DOI:
10.1039/C2RA20444D
(Paper)
RSC Adv., 2012,
2, 5199-5204
Synthesis of superacid-modified poly(arylene ether sulfone)s via post-bromination†
Received
9th March 2012
, Accepted 13th March 2012
First published on 15th March 2012
Abstract
A versatile synthetic method of superacid-modified poly(arylene ether sulfone)s via post-bromination has been developed. Three kinds of high molecular weight poly(arylene ether sulfone)s, in which differences lie in the main chain structures, were synthesized and brominated. Careful control of the reaction conditions enabled selective and quantitative bromination of the polymers. The bromo groups were converted to superacid groups via Ullmann coupling reaction to obtain the title ionomers (FSPE-1a, 1b, and 1c). The chemical structure and the ion exchange capacity (IEC) of the FSPE-1s were characterized by 1H and 19F NMR spectra. Tough, flexible, and transparent membranes with IEC ranging from 0.87 to 1.09 meq g−1 were obtained by solution casting. The FSPE-1 membranes showed comparable properties (chemical stability, phase-separated morphology, water absorbability, and proton conductivity,) to those of our previous version of the superacid-modified poly(arylene ether sulfone) (FSPE) synthesized from brominated monomers (pre-bromination method). The advantages of the post-bromination method have been proven by significant improvement in the mechanical strength of the FSPE-1 membranes since it could provide superacid-modified aromatic ionomers with much higher molecular weight.
1. Introduction
Polymer electrolyte membrane fuel cells (PEMFCs) are regarded as a clean and efficient source of electric power. Polymer electrolyte membranes (PEMs) are one of the key materials of PEMFCs in terms of performance and production cost.1 Perfluorinated sulfonic acid (PFSA) ionomers, such as Nafion, have been mostly used as PEMs because of their high proton conductivity and durability. PFSA ionomers suffer from several issues such as rather high gas permeability, environmental incompatibility, and high production and processing cost.2 They show relatively low glass transition temperature (ca. 110 °C) due to the flexible aliphatic framework, which limits the operational temperature of PEMFCs. Therefore, there has been a great demand for alternative proton conductive PEMs that contain no or little fluorine atoms and are high temperature operable.3–5
Aromatic ionomers are one of the attractive candidates and have been extensively investigated in the last decade. Their advantages include ease of synthesis and chemical modification, good film forming capability, high glass transition temperatures, low gas permeability, and low production cost. A number of aromatic ionomers have been developed such as sulfonated poly(phenylene)s,6,7 poly(arylene ether ketone)s,8,9 poly(arylene ether sulfone)s,10,11 and polyimides.12–14 These ionomer membranes show high proton conductivity under well-hydrated conditions, however, the conductivity drops by several orders of magnitude when membranes are dry or insufficiently hydrated. The weaker acidity of the aromatic sulfonic acid groups compared to that of superacid groups (e.g., the pKa values of ArSO3H and perfluorosulfonic acid are ca. −1 and less than −6, respectively) is partly responsible.15 Therefore, the effective proton concentration is lower in the aromatic ionomer membranes under low humidity conditions. In addition, rigid polymer main chains often cause less-developed hydrophilic/hydrophobic phase separation, which results in dead-end ionic channels and low proton conductivity. Block copolymer structures have been claimed to promote hydrophilic/hydrophobic phase separation and interconnected ionic channels for higher proton conductivity.16,17
Recently, superacid-modified aromatic ionomers have been developed by several groups.18–21 In our previous work, we have demonstrated that poly(arylene ether)s containing pendant perfluorosulfonic acid groups showed more phase-separated morphology and higher proton conductivity than those of the poly(arylene ether)s with conventional sulfonic acid groups.22–24 The synthetic method required bromo- or iodo-substituted monomers as starting materials. The objective of the present research is to provide a versatile synthetic method for superacid-modified aromatic ionomers via a simple post-bromination. We have synthesized three kinds of superacid-modified poly(arylene ether sulfone)s, which differ in the bulky aromatic moieties used as scaffolds for bromo and superacid groups. Their properties as PEMs such as proton conductivity, water absorbability, oxidative and hydrolytic stability, mechanical strength, and morphology have been investigated in detail and compared with those of our previous superacid-modified poly(arylene ether sulfone)s prepared from the brominated monomers.
2. Experimental
2.1 Materials
9,9-Bis(4-hydroxyphenyl)fluorene (BHF) (98%, TCI), 9-fluorenone (98%, TCI), 2-phenylphenol (98%, TCI), toluene (99.5%, Kanto Chemical), 3-mercaptopropionic acid (Kanto Chemical), sulfuric acid (96%, Kanto Chemical), 4,4′-dihydroxytetraphenylmethane (DHPM) (98%, TCI), potassium carbonate (99.5%, Kanto Chemical), bromine (99.5%, Aldrich), chloroform (99.5%, dehydrated, Kanto Chemical), dichloromethane (99.5%, dehydrated, Kanto Chemical), and copper powders (99.8%, 50 and 100 nm in diameter, Aldrich) were used as received. Bis(4-fluorophenyl)sulfone (FPS) (99%, Acros Organics) was purified by crystallization from ethanol. N,N-Dimethylacetamide (DMAc) (99%, Kanto Chemical) was dried over 3Å-molecular sieves prior to use. Potassium 1,1,2,2-tetrafluoro-2-(1,1,2,2-tetrafluoro-2-iodoethoxy)ethanesulfonate (PSA-K) was prepared according to the previous report.18 Other chemicals were of commercially available grade and used as received.
A 100 mL three-neck round-bottomed flask equipped with a magnetic stirring bar and an N2 inlet was charged with 9-fluorenone (10.0 mmol, 1.80 g), 2-phenylphenol (40.0 mmol, 6.81 g), toluene (5.0 ml), sulfuric acid (0.3 mL), and 3-mercaptopropionic acid (0.8 μL). The mixture was stirred at 80 °C for 15 h under N2 atmosphere. The mixture was poured dropwise into 1 L of deionized water to precipitate a brown flaky solid. The crude product was washed with hot deionized water several times and purified by crystallization from acetone/toluene to obtain pure BHPF in 72% yield. 1H NMR (DMSO-d6, δ, ppm): 7.85 (2H, d), 7.46 (2H, d), 7.35 (2H, t), 7.32 (4H, d), 7.28 (4H, t), 7.26 (2H, s), 7.19 (2H, t), 6.95 (2H, d), 6.93 (2H, t), 6.80 (2H, d).
2.3 Synthesis of polymers (PE-1a, 1b and 1c)
The polymerization procedure for PE-1a has been described previously.10 Other polymers were synthesized in a similar manner. A typical procedure is as follows. A 200 mL three-neck round-bottomed flask equipped with a magnetic stirring bar, an N2 inlet, and an addition funnel, was charged with BHPF (10.0 mmol, 5.02 g), FPS (10.0 mmol, 2.54 g), potassium carbonate (25.0 mmol, 3.46 g), toluene (3.0 mL), and DMAc (30 mL). The mixture was stirred at room temperature for a few minutes and heated at 140 °C for 3 h and at 165 °C for 3h under N2 atmosphere. Then, 30 mL of DMAc was added to the mixture to lower the viscosity. The mixture was poured dropwise into 1 L of deionized water to precipitate a white fibrous solid. The crude product was washed with hot deionized water and methanol several times and purified by re-precipitation from chloroform/acetone. The resulting product was dried under vacuum at 60 °C for 15 h to obtain PE-1b in 71% yield. Using BHF instead of BHPF gave PE-1a in 74% yield. PE-1c was obtained using DHPM in 80% yield.
2.4 Bromination
A typical procedure is as follows. A 200 mL round-bottomed flask equipped with a magnetic stirring bar was charged with PE-1a, -1b, or -1c (2.0 mmol) and chloroform (150 mL). Br2 (40.0 mmol, 6.40 g) in a chloroform solution (50 mL) was added gradually to the mixture and reacted at room temperature for 20 h. The mixture was poured dropwise into 1 L of methanol to precipitate a fibrous solid. The crude product was washed with 0.1 M Na2SO3 aqueous solution and deionized water. The resulting product was dried under vacuum at 80 °C for 15 h to obtain the brominated polymer (BrPE-1a, 1b, or 1c).
2.5 Perfluorosulfonation
A typical procedure is as follows. A 100 mL three-neck round-bottomed flask equipped with a magnetic stirring bar, an N2 inlet, and an addition funnel was charged with BrPE-1a (0.5 mmol, 0.39 g), copper powder (6.5 mmol, 0.41 g), and DMAc (10 mL). The mixture was stirred at 120 °C for 20 h under N2 atmosphere. PSA-K (2.6 mmol, 1.20 g) in DMAc solution (2 mL), was added to the mixture and heated at 160 °C for 96 h. The mixture was filtered and the filtrate was poured dropwise into 5 N HNO3 aqueous solution to precipitate a fibrous solid. The crude product was washed with 5 N HNO3 aqueous solution and deionized water. The resulting product was dried under vacuum at 80 °C for 15 h to give the perfluorosulfonated polymer (FSPE-1a).
2.6 Membrane preparation
FSPE-1s (0.4 g) in 10 mL of DMAc solution were cast onto a clean flat glass plate (9 cm × 6 cm). Drying the solution at 50 °C under atmospheric pressure for 15 h gave brown and transparent membranes (50 μm thick). The membranes were immersed in 1 M HNO3 for 12 h. The acidification process was repeated three times. The membranes were then washed with deionized water several times and dried under vacuum at 80 °C for 3 h.
3. Results and discussion
3.1 Synthesis and characterization of FSPE-1s
9,9-Bis(4-hydroxy-3-phenylphenyl)fluorene (BHPF) was synthesized via electrophilic substitution reaction of 9-fluorenone with 2-phenylphenol (Scheme 1). BHPF was obtained as a cubic crystal in high yield. The chemical structure and purity of BHPF was confirmed by 1H NMR spectroscopy. Synthesis of the title polymers is illustrated in Scheme 2. Three precursor poly(arylene ether sulfone)s, PE-1a, 1b, and 1c, were synthesized via nucleophilic substitution polycondensation reactions of FPS with BHF, BHPF, and DHPM, respectively. The polycondensation reaction proceeded well in DMAc in the presence of excess potassium carbonate. The progress of the polymerization was confirmed by an increase in the viscosity of the polymerization mixture. The obtained poly(arylene ether sulfone)s were soluble in DMF, DMSO, DMAc, chloroform, and tetrachloroethane. GPC analyses revealed that the polymers were high molecular weight (Mn = 158–270 kDa, Mw = 293–700 kDa) (Table 1). The molecular weight of PE-1b (Mn = 174 kDa, Mw = 293 kDa) was lower than those of PE-1a (Mn = 270 kDa, Mw = 700 kDa) and PE-1c (Mn = 158 kDa, Mw = 496 kDa), probably because of the steric hindrance of the phenyl groups attached ortho to the hydroxyl groups in BHPF. 1H NMR confirmed the chemical structure of the obtained polymers. The 1H NMR spectrum of PE-1b is shown in Fig. 1 (top) as an example, in which all of the aromatic protons were well assigned to the supposed polymer structure. The integral ratios of the peaks were in fair agreement with the expected values.
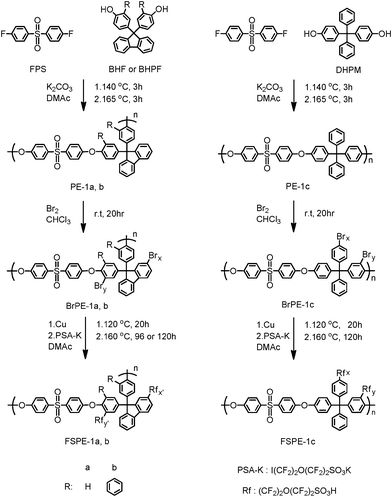 |
| Scheme 2 Synthesis of perfluorosulfonated poly(arylene ether)s (FSPE-1a, 1b, and 1c). | |
Polymer |
Before bromination |
Reaction temperature (°C) |
Reaction time (h) |
After bromination |
Br/unit |
Mn (kDa) |
Mw (kDa) |
Mn (kDa) |
Mw (kDa) |
x
|
y
|
BrPE-1a |
270 |
700 |
r.t. |
20 |
255 |
672 |
1.6 |
1.0 |
BrPE-1a |
270 |
700 |
50 |
20 |
8 |
70 |
2.0 |
1.0 |
BrPE-1b |
174 |
293 |
r.t. |
20 |
179 |
314 |
1.9 |
1.2 |
BrPE-1c |
158 |
496 |
r.t. |
20 |
129 |
418 |
1.0 |
1.0 |
BrPE-1c |
158 |
496 |
50 |
20 |
3 |
7 |
NA |
NA |
Bromination of PE-1a-c was carried out in chloroform with excess Br2. The results are summarized in Table 1. When the reaction mixture was heated at 50 °C, polymers were decomposed as confirmed by GPC analyses in which molecular weights decreased significantly. Therefore, the reaction was carried out under modest conditions at room temperature. The obtained brominated polymers showed similar solubility to the parent polymers. The molecular weight was Mn = 129–255 kDa, Mw = 314–672 kDa, comparable or slightly lower than those of the parent polymers. The 1H NMR spectrum of BrPE-1b is shown in Fig. 1 (middle), which suggests that the bromine groups were substituted at 3 and 7 positions in PE-1b. The assignment has been done by referring our previous bromo-substituted poly(arylene ether sulfone)s prepared from the brominated monomers.24 The degree of bromination was estimated from the integral ratios of the peaks (2/15–17) to be x = 1.9 and y = 1.2. Similarly, the degree of bromination was x = 1.6 and y = 1.0 in BrPE-1a and x = 1.0 and y = 1.0 in BrPE-1c, respectively.
Brominated polymers BrPE-1s were then reacted with PSA-K in DMAc solution in the presence of copper powder via Ullmann coupling reactions to obtain the superacid-modified poly(arylene ether sulfone)s FSPE 1a, 1b, and 1c. The coupling reaction was conducted in two steps (Table 2). In the first step, BrPE-1s were reacted with copper powder at 120 °C for 20 h. In the second step, two times excess of PSA-K to bromine groups was added into the reaction mixture. The reaction was carried out at higher temperature (160 °C) and for longer time (96–120 h) compared to those of the first step. The FSPEs were isolated as brown fibers, which were soluble in polar aprotic organic solvents such as DMAc, DMF and DMSO but insoluble in less polar solvents such as chloroform and tetrachloroethane. Casting from the solution gave pale brown, transparent, and ductile membranes. The ion exchange capacity (IEC) of the FSPE-1 membranes was determined by titration to be 1.09 for FSPE-1a, 1.05 for FSPE-1b, and 0.87 meq g−1 for FSPE-1c, which accounted for the degree of perfluorosulfonation being 42%, 42%, and 37%, respectively. These values were comparable to those (∼37%) of our previously reported FSPEs with lower molecular weight (Mw ∼220 kDa).24
Table 2 Perfluorosulfonation of BrPE-1a, 1b, and 1c
First step |
Second step |
Precursor |
Reaction temp. (°C) |
Reaction time (h) |
Diameter of Cu (nm) |
Reaction temp. (°C) |
Reaction time (h) |
Degree of perfluorosulfonation (%) |
IEC (meq g−1) |
BrPE-1a |
120 |
20 |
50 |
160 |
96 |
42 |
1.09 |
BrPE-1b |
120 |
20 |
100 |
160 |
120 |
42 |
1.05 |
BrPE-1c |
120 |
20 |
100 |
160 |
120 |
37 |
0.87 |
FSPE-1s were characterized by 1H and 19F NMR spectra. The 1H NMR spectrum of FSPE-1b is shown in Fig. 1 (bottom). Due to the higher viscosity of the solution of FSPE-1b, the peaks were broader than those of PE-1b and BrPE-1b. The new peaks (18–22) at lower magnetic field were assigned to the protons of aromatic groups attached with perfluorosulfonic acid groups. Instead, the peaks 15–17 of the brominated aromatic rings were smaller than those of BrPE-1b. The integral ratio of these peaks (15–17/19,22/18,20,21) gave a slightly smaller IEC value (0.88 meq g−1) compared with that (1.05 meq g−1) by titration. Fig. 2 shows the 19F NMR spectrum of FSPE-1b, in which four peaks 1–4 observed between −120 and −80 ppm were well-assigned to the perfluorosulfonic acid groups. The results indicate that the perfluorosulfonic acid groups were successfully introduced onto the polymers.
3.2 Oxidative, hydrolytic, and mechanical stability of FSPE-1 membranes
Taking fuel cell applications into account, oxidative and hydrolytic stability is a critical issue for PEMs. The oxidative and hydrolytic stability of the FSPE-1 membranes was investigated under accelerated testing conditions. The residue in weight, molecular weight (Mw) and IEC after the stability tests is plotted as a function of IEC in Fig. 3 and 4. The FSPE-1a, 1b, and 1c membranes with IEC of 1.09, 1.05 and 0.87 meq g−1, respectively, kept their membrane form with only 10% weight losses after treatment with hot Fenton's reagent. The IEC values decreased by ca. 15–25% and the molecular weight (Mw) decreased more significantly (59% loss for FSPE-1a, 48% loss for FSPE-1b, and 63% loss for FSPE-1c). The results indicate that the major mechanism of the oxidative degradation involves main chain scission. It is reasonable that the electron-withdrawing and hydrophobic perfluorosulfonic acid groups are more oxidatively stable than the polymer main chains with electron-donating ether groups. The oxidative stability of FSPE-1 membranes was comparable with typical sulfonated poly(arylene ether) membranes with no superacid groups.25
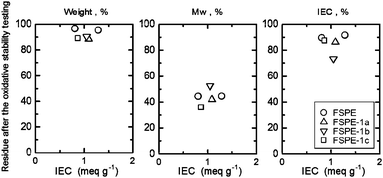 |
| Fig. 3 Oxidative stability of FSPE-1 membranes; losses in weight, Mw and IEC. | |
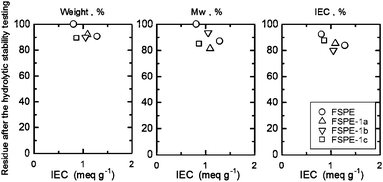 |
| Fig. 4 Hydrolytic stability of FSPE-1 membranes; losses in weight, Mw and IEC. | |
FSPE-1 membranes were more stable to hydrolysis than oxidation and decreases in molecular weight were rather minor (19% loss for FSPE-1a, 7% loss for FSPE-1b, and 14% loss for FSPE-1c). Similar to the oxidative stability, weight and IEC of FSPE-1a-c membranes decreased by ca. 10% and 10–20%, respectively. Since hydrolytic degradation should involve nucleophilic attack by water molecules, arylene ether polymers with high electron density are rather stable to hydrolysis. The oxidative and hydrolytic stability of the newly synthesized FSPE-1 membranes was similar to that of the low molecular weight FSPEs (Fig. 5, IEC = 0.81 and 1.29 meq g−1, Mn = 36 kDa, Mw = 85 kDa as brominated precursor before the perfluorosulfonation), which were our previous version of superacid modified poly(arylene ether)s prepared from the brominated monomer.24
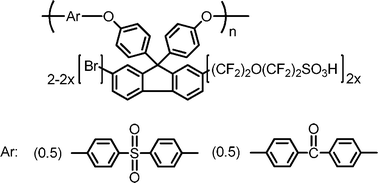 |
| Fig. 5 Chemical structure of FSPEs (IEC = 0.81 and 1.29 meq g−1). | |
Stress–strain curves were measured for FSPE-1a-c membranes at 80 °C and 60% RH and are compared to that of the FSPE membrane in Fig. 6. FSPE-1a-c membranes showed 65 MPa, 38 MPa, and 34 MPa of the maximum stress at break, respectively. These values were significantly higher than that of the FSPE membrane (6 MPa). While FSPE had slightly higher IEC (1.29 meq g−1), it is reasonably considered that the higher molecular weight FSPE-1a-c membranes contributed to much better mechanical stability. It was not possible to obtain high molecular weight FSPE polymers from the brominated monomers since the polymerization conditions need to be mild to avoid unfavorable reactions of bromo groups. The post-bromination method reported herein could give the higher molecular weight FSPE-1a-c with improved mechanical stability.
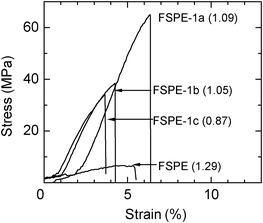 |
| Fig. 6 Stress versus strain curves of FSPE-1a, 1b and 1c membranes at 80 °C and 60% RH with IEC in parenthesis (meq g−1). | |
3.3 STEM images of FSPE-1 membranes
STEM images of lead-ion exchanged FSPE-1a–c membranes are shown in Fig. 7. The images were taken in transmission electron mode, in which the black domains were regarded as the ion-exchanged superacid groups. The FSPE-1a–c membranes showed small uniformly dispersed hydrophilic clusters of ca. 2–3 nm in diameter. The STEM images indicate that the superacid groups aggregate to form ionic clusters and that the main chain structures are less likely to affect the phase separated morphology. The ionic clusters observed in the FSPE-1 membranes were somewhat smaller compared to those of Nafion membrane (ca. 5–6 nm in diameter). The smaller ionic clusters in FSPE-1 membranes presumably reflect the rigid structure of the aromatic main chains, which limit the self-aggregation of the superacid groups in the side chains, resulting in less inter-connected ionic clusters. Similar results were obtained with our previous FSPE membranes.22–24
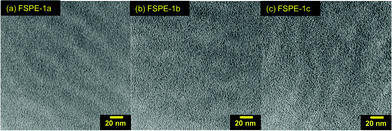 |
| Fig. 7 STEM images of (a) FSPE-1a (IEC = 1.09 meq g−1), (b) FSPE-1b (IEC = 1.05 meq g−1), and (c) FSPE-1c (IEC = 0.87 meq g−1) membranes. | |
3.4 Water uptake and proton conductivity of FSPE membranes
Water uptake and proton conductivity of the FSPE-1 membranes were measured at 80 °C and plotted as a function of relative humidity (RH) in Fig. 8. For comparison, data of Nafion (NRE212) and FSPE are also included. The water uptake of FSPE-1a–c membranes was not in the order of their IEC. Despite its lower IEC, FSPE-1b (IEC = 1.05 meq g−1) membrane showed comparable water uptake to FSPE (IEC = 1.29 meq g−1) membrane. This is possibly because of the sterically bulky structure of the FSPE-1b main chain, which could produce more free volume to contain water molecules. Similarly, FSPE-1c (IEC = 0.87 meq g−1) membrane containing bulky tetraphenylene carbon moieties showed comparable water uptake to FSPE-1a (IEC = 1.09 meq g−1) membrane despite the former's lower IEC.
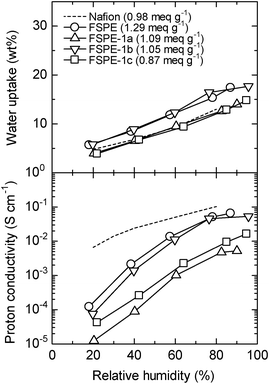 |
| Fig. 8 Humidity dependence of water uptake and proton conductivity of FSPE-1a, 1b, 1c, FSPE, and Nafion NRE 212 membranes at 80 °C. | |
Proton conductivity of the FSPE-1 membranes was approximately in the order of their water uptake. Among the three FSPE-1s, FSPE-1b showed the highest proton conductivity at the humidity range examined. The advantage of FSPE-1b membrane is that its conductivity was comparable to that of FSPE membrane despite its lower IEC. However, the proton conductivity of FSPE-1 membranes was still lower than that of Nafion NRE212 membrane especially at lower humidity, while they share similar IEC values. The results imply that, while introducing superacid groups is effective in improving the proton conductivity, ionic channels and their interconnectivity, which are related to structural factors, such as hydrophobicity and flexibility of the main chains need to be optimized for further improving the properties of aromatic ionomer membranes.
4. Conclusions
A novel versatile synthetic route for superacid-modified poly(arylene ether sulfone)s (FSPE-1s) via post-bromination has been explored. Under mild reaction conditions (at r.t. for 20 h), the post-bromination was controllable and specific positions of the polymers were brominated with minor losses in molecular weights. The degree of bromination was up to 3.1 Br per repeat unit, depending on the polymer structure. The following perfluorosulfonation via Ullmann coupling reaction was not very efficient (conversion was 37–42%). The obtained superacid-modified poly(arylene ether sulfone)s were soluble in polar aprotic organic solvents and provided tough and flexible membranes. The membranes were mechanically more stable (34–65 MPa of the maximum stress at break) than the ionomer membrane (6 MPa) of the similar chemical structure prepared by the pre-bromination method, because of the higher molecular weight of the former (Mn = 129–255 kDa, Mw = 314–672 kDa as brominated precursors). The other properties, such as oxidative and hydrolytic stability, phase-separated morphology, water absorbability, and proton conductivity, were more or less comparable. The main chain structures had some effect on the water uptake and proton conducting behavior. Among the three FSPE-1s investigated, FSPE-1b membrane (IEC = 1.05 meq g−1) showed the highest water uptake and proton conductivity. This is possibly because of the sterically bulky (tetraphenylene carbon) structure of the FSPE-1b main chains, which could produce more free volume to contain more water molecules. The highest proton conductivity was 50 mS cm−1 at 80 °C and 95% RH. However, FSPE-1 membranes performed less well than the Nafion membrane with the comparable IEC value, and need further improvement, especially in terms of the development of ionic channels and their interconnectivity. The post-bromination method reported herein is beneficial for the purpose since it would be applicable to the other aromatic polymers containing electron-donating groups.
Acknowledgements
This work was partly supported by the New Energy and Industrial Technology Development Organization (NEDO) through the HiPer-FC Project, and the Ministry of Education, Culture, Sports, Science and Technology (MEXT) Japan through a Grant-in-Aid for Scientific Research (23350089 and 23656427).
References
-
P. Colomban, Proton Conductors: Solids, Membranes and Gels - Materials and Devices, Cambridge University Press, 1992 Search PubMed.
- K. A. Mauritz and R. B. Moore, Chem. Rev., 2004, 104, 4535–4586 CrossRef CAS.
- M. Rikukawa and K. Sanui, Prog. Polym. Sci., 2000, 25, 1463–1502 CrossRef CAS.
- M. A. Hickner, H. Ghassemi, Y. S. Kim, B. R. Einsla and J. E. McGrath, Chem. Rev., 2004, 104, 4587–4611 CrossRef CAS.
- K. Miyatake and M. Watanabe, Electrochemistry, 2005, 73, 12–19 CAS.
- C. H. Fujimoto, M. A. Hickner, C. J. Cornelius and D. A. Loy, Macromolecules, 2005, 38, 5010–5016 CrossRef CAS.
- K. Goto, I. Rozhanskii, Y. Yamakawa, T. Otsuki and Y. Naito, Polym. J., 2008, 41, 95–104 CrossRef.
- P. Xing, G. P. Robertson, M. D. Guiver, S. D. Mikhailenko and S. Kaliaguine, Macromolecules, 2004, 37, 7960–7967 CrossRef CAS.
- B. Liu, D.-S. Kim, J. Murphy, G. P. Robertson, M. D. Guiver, S. Mikhailenko, S. Kaliaguine, Y.-M. Sun, Y.-L. Liu and J.-Y. Lai, J. Membr. Sci., 2006, 280, 54–64 CrossRef CAS.
- Y. Chikashige, Y. Chikyu, K. Miyatake and M. Watanabe, Macromolecules, 2005, 38, 7121–7126 CrossRef CAS.
- K. Miyatake, Y. Chikashige, E. Higuchi and M. Watanabe, J. Am. Chem. Soc., 2007, 129, 3879–3887 CrossRef CAS.
- K. Miyatake, H. Zhou, H. Uchida and M. Watanabe, Chem. Commun., 2003, 368–369 RSC.
- Y. Yin, J. Fang, T. Watari, K. Tanaka, H. Kita and K.-I. Okamoto, J. Mater. Chem., 2004, 14, 1062–1070 RSC.
- K. Miyatake and M. Watanabe, J. Mater. Chem., 2006, 16, 4465–4467 RSC.
- K. D. Kreuer, J. Membr. Sci., 2001, 185, 29–39 CrossRef CAS.
- T. J. Peckham and S. Holdcroft, Adv. Mater., 2010, 22, 4667–4690 CrossRef CAS.
- Y. A. Elabd and M. A. Hickner, Macromolecules, 2011, 44, 1–11 CrossRef CAS.
- K. Yoshimura and K. Iwasaki, Macromolecules, 2009, 42, 9302–9306 CrossRef CAS.
- H. Li, A. B. Jackson, N. J. Kirk, K. A. Mauritz and R. F. Storey, Macromolecules, 2011, 44, 694–702 CrossRef CAS.
- K. Nakabayashi, T. Higashihara and M. Ueda, Macromolecules, 2011, 44, 1603–1609 CAS.
- K. Xu, H. Oh, M. A. Hickner and Q. Wang, Macromolecules, 2011, 44, 4605–4609 CrossRef CAS.
- K. Miyatake, T. Shimura, T. Mikami and M. Watanabe, Chem. Commun., 2009, 6403–6405 RSC.
- T. Mikami, K. Miyatake and M. Watanabe, ACS Appl. Mater. Interfaces, 2010, 2, 1714–1721 CAS.
- T. Shimura, K. Miyatake and M. Watanabe, Bull. Chem. Soc. Jpn., 2010, 83, 960–968 CrossRef CAS.
- T. Shimura, K. Miyatake and M. Watanabe, Eur. Polym. J., 2008, 44, 4054–4062 CrossRef CAS.
Footnote |
† Electronic Supplementary Information (ESI) available: Experimental details include measurements and characterization procedures of the membranes. See DOI: 10.1039/c2ra20444d |
|
This journal is © The Royal Society of Chemistry 2012 |