DOI:
10.1039/C2RA20243C
(Paper)
RSC Adv., 2012,
2, 3678-3683
Sol–gel prepared bismuth titanate for high temperature ultrasound transducers
Received
10th February 2012
, Accepted 13th February 2012
First published on 14th February 2012
Abstract
Bismuth titanate (BIT) ceramic was prepared via a sol–gel technique and characterised for use as a high temperature ultrasound sensor. Transducers were prepared as both a pressed ceramic disc and a thick film screen printed on a stainless steel shim. Platinum paste was used as an electrode, and oil bath poling at 120 kV cm−1 and 140 °C was done to align dipoles within the samples. The thermal stability of the BIT with regards to the piezoelectric coefficient was examined. Through-thickness ultrasound measurements were made on aluminium and mild steel blocks, and also on a stainless steel pipe at temperatures of up to 230 °C.
Introduction
The use of ultrasound for non-destructive testing (NDT) is well established but the current lack of a system which will operate at raised temperatures limits the use for industries, such as power generation, steel, and petrochemical, which have a need for inspection of the plant components. Two possible methods which have been reported include high temperature electromagnetic acoustic transducers (EMATs), which have been shown to work up to 250 °C,1 and integrated piezoelectric transducers prepared through a sol–gel spraying route, which have shown good properties up to 600 °C for bismuth titanate2 and 800 °C for LiNbO3/PZT composites.3 Bismuth titanate ceramics are favoured as a possible high temperature ultrasound transducer due to the high Curie temperature of 675 °C.
Bismuth titanate Bi4Ti3O12 is a ferroelectric with an Aurivillius structure,4,5 where a perovskite-like (Bi2Ti3O10)2− layer is sandwiched between (Bi2O2)2+ layers. The piezoelectric properties arise below the Curie temperature where there is displacement of the titanium ion in the perovskite layer; this asymmetry generates a dipole within the crystal. For a ceramic, rather than a single crystal, this displacement can be aligned by poling the sample, where a voltage is applied across a crystalline sample, often at a raised temperature to reduce the coercive field. Bismuth titanate can be difficult to pole due to its high electrical conductivity, although this can be reduced by doping. The conductivity is also related to the preparation route; it was found that for the W6+ doped BIT ceramic, a chemical preparation route showed lower conductivity than a conventional mixed oxide route. This was ascribed to the platelet growth with the aspect ratio of the platelets determining levels of conductivity.6 Some piezoelectric ceramics can suffer from thermal depoling, for example bismuth sodium titanates, where the dielectric properties and Curie temperature appear suitable for a high temperature ultrasound sensor but will depole as the temperature is raised above 187 °C, leading to reduced d33 values.7
The sol–gel route is an established technique for the preparation of ceramic powders. Because mixing occurs on a molecular level, very fine homogeneous powders can be prepared, leading to lower processing temperatures and the possibility of preparing compositions which are not viable through traditional mixed oxide processing. The sol–gel preparation of bismuth titanate has been reported in the literature;8,9,10 it is a non-trivial process due to the highly reactive nature of the titanium alkoxide precursor which needs to be handled in a dry atmosphere. Co-precipitation and citrate gel preparation routes of bismuth titanate have also been shown to produce fine homogeneous powders leading to ceramics with better microstructures than conventionally prepared BIT.11,12 In this paper a sol–gel route has been developed which enabled bismuth titanate to be prepared, characterised and subsequently formed as an ultrasound transducer capable of operating at high temperatures.
Experimental
Bismuth titanate was formed through a sol–gel process using stoichiometric quantities of bismuth nitrate pentahydrate BiNO3·5H2O and titanium propoxide Ti(OC3H7)4 as precursors. Titanium alkoxides are extremely sensitive to moisture due to the electronegativity of the titanium atom. To reduce the reactivity of the titanium propoxide, the co-ordination number of the titanium ion can be increased from 4 to 6 by forming a complex with nucleophilic acetate ions.13 This was done by the addition of acetic acid to the titanium propoxide in a stoichiometric ratio of 4
:
1. This stabilised the compound sufficiently for it to be exposed to air without immediate hydrolysis and condensation. The bismuth nitrate crystals were then dissolved in acetylacetonate to form another stable complex. The two solutions were mixed and exposed to the air to allow gelling to take place. Although there was an increase in viscosity, gelling did not complete, possibly due to incomplete hydrolysis, or the complexes formed which reduce the possibility of crosslinking. The sample was dried, vibromilled in methanol, dried and sieved to give a powder with a particle size <38 μm suitable for screen printing. Powders were calcined at 400 °C to remove any remaining organic species.
Sample discs were prepared for characterisation by uniaxial pressing in a 13 mm die and firing in a belt furnace. The firing zones were set with a top temperature of either 750 °C, 800 °C, 850 °C, 900 °C, 950 °C or 1000 °C. Thick film samples were also prepared by mixing calcined powder with a binder and diluent (Gwent Electronic Materials) in a triple roll mill, to break up any aggregates and produce a pseudoplastic paste of a suitable viscosity for screen printing. Screen printing was done using a DEK 240 to print 8 mm circles onto 100 μm stainless steel shim substrate. To give a good ceramic-to-metal seal, an oxide layer needs to develop on the surface of the metal so that a chemical bond can form across the interfacial layer.14 The stainless steel was pre-oxidised by cleaning with acetone in an ultrasonic bath followed by firing at 650 °C. After printing the substrate was dried at 150 °C for 15 min before firing in the belt furnace, with a burnout zone to remove any binder.
Platinum paste was chosen to deposit electrodes on the faces of the discs since it suffers from fewer migratory problems at elevated temperatures than the more commonly used silver dag. After platinum electrodes were deposited on each face, oil bath poling was carried out on the BIT discs to align the dipoles within the structure. A voltage of 8 kV was applied across the discs at a temperature of 140 °C in a silicone oil bath for 20 min; the temperature was allowed to fall to room temperature while the voltage was still applied, leading to a field across the sample of 80 kV cm−1 for a 1 mm thick disc. A second poling at 12 kV (120 kV cm−1) increased the d33 piezoelectric coefficient. Subsequent heating of the sample to 500 °C showed no change in d33 value, although heating to 600 °C for one hour showed a slight decrease. This allowed platinum wires to be attached to the transducer with a high temperature paste fired at 600 °C; the thermal build of a sensor is important so that subsequent heat processes have no effect on previous steps. A platinum paste contact on the side of the sample combined with use of a laser micromachining system to scribe an arc on the top and provide electrical separation allowed both platinum wire contacts to be on the top face of the transducer, as shown in Fig. 1.
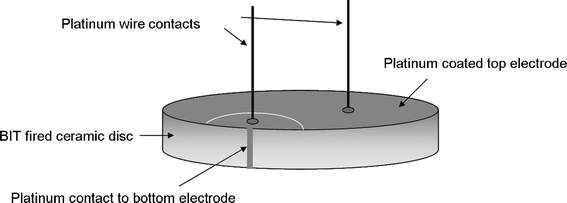 |
| Fig. 1 Sensor build for high temperature BIT transducer. | |
Oil bath poling was also carried out on the screen printed samples, applying a voltage of 2 kV across a film thickness of 40 μm for 15 min at 140 °C. Here the stainless steel shim acted as the bottom electrode, while platinum paste was fired on the surface to provide a top electrode.
The effect of ageing on the samples was examined, along with effects due to thermal depoling. Two discs of sol–gel based BIT were prepared and poled. The piezoelectric coefficient was measured for each over a period of a few days, then on subsequent days one disc (BIT A) was heated for two hours before the piezoelectric coefficient was measured again and compared with the unheated disc (BIT B). By increasing the set temperature each day until the Curie temperature was reached, the effects of thermal depoling could be ascertained. Thermal cycling of a third disc to 600 °C simulated the thermal effects on a transducer in a working environment, with ten cycles being employed and a hold time at temperature of ten minutes.
Characterisation
The crystalline development of BIT with temperature was followed by X-ray diffraction (XRD) using a Bruker with a Cu-Kα source and an Anton Parr furnace for high temperature, while thermal characterisation was carried out using differential thermal analysis (DSC/DTA). d33 measurements were made with a YE2730A meter, and density measurements were made on fired pellets using the Archimedes method. The value of the piezoelectric coefficient lies in a range similar to undoped BIT15 with a d33 value of 17 pC N−1 being typical. For the screen printed samples on shim, d33 values of 8 pC N−1 were produced. Depoling is thought to arise from point defects which allow rearrangement of domain boundaries to give a lower net polarisation when the temperature is raised.16 A low defect concentration should protect against this, and so a sol–gel preparation technique which requires a reduced firing temperature compared with a conventional mixed oxide technique should be more resistant to depoling. To look at the effect of temperature on depoling, a sensor was heated for two hours at 100° intervals, with a final two heat treatments being at 650 °C and 700 °C. The sample was allowed to cool between each heat treatment and the d33 piezoelectric coefficient measured. The results in Fig. 2 show that the piezoelectric coefficient remains stable to about 500 °C, decreasing sharply above 600 °C and disappearing above the Curie temperature. Thermal cycling showed a small effect on the piezoelectric coefficient, with a d33 value of 11 pC N−1 after ten cycles to 600 °C.
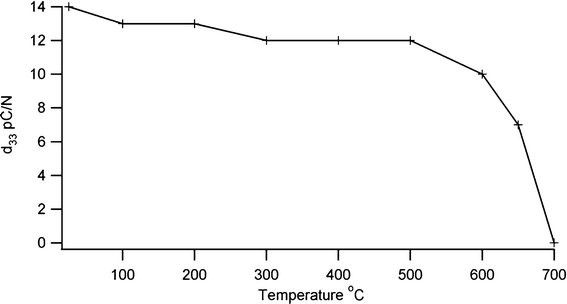 |
| Fig. 2 Effect of thermal depoling on piezoelectric coefficient of bismuth titanate. | |
Differential thermal analysis (DTA) of the sol–gel prepared powder was done using a Stanton Redcroft DTA673-4, against a quartz reference and with a heating rate of 10° min−1. Results are shown in Fig. 3. On heating there is an exotherm present at 573 °C due to the α-β quartz transition. A second peak, endothermic, at 737 °C is due to the α-δ phase transition of unreacted Bi2O3, and a third at 872 °C arises from a further phase transition of Bi4Ti3O12, currently under investigation. The melting point of Bi12TiO20 is reported as 873 °C,17 but the presence of this phase is not indicated by X-ray diffraction patterns. On cooling Bi4Ti3O12 undergoes two phase transitions at 861 °C and 672 °C, with the Curie temperature being identified at 672 °C, where Bi4Ti3O12 changes from tetragonal to orthorhombic crystal structure. The thermal coefficient of expansion (TCE) of the bismuth titanate was measured using a Netzsch dilatometer, the sample being prepared as an isostatically pressed rod, sintered at 900 °C. The TCE value was found to be 7.9 MK−1 (200–600 °C).
High temperature XRD showed the presence of Bi4Ti3O12, Bi2Ti2O7 and Bi2O3 at 650 °C, the pattern being shown in Fig. 4. Although Bi4Ti3O12 is clearly indicated at this temperature, the transition to tetragonal phase is not present in the heating trace for the DTA, possibly due to a lack of order or symmetry within the structure prior to sintering. It is possible that the peak at 737 °C is due to this displaced transition rather than to B2O3. Further high temperature diffraction patterns shown in Fig. 5 indicate that peaks due to various phases of titanium oxide are present at 600 °C; Bi2Ti2O7 is no longer present at 700 °C and Bi2O3 is no longer present at 900 °C. The loss in low angle signal intensity at 850 °C and 900 °C is due to shrinkage of the sample in the holder through sintering and this is borne out by density measurements in Table 1. Table 1 also indicates the effect of increasing firing temperature with decrease in d33 values; this is thought to arise from platelet growth, with a corresponding increase in conductivity.6 A room temperature XRD pattern of the powdered material after sintering shows the presence of both monoclinic and orthorhombic forms of Bi4Ti3O12.
Table 1 The effect of firing temperature on density and d33 values, showing that density increases with firing temperature while d33 values decrease
Firing temperature |
Density g cm−3 |
d
33 pC N−1 ±2 |
750 °C |
5.9 |
17 |
800 °C |
5.7 |
17 |
850 °C |
7.2 |
16 |
900 °C |
7.5 |
12 |
1000 °C |
7.7 |
6 |
Ultrasonic response
Thickness measurements were made using a Sonemat PR100 pulser-receiver unit with high gain as a signal generator. A schematic of the set-up is shown in Fig. 6. Initial measurements were done at room temperature on a 25.6 mm thick aluminium block with a standard ultrasound gel couplant. Back wall reflections can clearly be seen in Fig. 7, with the first reflection arriving at 16 μs and subsequent reflections arriving at 8.02 μs intervals corresponding to the longitudinal wave velocity in aluminium of 6380 ms−1. The ringing observed in the response is due to the undamped nature of the transducer. Pyrogel 100, capable of operating up to 300 °C, was used as a high temperature couplant and the sample heated on a hotplate, with the surface temperature of the sample recorded as 230 °C using a K-type thermocouple. The response, which has a reduced signal amplitude, clearly indicates the back wall reflections, as shown in Fig. 8. The time interval between reflections is 8.33 μs, indicating a slower longitudinal wave velocity of 6140 ms−1. Measurements taken using a thick film bismuth titanate transducer on a 9 mm thick 316 stainless steel pipe are shown in Fig. 9, at room temperature and in Fig. 10, at 225 °C on a mild steel plate. Again there is reduced signal amplitude at higher temperatures but the reflections can be clearly seen. The pressed disc transducers were operating at a typical frequency of 5 MHz, while the thick film transducers were operating with frequencies between 11 and 12 MHz.
 |
| Fig. 6 Schematic of set-up for through thickness measurements. | |
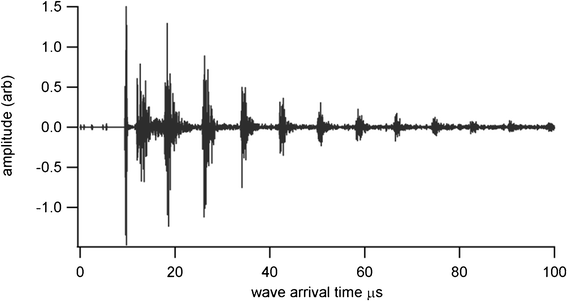 |
| Fig. 7 Undamped room temperature ultrasound response for BIT sensor with standard gel couplant, for thickness measurement of 25.6 mm aluminium plate. | |
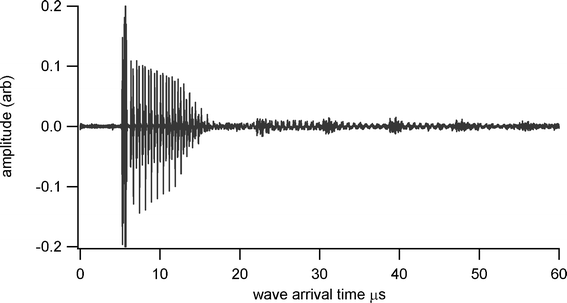 |
| Fig. 8 Undamped ultrasound response at 230 °C for BIT sensor with Pyrogel couplant, for thickness measurement of 25.6 mm aluminium plate. | |
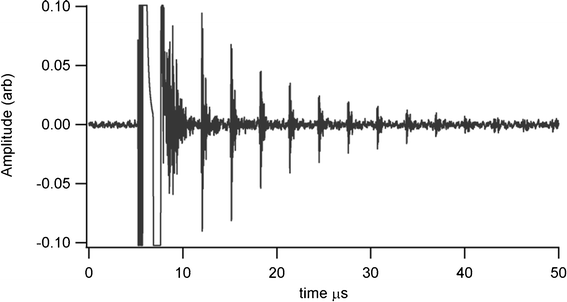 |
| Fig. 9 Undamped room temperature ultrasound response for screen printed BIT transducer on stainless steel shim, for thickness measurement of 9 mm thick stainless steel pipe. | |
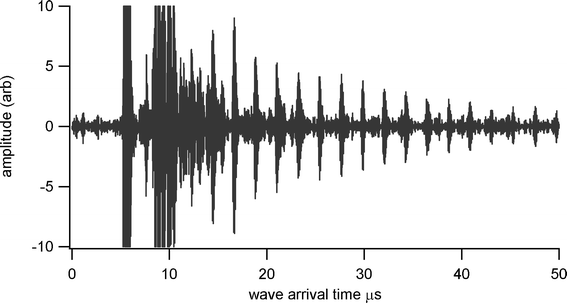 |
| Fig. 10 Undamped ultrasound response at 225 °C for screen printed BIT transducer on stainless steel shim, for thickness measurement of 6 mm thick steel plate. | |
Conclusion and discussion
Bismuth titanate Bi4Ti3O12 was prepared by a sol–gel method using titanium propoxide and bismuth nitrate pentahydrate as starting materials. High temperature XRD showed that Bi4Ti3O12 starts to develop by 600 °C, with 100% phase formation by 850 °C; this is a lower temperature than conventional preparation routes. Sintering begins at about 850 °C with an increase in density to 7.2 g cm−3. Increasing the firing temperature leads to an increase in density but is also accompanied by a reduction in d33 values. Oil bath poling of a pressed sample enabled a piezoelectric coefficient of 17 pC N−1 to be achieved, and thermal cycling to 600 °C showed only a small reduction, indicating that a sensor will remain piezoelectrically active after use at high temperature. Thick film samples typically showed a piezoelectric coefficient of 8 pC N−1. Successful through thickness measurements on both mild and stainless steel samples were undertaken using transducers prepared as a disc and also as a thick film (screen printed bismuth titanate on stainless steel shim) using platinum as a high temperature electrode. These measurements were made at both room temperature and at up to 225 °C using high temperature gel couplants. For permanent bonding, couplants capable of working at higher temperature will need to have a thermal coefficient of expansion compatible with both the bismuth titanate transducer measured as 7.9 MK−1, and the sample to be measured.
Acknowledgements
This work was supported by BP, RWE npower, Shell and Tenaris through the Research Centre for Non-Destructive Evaluation (RCNDE) and EPSRC grant EP/G042284/1.
References
- F. Hernandez-Valle and S. Dixon, NDT&E Int., 2010, 43, 171–175 CrossRef CAS.
- C. T. Searfass, B. R. Tittmann and D. K. Agrawal, Rev. Prog. Quant. Nondestr. Eval., 2009, 28, 1751–8 Search PubMed.
- M. Kobayashi, C.-K. Jen, J. F. Bussiere and K.-T. Wu, NDT&E Int., 2009, 42, 157–161 CrossRef CAS.
- B. Aurivillius, Ark. Kemi, 1949, 1(1), 499–512 CAS.
- T. Jardiel, A. C. Caballero and M. Villegas, J. Ceram. Soc. Jpn., 2008, 116(1352), 511–518 CrossRef CAS.
- M. Villegas, A. C. Caballero, C. Moure, P. Durán and J. F. Fernández, J. Am. Ceram. Soc., 1999, 82(9), 2411–16 CrossRef CAS.
- Y. Hiruma, H. Nagata and T. Takenaka, J. Appl. Phys., 2009, 105, 084112 CrossRef.
- M. Sedlar and M. Sayer, Ceram. Int., 1996, 22, 241–247 CrossRef CAS.
- A. V. Prasada Rao, A. I. Robin and S. Komarneni, Mater. Lett., 1996, 28, 469–473 CrossRef.
- H. Gu, P. Chen, Y. Zhou, M. Zhou, A. Kuang and X. Li, Ferroelectrics, 1998, 211, 271–280 CrossRef CAS.
- S. R. Dhage, Y. B. Khollam, S. B. Dhespande, H. S. Potdar and V. Ravi, Mater. Res. Bull., 2004, 39, 1993–8 CrossRef CAS.
- M. Villegas, C. Moure, J. F. Fernandez and P. Duran, J. Mater. Sci., 1996, 31, 949–955 CrossRef CAS.
- J. Livage and C. Sanchez, J. Non-Cryst. Solids, 1992, 145, 11–19 CrossRef CAS.
-
A. J. Sturgeon, PhD thesis, Warwick University, 1987 Search PubMed.
- M. Villegas, T. Jardiel and G. Farías, J. Eur. Ceram. Soc., 2004, 24, 1025–9 CrossRef CAS.
- T. Zeng, H. Yan and M. J. Reece, J. Appl. Phys., 2010, 108, 096101 CrossRef.
- T. M. Bruton, J. Solid State Chem., 1974, 9, 173–5 CrossRef.
|
This journal is © The Royal Society of Chemistry 2012 |