in the case of water. However, the calculated KD derived from this interaction is in the mM range (Table 3). The KD observed is similar to values obtained by SPR.
Table 3 Hydrogen bond lifetimes and activation energies
|
Lifetime (ps) |
ΔG (kJ mol−1) |
KD (mM) |
Water–protein |
7.462 |
9.510 |
− |
[Bmin][PF6] |
12.653 |
10.819 |
12.66 |
[Omin][PF6] |
9.977 |
10.230 |
12.06 |
Conclusions
In this work, we provide a rational explanation for the positive effect of ILs on the synthetic activity of the β-galactosidase from Thermus themophilus (TTP0042). First, we found an interesting change from the classic regioselectivity for this enzyme, reducing self-condensation of the donor and increasing disaccharide production in the presence of 30% IL. This effect was also reproducible by using different ILs. The apparent molecular interaction was measured using SPR. As a result, the apparent KD value obtained for these liquids was in the millimolar range and could mean that they bind to the enzyme through non-specific interactions. The concentration of the ILs also affects the reaction results, which may be related to the proportional interface generated by magnetic stirring. Then CD and fluorescence measurements found a modification of the enzyme secondary and tertiary structures, respectively, due to the presence of [CPMA][MS]. The molecular modeling study indicates that the enzyme becomes more flexible in the IL–water mixture and it allows stabilization of the GlcNAc molecule in the active center of the enzyme, developing a new product according to the original regioselectivity of the reaction. Our results indicate that the combination of these techniques and molecular modeling are good strategies for unraveling the molecular basis of other IL–enzyme interactions and explain changes in enzymatic reactions using ILs as co-solvents.
Experimental section
Materials and reagents
ILs: 1-butyl-3-methylimidazolium hexafluorophosphate ([Bmim][PF6], 99% purity), 1-octyl-3-methylimidazolium hexafluorophosphate ([Omim][PF6], 99% purity) and cocosalkyl pentaethoxy methyl ammonium methosulfate ([CPMA] [MS]) were obtained from Solvent Innovation GmbH (Germany). The IL trioctylmethylammonium bistriflimide ([Troma][NTf2]) was synthesized as described previously.37 Bovine serum albumin (BSA), p-nitrophenol (pNP), p-nitrophenyl-β-D-galactopiranosyde (pNP-β-Gal), N-acetyl-D-glucosamine (GlcNAc) and analytical standards of monosaccharides for HPLC were purchased from Sigma-Aldrich. All other chemicals were of analytical grade.
Bacterial strains, enzyme and media
Thermus thermophilus HB27 was cultivated in the TB medium38 diluted by ¼, by incubation at 70 °C under aerobic conditions, in order to induce β-galactosidase activity in cell extracts.31 Cells were disrupted by sonication and unbroken cells and insoluble debris were eliminated by centrifugation (14
000g for 15 min at 4 °C). Recombinant β-galactosidase from T. Thermophilus HB27 (TTP0042 his6tag) was cloned in Escherichia coli using pET28b+ vector (Novagen) as reported previously.31E. coli cultures were grown aerobically at 37 °C in LB with kanamycin (30 mg L−1) and induced with IPTG (isopropyl-β-D-thiogalactopyranoside, 0.5 mM) at 37 °C for 6 h. Cell extracts were prepared as above and then incubated at 70 °C for 40 min to denature most of the E. coli protein, which were further removed by centrifugation at 14
000g for 20 min at 4 °C. The solution obtained was passed through a Ni2+–agarose column (3 mL) according to the manufacturer's protocol (BioRad). Fractions were monitored by absorbance at 280 nm, pooled, concentrated and then desalted in an Amicon ultra centrifuge filter (Millipore). The purification process was followed by SDS-PAGE. Protein quantification was done by the Bradford method39 using bovine serum albumin as standard. Enzyme activity was measured with pNP-β-Gal as the substrate using the discontinuous method as described previously.31 One enzyme unit (U), was defined as the amount of protein that hydrolyzes 1.0 μmol substrate per minute.
Transglycosylation reactions
pNP-β-Gal (51.2 mg) and GlcNAc (183.0 mg) were dissolved in 1.00 mL of the buffer–ionic liquid mixture (30% v/v ionic liquid as final concentration) and pre-warmed to the reaction temperature (80 °C for cell extracts and 65 °C for the recombinant enzyme). The reaction was started by addition of the biocatalyst to the mixture: 20 U of lyophilized cell extract or 1.80 U of recombinant enzyme. Aliquots (50 μL) were withdrawn from the reaction media after mixing in a vortex; the aliquot composition was representative of the sample, including the buffer and the ionic liquid. The reaction was quenched by addition of 450 μL pure methanol per 50 μL of aliquot. Under these conditions, methanol dissolves ionic liquids, making a monophasic system in order to analyze by HPLC. Then, samples were conserved immediately at −20 °C. Analytical determination of the products was performed by HPLC using a NH2P50-4E amino column (Asahipak, Japan) eluted at 0.80 mL min−1, 80% acetonitrile and 20% water, using three detectors: ELSD (light scattering), UV-vis and CD (circular dichroism) at 317 nm. Products were separated on a charcoal/celite column, and structural determination was done by 1H-NMR and 13C-NMR (D2O, 700 MHz) on a Bruker Avance 700. Spectra were identical as previously reported.31,40,41
SPR studies
Assays were carried out at 25 °C and 5 μL min−1 on a Biacore 3000 (GE Healthcare, Uppsala, Sweden) using a commercial CM-5 sensor chip. TTP0042 was covalently immobilized on the surface chip (see ESI† for details). Soluble ILs were flown using 4.18, 8.36, 16.7, 25.1 and 33.5 mM solutions of [Bmim][PF6] and 1.19, 2.38, 3.56, 4.75, 5.94 and 7.13 mM solutions of [Omim][PF6]. The interaction was measured, and steady state affinity plots were created for both ILs using these values. The maximum solubility of the IL in buffer solution was reached after shaking the buffer–IL mixture for 10 min at 25 °C in a sonic-bath and then mixing in a vortex.
Effect of IL concentration on enzyme activity
Transglycosylation reactions were done as described above, using 0%, 5%, 10%, 20% and 30% of [Bmim][PF6], [Omim][PF6], [CPMA][MS] and [Troma][NTf2]. Disaccharide yields (LacNAc) were plotted against IL concentration.
Circular dichroism measurements
A Jasco-710 dichrograph previously calibrated with d-10-camphorsulphonic acid was used to record the far-UV CD spectra of TTP0042. Measurements were performed in 0.1 cm cells at 25 °C. Five accumulations were acquired for each spectrum. The ellipticity was measured with a 2 nm bandwidth and a 2s response. The Mean Residue Ellipticity (MRE) was calculated as elsewhere,42 using a molecular weight of 50664.2 Da for TTP0042 (his6tag) and 450 residues. Initial protein concentration was 19.8 μM, in 10 mM sodium phosphate buffer at pH 6.00. Then, a CD spectrum was obtained for [CPMA][MS] (water dissolved) in order to determine the maximum concentration in which to perform the study. 5% m/v was determined as the maximum amount of IL to measure the CD spectra. Then several additions of IL (20% m/v) were made in order to reach the following final concentrations of [CPMA][MS] in the cuvette: 0.08%, 0.15%, 0.50%, 1.50%, 3.0% and 5.0% m/v. CD spectra were recorded for those enzyme–IL mixtures. MRE was plotted against wavelength.
Fluorescence study
Fluorescence emission spectra were obtained on a PTI modular spectrometer. 295 nm was set as the excitation wavelength because it is highly selective for tryptophan, and emission was acquired from 310 to 500 nm. The excitation and emission bandwidths were adjusted to 2 nm, measurements were done in a 0.20 cm pathlength cell at 25 °C with a thermoelectric temperature regulator TLC 50. Spectra analysis was done using Felix 32 software. An initial spectrum was taken for TTP0042 19.8 μM, in 10 mM sodium phosphate buffer at pH 6.00. Then, several additions of [CPMA][MS] (water dissolved) were performed in the cuvette. Emission spectra were recorded for those enzyme–IL mixtures. Fluorescence was plotted against wavelength.
The X-ray solved structure of the β-glucosidase of T. thermophilus HB8 (PDB code 1UG6) was used as the initial coordinates for the protein. The mutation Y320H present in the HB27 strand was modelled using the CPH model server.43 All the ligands were geometrically optimized at B3LYP/6-31G** level of theory using the Massively Parallel Quantum Chemistry package.44
Molecular docking and parametrization
The active center of the enzyme was identified as the only cavity available in the structure with two glutamic acid residues, Glu-164 and Glu-338, at the bottom of the pocket. The docking was carried out with Autodock45 using the Lamarkian genetic algorithm, the default parameters and a grid of 20 Å around the two glutamic residues. Ligand parameters were obtained from the PRODRG server.46 [Omim][PF6] and [Bmim][PF6] molecules were optimized with DFT theory at the level B3LYP/6-31G**. Bond lengths and angles and charges were extracted from DFT calculations and the rest of the parameters were derived from the parameters of the force field.
To validate the solvent parameters, simulations of 216 ionic pairs of each IL were set up with the following protocol: 216 cations with 216 anions of each species were randomly disposed in boxes and equilibrated over 300 ps at 298 K and 1 bar, then 10 ns production simulations were carried out. Density and enthalpy of vaporization were measured and compared with the experimental values. In both case the values were in agreement with the experimental data available (Table 4) and with the results of other force fields for these kinds of molecules.51
Table 4 Experimental and theoretical solvent parameters for [Omim][PF6] and [Bmim][PF6]
IL |
ρ
calc (Kg m−3) |
ρ
exp (Kg m−3) |
ΔHvcalc (kcal mol−1) |
ΔHvexp (kcal mol−1) |
[Omim][PF6] |
1382 |
136847 |
189 |
19148 |
[Bmim][PF6] |
1290 |
123549 |
261 |
16950 |
Molecular dynamics
Simulations were performed using the parameters of the GROMOS 96 43a1 force field implemented in the GROMACS52 software package v4.0.7. Solvents with no parameters available for the force field and ligands were parametrized as described before. All the molecular dynamics simulations were carried out with the same protocol. The complexes resulting from the docking were solvated in boxes of mixtures of ionic liquids and water to reproduce the experimental conditions, fulfilling the requirement of 12 Å from any atom of the enzyme and the edge of the box. Energy minimizations of 500 steps of steepest descent were followed by 2500 steps of Polak–Ribière conjugate gradients. Then, an equilibration run of 300 ps NVT conditions (338 K) followed by 300 ps NPT conditions (338 K, 1 bar) were carried out. Finally, 10 ns simulations were performed and analyzed using principal component analysis (PCA).53
Acknowledgements
This work has been supported by four research projects, three of the MICINN (“Ministerio de Ciencia e Innovación”), CTQ2009-11801, BIO2010-18875 and MAT 2008-02542; and one of the Complutense University GR35/10-A-950247. Manuel Sandoval thanks a PhD fellowship granted by Universidad Nacional de Costa Rica. We are grateful to Prof. Dr Francisco García-Blanco for critical reading of the manuscript.
References
- H. E. Schoemaker, D. Mink and M. G. Wubbolts, Science, 2003, 299, 1694–1697 CrossRef CAS.
- M. J. Hernaiz, A. R. Alcántara, J. I. García and J. V. Sinisterra, Chem.–Eur. J., 2010, 16, 9422–9437 CrossRef CAS.
- P. Domínguez de Maria, Angew. Chem., Int. Ed., 2008, 47, 6960–6968 CrossRef.
- M. Matzke, S. Stolte, J. Arning, U. Uebers and J. Filser, Green Chem., 2008, 10, 584–591 RSC.
-
N. V. Plechkova and K. R. Seldon, in Methods and Reagents for Green Chemistry, ed. P. Tundo, A. Perosa and F. Zecchini, Wiley, New York, 2007, pp. 105–130 Search PubMed.
- M. Galínski, A. Lewandowski and I. Stepniak, Electrochim. Acta, 2006, 51, 5567–5580 CrossRef.
- M. Kosmulski, J. Gustafsson and J. B. Rosenholm, Thermochim. Acta, 2004, 412, 47–53 CrossRef CAS.
- S. V. Dzyuba and R. A. Bartsch, Tetrahedron Lett., 2002, 43, 4657–4659 CrossRef CAS.
- C. Chiappe and D. Pieraccini, J. Phys. Org. Chem., 2005, 18, 275–297 CrossRef CAS.
- M. J. Earle, J. M. S. S. Esperanca, M. A. Gilea, J. N. Canongia Lopes, L. P. N. Rebelo, J. W. Magee, K. R. Seddon and J. A. Widegren, Nature, 2006, 439, 831–834 CrossRef CAS.
- D. M. Fox, W. H. Awad, J. W. Gilman, P. H. Maupin, H. C. De Long and P. C. Trulove, Green Chem., 2003, 5, 724–727 RSC.
- S. Tsuzuki, H. Tokuda, K. Hayamizu and M. Watanabe, J. Phys. Chem. B, 2005, 109, 16474–16481 CrossRef CAS.
- P. Wasserscheid and W. Keim, Angew. Chem., Int. Ed., 2000, 39, 3772–3789 CrossRef CAS.
- R. Hagiwara and Y. Ito, J. Fluorine Chem., 2000, 105, 221–227 CrossRef CAS.
- T. De Diego, P. Lozano, M. A. Abad, K. Steffensky, M. Vaultier and J. L. Iborra, J. Biotechnol., 2009, 140, 234–241 CrossRef CAS.
- K. Sangeetha, V. B. Morris and T. Emilia Abraham, Appl. Catal., A, 2008, 341, 168–173 CrossRef CAS.
- W. Hussain, D. J. Pollard, M. Truppo and G. J. Lye, J. Mol. Catal. B: Enzym., 2008, 55, 19–29 CrossRef CAS.
- D. Z. B. Eker, G. Zhu, R. J. Linhardt and J. S. Dordick, J. Mol. Catal. B: Enzym., 2009, 59, 177–184 CrossRef.
- M. M. Musa, K. I. Ziegelmann-Fjeld, C. Vieille and R. S. Phillips, Org. Biomol. Chem., 2008, 6, 887–892 CAS.
- N. Kaftzik, P. Wasserscheid and U. Kragl, Org. Process Res. Dev., 2002, 6, 553–557 CrossRef CAS.
- M. Lang, T. Kamrat and B. Nidetzky, Biotechnol. Bioeng., 2006, 95, 1093–1100 CrossRef CAS.
- J. P. Mann, A. McCluskey and R. Atkin, Green Chem., 2009, 11, 785–792 RSC.
- D. G. Myszka, Curr. Opin. Biotechnol., 1997, 8, 50–57 CrossRef CAS.
- L. Whitmore and B. A. Wallace, Biopolymers, 2008, 89, 392–400 CrossRef CAS.
- S. R. Tello-Solis, J. Jimenez-Guzman, C. Sarabia-Leos, L. Gomez-Ruiz, A. E. Cruz-Guerrero, G. M. Rodriguez-Serrano and M. Garcia-Garibay, J. Agric. Food Chem., 2005, 53, 10200–10204 CrossRef CAS.
- H. Herberhold, S. Marchal, R. Lange, C. H. Scheyhing, R. F. Vogel and R. Winter, J. Mol. Biol., 2003, 330, 1153–1164 CrossRef CAS.
- A. Henne, H. Bruggemann, C. Raasch, A. Wiezer, T. Hartsch, H. Liesegang, A. Johann, T. Lienard, O. Gohl, R. Martinez-Arias, C. Jacobi, V. Starkuviene, S. Schlenczeck, S. Dencker, R. Huber, H.-P. Klenk, W. Kramer, R. Merkl, G. Gottschalk and H.-J. Fritz, Nat. Biotechnol., 2004, 22, 547–553 CrossRef CAS.
- M. Dion, L. Fourage, J.-N. Hallet and B. Colas, Glycoconjugate J., 1999, 16, 27–37 CrossRef CAS.
- V. Chiffoleau-Giraud, P. Spangenberg, M. Dion and C. Rabiller, Eur. J. Org. Chem., 1999, 757–763 CrossRef CAS.
- L. Fourage and B. Colas, Appl. Microbiol. Biotechnol., 2001, 56, 406–410 CrossRef CAS.
- M. Sandoval, E. Ferreras, M. Pérez-Sánchez, J. Berenguer, J. V. Sinisterra and M. J. Hernaiz, J. Mol. Catal. B: Enzym., 2012, 74, 162–169 CrossRef CAS.
- V. Tran, L. Hoffmann, C. Rabiller, C. Tellier and M. Dion, Protein Eng., Des. Sel., 2010, 23, 43–49 CrossRef CAS.
- J. T. Vivian and P. R. Callis, Biophys. J., 2001, 80, 2093–2109 CrossRef CAS.
- P. J. V. M. D. van der Spoel, P. Larsson and N. Tìmneanu, J. Phys. Chem. B, 2006, 110, 4393–4398 CrossRef.
- F. L. W. Zhao, B. Heggen, S. Zahn, B. Kirchner, S. Balasubramanian and F. Muller-Plathe, J. Am. Chem. Soc., 2009, 131, 15825–15833 CrossRef.
- C. M. S. N. M. Micaêlo, J. Phys. Chem. B, 2008, 112, 2566–2572 CrossRef.
- P. Lozano, T. de Diego, J. P. Guegan, M. Vaultier and J. L. Iborra, Biotechnol. Bioeng., 2001, 75, 563–569 CrossRef CAS.
- S. Ramirez-Arcos, L.A. Fernandez-Herrero, I. Marin and J. Berenguer, J. Bacteriol., 1998, 180, 3137–3143 CAS.
- M. M. Bradford, Anal. Biochem., 1976, 72, 248–254 CrossRef CAS.
- M. Pérez-Sánchez, M. Sandoval, A. Cortés-Cabrera, H. García-Marín, J. V. Sinisterra, J. I. García and M. J. Hernaiz, Green Chem., 2011, 13, 2810–2817 RSC.
- N. Bridiau and T. Maugard, Biotechnol. Prog., 2011, 27, 386–394 CrossRef CAS.
- Z. G. Peng, K. Hidajat and M. S. Uddin, Colloids Surf., B, 2004, 33, 15–21 CrossRef CAS.
- M. Nielsen, C. Lundegaard, O. Lund and T. N. Petersen, Nucleic Acids Res., 2010, 38, W576–W581 CrossRef CAS.
-
C. L. Janssen, I. B. Nielsen, M. L. Leininger, E. F. Valeev and E. T. Seidl, Sandia National Laboratories: Livermore, CA, USA, 2004.
- G. M. Morris, R. Huey, W. Lindstrom, M. F. Sanner, R. K. Belew, D. S. Goodsell and A. J. Olson, J. Comput. Chem., 2009, 30, 2785–2791 CrossRef CAS.
- A. Schuttelkopf and D. van Aalten, Acta Crystallogr., Sect. C: Cryst. Struct. Commun., 2004, 60, 1355–1363 Search PubMed.
- A. Kumar, J. Solution Chem., 2008, 37, 203–214 CrossRef CAS.
- K. Swiderski, A. McLean, C. Gordon and D. Vaughan, Chem. Commun., 2004, 2004, 2178–2179 RSC.
- A. Pereiro and J. Legido, J. Chem. Thermodyn., 2007, 39, 1168–1175 CrossRef CAS.
- J. Armstrong, C. Hurst, R. Jones, P. Licence, K. Lovelock, C. Satterley and I. Villar-Garcia, Phys. Chem. Chem. Phys., 2007, 9, 982–990 RSC.
- Z. Liu, X. Wu and W. Wang, Phys. Chem. Chem. Phys., 2006, 8, 1096–1104 RSC.
- B. Hess, C. Kutzner, D. Van Der Spoel and E. Lindahl, J. Chem. Theory Comput., 2008, 4, 435–447 CrossRef CAS.
- A. Amadei, A. B. M. Linssen and H. J. C. Berendsen, Proteins: Struct., Funct., Genet., 1993, 17, 412–425 CrossRef CAS.
|
This journal is © The Royal Society of Chemistry 2012 |
Click here to see how this site uses Cookies. View our privacy policy here.