DOI:
10.1039/C2RA20136D
(Paper)
RSC Adv., 2012,
2, 3396-3402
“Green” anionic wormlike micelles induced by choline†
Received
23rd January 2012
, Accepted 25th January 2012
First published on 28th February 2012
Abstract
In such application fields as oilwell stimulation, tertiary oil recovery and drug release, environmental-friendly wormlike micelles are largely preferred. The present work reports a “green” wormlike micellar system formed by a bio-based anionic surfactant, sodium erucate (NaOEr), and a biodegradable hydrotrope, choline. The micellar solutions were examined by means of rheology and cryo-TEM observation. Besides possessing lower toxicity, choline exhibits a stronger ability to induce micelle growth than tetramethylammonium, which is commonly used to promote the formation of anionic worms, and the choline–NaOEr system is more thermosensitive with increasing counterion concentration. These notable advantages enable the choline–NaOEr wormlike micellar system to find potential applications in biomedical areas and clean fracturing fluids.
Introduction
Some surfactants can self-assemble into long, flexible wormlike micelles under certain conditions, for example, by increasing its concentration or adding a hydrotrope. Above a threshold concentration, these wormlike micelles will entangle into a transient network, showing viscoelastic behavior analogous to polymer solutions.1 However, unlike polymers that are readily degraded under shear, wormlike micelles can reversibly break and recombine. Such unique rheological properties furnish them with the potential to be applied in fracturing stimulation of oilwells,2 tertiary oil recovery,3,4 and drug delivery.5 In recent years, with increasing appeals for environmental protection, more environmentally-benign chemicals in crude oil production6 and less toxic carrier compounds in biomedicines7 are demanded. Thus, it is no doubt that “green” wormlike micelles are desirable when used in these industries.
It is well recognized that anionic surfactants demonstrate more biodegradable and less toxic bahaviour than cationic ones.8,9 In addition, when used in oil production, anionic surfactants are always preferred as they are less adsorbed on negatively-charged rock surfaces than their cationic and zwitterionic counterparts.10,11 Nevertheless, the overwhelming majority of research to date has centered around wormlike micelles constituted by C16-tailed cationic surfactants,12–14 and less attention has been paid on the anionic systems. The anionic worms reported so far are mainly formed by oleate salts or alkyl sulfates with C18 chains and below, for example, sodium dodecyl sufate15,16 and sodium oleate.9,17 Comparatively, these micellar systems exhibit either weak viscoelasticity even at very high surfactant and salt concentrations,9,15,18 or poor stability at high temperatures.17,19 According to the reports on cationic and zwitterionic wormlike micelles,20–26 one can find that the longer the hydrophobic tail length, the stronger the viscoelasticity and the better the thermostability. Given the enhanced viscoelasticity of C22-tailed cationic systems,21,22 an attempt has been made in our laboratory to investigate C22-tailed anionic systems, and we found that the C22-tailed anionic surfactant sodium erucate (NaOEr), can form very strong viscoelastic wormlike micelles even at very low concentrations in the presence of a tetraalkylammonium salt.27 NaOEr is simply prepared by neutralizing erucic acid which is a low-toxic renewable resource from rape and mustard seeds.28 Moreover, the fatty carboxylates can be degraded by β-oxidation and completely mineralised or incorporated into biomass.29 Our latest study25 also showed that erucate-based surfactants can be decomposed at the cis double bond of C-13 in the hydrophobic tail when aging at high temperature. According to these two characteristics, i.e., low toxicity and biodegradability, NaOEr qualifies as a green surfactant,30 and probably this is why its short-chain fatty carboxylates counterparts are widely used in household and cosmetics formulations.31,32
Hydrotropes or salts are often required for ionic surfactants to form wormlike micelles. Tetraalkylammonium ions (TAA+) are common hydrotropes for anionic surfactants.9,27 Nevertheless, the introduction of TAA+ may simultaneously render the solution potentially harmful, because TAA+ usually acts as a phase-transfer catalyst and can transport ions across biological membranes,33 and can block cellular ion channels even at very low concentrations.34 An alternative way to avoid the underlying harm but retain the positive functions of TAA+ is to use bio-origin quaternary ammonium ions such as choline. Choline chloride is the least toxic amine, 5.5 times less toxic than TMA+ chloride and over 500 times less toxic than tetrabutylammonium chloride for rat glioma cells;35 moreover, it can be esterified to acetylcholine and phospatidylcholine and decomposed in the environment or the human body.35 On the other hand, choline is a dietary component essential for normal function of all cells and plays a key role in early brain development,36 and therefore it was identified as a necessary nutrient for humans and a daily intake was recommended by the National Academy of Sciences, USA, in 1998.37
Despite the biocompatibility and predominant use of choline in nutrition, to the best of knowledge, no reports to date concern its use in formulating wormlike micelles. In the present study, this environmentally-friendly hydrotrope was employed to induce the growth of NaOEr-based wormlike micelles, and the effects of both salt concentration and temperature were examined by rheology measurement and cryogenic transmission electron microscopic (cryo-TEM) observation. For comparison, the addition of TMA+ into NaOEr solution was also studied under the same conditions.
Experimental section
Materials
Tetramethylammonium bromide and choline chloride (Sinopharm Chemical Reagent Co.), both of analytical grade, were used as received. NaOEr was prepared via saponification of erucic acid according to our previously reported procedure.27 The molecular structures of these compounds are shown in Scheme 1.
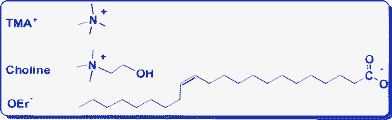 |
| Scheme 1 Molecular structures of choline, TMA+ and OEr− used in this work. | |
The surfactant solutions were prepared by dissolving set amounts of NaOEr and the organic salt in triply-distilled water with gentle agitation. The solution mixture was then homogenized and kept in a water bath at 50 °C for at least 24 h to ensure equilibration. The pH of the solution was set at ∼10 to prevent soap hydrolysis.38
Rheology
The rheological measurements of the sample solutions were performed on a Physica MCR301 rotational rheometer (Anton Paar, Austria), using a concentric cylinder geometry with a measuring bob radius of 13.33 mm and a measuring cup radius of 14.46 mm. Frequency sweep measurements were performed in the linear viscoelastic regime of the samples and a solvent trap was used to minimize water evaporation.
Cryo-TEM observation
The specimens were pre-heated at 50 °C, and then 5 μL of the micellar solution was taken to spread on a holey carbon film supported by a copper grid. The thin layer of the micellar solution on the grid was immediately vitrified by rapid immersion into liquid ethane cooled at ca. −175 °C by liquid nitrogen in a temperature-controlled freezing unit. Then the vitrified specimen stored in liquid nitrogen was transferred into a JEM2010 cryo-microscope using a Gatan 626 cryo-holder and its workstation. The temperature was monitored and kept constant in the chamber during all the sample preparation steps. Images were obtained at a temperature of approximately −170 °C and with a 200 kV acceleration voltage, using a Gatan 832 camera.
Results
Effect of hydrotrope concentration on rheological behaviors
The shape and size of the micelles depend not only on the geometry of the surfactant, but also on the nature of hydrotrope added.39 The influence of the structural difference between choline and TMA+ on NaOEr micellar solution is compared by rheological behaviours. The concentration of NaOEr, CD, was fixed at 100 mM, while the concentration of the hydrotropes, CS, was varied to induce the micellar morphology transition.
Steady rheology.
Fig. 1A shows the steady rheological results of the NaOEr–choline system at 50 °C while analogous data for the NaOEr–TMA+ system are given in Fig. S3A (ESI†). It is seen that all the sample solutions show similar behaviours irrespective of hydrotrope concentration. At low shear rate, the shear viscosity is independent of shear rate and a viscosity plateau is observed, showing a typical Newtonian fluid behaviour. Upon extrapolating the plateau values to zero shear rate, the zero-shear viscosity (η0) can be obtained. The high viscosity observed in the plateau can be interpreted as the result of the presence of an entangled network of wormlike micelles,40 which is also verified by cryo-TEM observation for the representative sample of 100 mM NaOEr + 1 M choline (Fig. 2). When exceeding critical shear rates, shear-thinning behaviour occurs, indicative of alignment of the entangled network of wormlike micelles with increasing shear rate.20
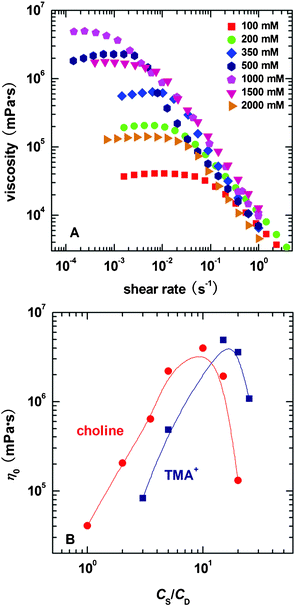 |
| Fig. 1 (A) typical steady rheological results for the NaOEr–choline system with varied choline concentrations, and (B) comparison of the concentration effect for choline and TMA+ on η0. NaOEr concentration is held constant at 100 mM. All the measurements are carried out at 50 °C. | |
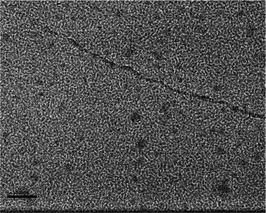 |
| Fig. 2 Cryo-TEM image for the specimen from 100 mM NaOEr + 1 M choline. The scale bar is 100 nm. | |
The effect of CS on η0 is shown in Fig. 1B. The sample solution becomes visibly viscous at 100 mM choline, indicating the formation of long threadlike micelles. Such a hydrotrope concentration is denoted the threshold concentration (CS*) elsewhere.41 Above CS*, η0 of the two systems both show maximum values as a function of the ratio of salt concentration to surfactant concentration (CS/CD). The initial increase of η0 with salt concentration should be ascribed to micellar growth, while the decrease at high salt concentrations is universally explained as due to the formation of branched micelles.13,42 The increase of salt concentration weakens the repulsion between headgroups, and compared to that of the molecules in the cylindrical body of the micelles, the curvature energy of the surfactant molecules in the end caps will increase.40 However, when the free energy cost associated with the cross-link formation becomes comparable to that needed for the formation of end caps, branching is expected to occur.40
Dynamic rheology.
Representative plots of elastic modulus (G′) and loss modulus (G′′) as a function of oscillatory shear frequency (ω) are shown in Fig. 3A. The corresponding experimental results for the NaOEr–TMA+system are given in Fig. S3B (ESI†). These curves are for two samples at different choline concentrations, 1.5 and 2 M. They both exhibit viscoelastic responses, and at low frequencies agree well with the Maxwell model (eqn (1)):14 |  | (1) |
where G0 is the plateau modulus, i.e., the value of G′ at high frequencies, and τR refers to relaxation time. The solid lines in Fig. 3A represent the fits of experimental G′ and G′′ which follow the power-law model at low frequencies with exponents of 2 and 1, respectively. At intermediate frequencies, the dynamic moduli G′ and G′′ show crossover at a characteristic frequency (ωC), the inverse of which represents τR. Thereafter a deviation from Maxwell model occurs and the system shows a predominantly elastic response, i.e., G′ > G′′. The storage modulus, G′, tends asymptotically to G0, and G′′ shows an upturn instead of a monotonous decline with increasing frequency, which is generally considered as a Rouse mode due to the faster relaxation processes.43 The remarkable difference between the two spectra is that the frequency range shifts towards higher frequencies as the choline concentration increases.
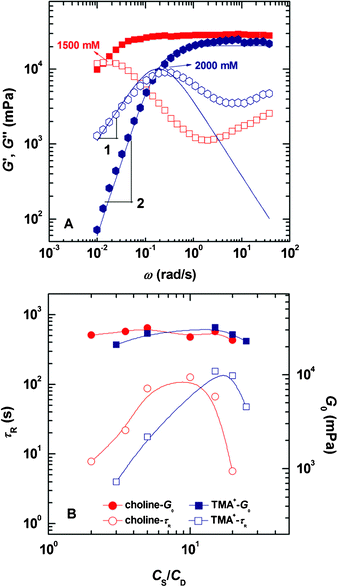 |
| Fig. 3 Representative dynamic rheological results (A) for the NaOEr–choline system and variation of the rheological parameters, τR and G0 (B) as a function of CS for the two systems; NaOEr concentration is fixed at 100 mM. | |
The variations of G0 and τR as a function of CS/CD are presented in Fig. 3B; τR of the two systems both show peaks, but G0 demonstrates little dependence on salt concentration. According to Cates et al.,14,43 reptation or diffusion of wormlike micelles along their own contour network conforms to the stress relaxation mechanism. Thus the magnitude of τR is concerned with the average length of the wormlike micelles and is relevant to both surfactant and hydrotrope concentrations. G0 is related to the density of entanglement in the network,44 and only depends on the surfactant concentration.
It is apparent that CS* and the salt concentration where the maximum η0 and τR lie for choline system are both lower than those of the TMA+ system. The discrepancy should be assigned to their structural diversity. According to Klein et al.,45 TMA+ and choline exert similar steric hindrance influences on the micellization process of spherical micelles formed by aliphatic carboxylates, and therefore the alcohol group makes little difference to the size of choline. In other words, the screening effect of the two counterions to the headgroups is comparable to each other. This reflects that more than one factor influences the micelle growth.
Effect of temperature on rheological behavior
Temperature is one of the most important factors determining solution properties; moreover, the practical applications of micellar solutions are usually confined at given temperatures. Therefore it is necessary to investigate the influence of temperature on the micellar system formed in this work. The effect of temperature on phase behaviors of NaOEr solutions is shown in Fig. S1 (ESI†). Fig. 4 shows the steady and dynamic rheological responses for the sample 100 mM NaOEr + 1 M choline (corresponding to the solution at the peak of the η0–CS/CD plot in Fig. 3A) in the temperature range of 50–90 °C. With increasing temperature, the entire dynamic frequency spectra shift to higher frequencies or shorter timescales (Fig. 4B), while η0 decreases monotonously (Fig. 4A). The influence of temperature on viscosity is due to the decreased average micelle length upon increasing temperature.13 It is worth noting that η0 is still >103 mPa s at 90 °C, which suggests the good stability of the entangled micelles at high temperature. This can be attributed to the long breaking time of the micelles because of the long hydrophobic tail attached on the surfactants.22 It is known14 that the micellar contour length decreases with increasing temperature according to the Arrhenius equations: | 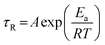 | (2) |
| 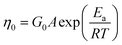 | (3) |
where Ea is the activation energy, R is the gas constant, T is the absolute temperature, and A is the pre-exponential factor. According to eqn (3), G0 is independent of temperature, which is also confirmed by the experimental results (Fig. 4). As shown in the inset of Fig. 4A, semilog plots of η0 and τRvs. 1/T fall on straight lines, indicative of Arrhenius behaviors. The activation energy estimated from the slope of both two plots is ca. 166.3 kJ mol−1, close to those found in other wormlike micelles.20,40
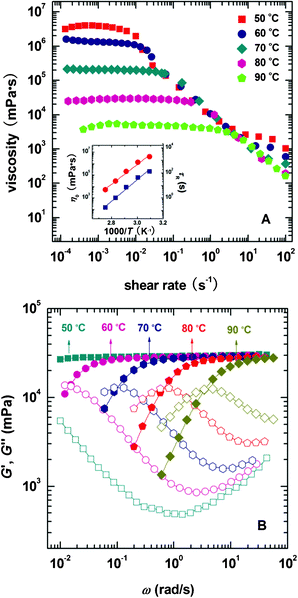 |
| Fig. 4 Effect of the temperature on the rheology of the 100 mM NaOEr + 1 M choline system: (A) steady-shear viscosity plots, the inset shows Arrhenius plots of η0 and τRvs. 1/T. The slopes of the straight lines yield the activation energy Ea. (B) dynamic frequency spectra, the lines are a guide for the eye. | |
In Fig. 5, η0 is plotted as a function of choline content at different temperatures. Within the investigated temperature range, η0 all show maxima with increasing salt concentration, and the peak values decrease monotonously with increasing temperature. However, the location of maxima shifts to lower salt concentration with increasing temperature. This implies that the micellar morphology variation with salt concentration is similar at different temperatures, but at high temperature a lower amount of choline is needed to obtain the maximum viscosity. Such a finding is consistent with other wormlike micelle systems, which results from the increase of free energy of hemispherical end-caps when increasing temperature.46 Alternatively, the rapid motion of counter-ions at high temperature results in more frequent contact with surfactant molecules, thus enhancing the screening effect. Therefore less salt is required at high temperature to reach the viscosity peak maximum.
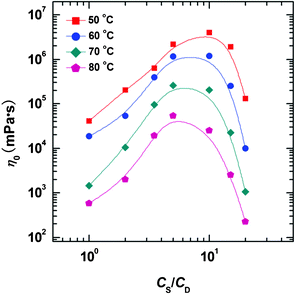 |
| Fig. 5
η
0 plotted as a function of CS/CD at different temperatures for the NaOEr–choline system at fixed NaOEr concentration of 100 mM. | |
The plots of activation energy as a function of salt content are presented in Fig. 6. An interesting observation is that the fluctuations of Eavs. CS are obviously opposite for these two systems. At low CS, Ea of TMAB system is close to 180 kJ mol−1. With increasing TMAB concentration, Ea decreases successively down to around 120 kJ mol−1; while for the choline system, Ea continuously increases from 145 to 203 kJ mol−1 with increasing choline concentration. This suggests that the mechanism of the Ea evolution with CS is different for these two systems.
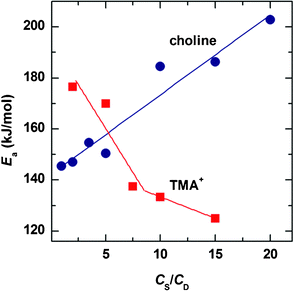 |
| Fig. 6 Comparison of the effect of hydrotrope concentration on activation energy variation. | |
Discussion
Enhanced contribution of choline to micelle growth
Subtle changes of the counter ion may lead to a marked effect on the macroscopic rheological behavior of micellar systems. The smaller CS* for the choline system relative to TMA+ indicates that choline possesses a stronger ability to induce the micelle growth due to the replacement of –CH3 on TMA+ by –CH2CH2OH. The presence of –OH in the choline molecule enhances its hydrophilicity compared with TMA+,47 but it is unfavorable for choline to penetrate the micelle because of the repulsive interaction between the hydrophilic –OH and the hydrophobic hydrocarbon tail of the surfactant.48 Therefore choline ions are supposed to be exclusively oriented with the –OH moiety projecting outside and the positively charged end approaching the hydrophilic headgroup of the surfactant by electrostatic interactions. The affinity to water of the –OH group on choline tends to drag the charged end to depart from surfactant headgroups, which may adversely affect the screening effect. If this is the case, –OH yields a negative effect on micelle aggregation. However, –OH as an H-donor also forms a intermolecular hydrogen bond with a carboxyl group49 and here a hydrogen bond should also readily be formed between choline and the carboxyl group of NaOEr, which enhances the interaction between the choline molecules and surfactants.50 Thus choline is tightly bound to the headgroup of NaOEr, and the electrostatic screening is enhanced due to this compact contact. Despite the presence of this hydrogen bond, several times CS relative to CD is required to obtain the maximum viscosity (Fig. 3A). By this observation, the screening is not sufficient only by those cholines bound via hydrogen bond at a stoichiometric ratio. Because the bulky volume of choline restricts the proximity of its charged centre to the headgroups of surfactant, their screening efficiency is thus weakened,51 and the remaining choline molecules play only a supplementary role in the screening. For TMA+ system, there is no additional avenue for electrostatic interaction yet it possesses a similar steric hindrance as the choline system.45 As a result, TMA+ is less effective than choline in favoring micelle morphology.
Enhanced sensitivity of micelles for the choline–NaOEr system to temperature
The difference in the structure of the counter ions also has an impact on the activation energy of micelles. A relatively high Ea value reflects the strong dependence of viscosity on temperature while a low Ea suggests the micelles formed are more stable.13 Hydrogen bonds (5–30 kJ mol−1) are stronger than van der Waals interactions,52,53 but weaker than covalent or ionic bonds, and are gradually destroyed with increasing temperature. At low temperature, the influence of temperature on hydrogen bonding is negligible but upon heating the motion of choline molecules is enhanced, and bound cholines tend to desorb from the micelles. Thus not only the benefit for hydrogen bonding to micelle growth disappears, but also the hydrophilicity of choline repels it from the surfactant. This factor must be considered for all the choline molecules, i.e., including those bound by hydrogen bonds and free molecules. As a result, the electrostatic screening effect from the choline molecules is reduced and is more severe with increasing choline composition. Accordingly, with increasing temperature, the micelles will become unstable, and this instability will be intensified at higher choline concentration. This explains the increasing Ea with choline concentration and that the choline system is more sensitive to temperature than TMA+. Note that these two key factors for choline, i.e., hydrogen bonding and hydrophilicity, both arise from the –OH group. The mechanism interpreting the effects of concentration and temperature is illustrated in Fig. 7.
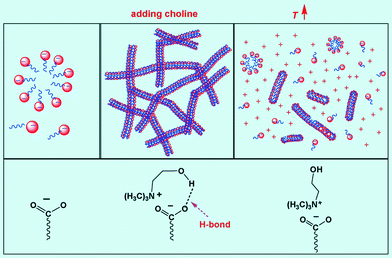 |
| Fig. 7 Schematic illustration of the influence of temperature on the interactions between NaOEr and choline. | |
Another possible explanation on the difference of Ea between choline and TMA+ could be related to interactions among the micelles. According to Fischer et al.,54 the activation energy describes the energy necessary to move individual micelles in an environment of surrounding micelles and therefore, it is determined by the interactions among individual aggregates.40 At low TMA+ concentration, the micelles are rigid due to poor screening and so interaction and fusing with surrounding micelles is difficult. Their movements are constrained by entanglements with other wormlike micelles and therefore a high Ea is found. At intermediate TMA+ concentration, the wormlike micelles are imparted with greater flexibility by thorough screening and they can fuse, break and reform readily. At high TMA+ content, branched micelles are formed and the cross-links can slide along the micelles easily. With increasing TMA+ concentration, the progressively increased mobility results in decreased Ea. A conclusion is reached that the screening to net charges plays a key role on Ea variation. This conclusion is also applicable to the choline system, although the variation of Ea to choline concentration is almost opposite to that of the TMA+ system.
At low and intermediate choline contents, the Ea values show weak dependence on the composition, which is more or less consistent with the TMA+ system though values relative to the TMA+ system are lower resulting from the contribution of hydrogen bonding to screening. At high choline concentration, however, Ea shows a significant increase. Due to the bulky volume of choline, the electrostatic screening between equimolar choline and NaOEr connected by hydrogen bonding is not sufficient. According to Fig. 3A, upon further adding choline above a ratio of CS/CD ∼ 1, the value of η0 increases. –OH groups on the added choline ions cannot penetrate into the micellar interior but project outside of the micelle surface owing to their hydrophilicity. Thus interactions among neighboring micelles is enhanced by hydrogen bonds formed by such hydroxyl groups. Under such conditions, it becomes more difficult for one micelle to break free from the surrounding micelles and therefore Ea is increased.
Above CS/CD ∼ 4, the choline system becomes more sensitive to temperature than the TMA+ system; additionally, just above the same ratio of CS/CD, NaOEr can be solubilised below 37 °C (see Fig, S1, ESI†), corresponding to normal body temperature. Therefore we are inspired that by fine adjustment of the composition of choline or temperature, we can manipulate the micelle morphology and this may find potential application in drug release.
Conclusions
Choline is introduced to induce NaOEr to form strong viscoelastic wormlike micelles for the first time. By comparing the different influences of adding choline and TMAB, some conclusions can be drawn. First, choline is much more biodegradable than TMA+. Considering that NaOEr is prepared from biodegradable erucic acid, the choline–NaOEr wormlike system can be regarded as environmentally friendly. Choline carboxylates have already been reported55,56 as ingredients of certain formulations, for example, cosmetic products or cryoprotectant agents for plants. Second, choline shows a stronger ability to favor micelle growth. The enhanced electrostatic effects via hydrogen bonding as a result of –OH on choline plays a vital role. Third, the choline system exhibits enhanced sensitivity to temperature with increasing hydrotrope concentration. When exposed to high temperature, the hydrogen bonding breaks down and the screening disappears. Moreover, the increased hydrophilicity from released –OH will make the counterion unfavorable to approach the headgroup. Therefore, the choline system will be more sensitive to temperature than TMA+ system, and an appropriate choice of the hydrotrope can tune the performances of the formed wormlike micelles, rendering possible specific applications such as drug release.
Acknowledgements
Y. F. acknowledges the financial support from Shandong Provincial government through the “Taishan Scholar” project, Natural Science Foundation of China (No. 21173207), Sichuan Provincial Bureau of Science and Technology (No. 2010JQ0029 and No. 2012NZ0006), as well as the Key Laboratory for Colloid and Interface Chemistry of the State Education Ministry at Shandong University (No. 200601). Y. H. thanks the financial support from Natural Science Foundation of China (No. 60979020 and No. 60939001).
References
- C. A. Dreiss, Soft Matter, 2007, 3, 956–970 RSC.
- S. Kefi, J. Lee, T. L. Pope, P. Sullivan, E. Nelson, A. N. Hernandez, T. Olsen, M. Parlar, B. Powers, A. Roy, A. Wilson and A. Twynam, Oilfield Rev., 2004, 16, 10–23 CAS.
- A. Watkins, Inform, 2009, 11, 682–685 Search PubMed.
- X. P. Li, L. Yu, Y. Q. Ji, B. Wu, G. Z. Li and L. Q. Zheng, Chin. Chem. Lett., 2009, 20, 1251–1254 CrossRef CAS.
- P. Dalhaimer, A. J. Engler, R. Parthasarathy and D. E. Discher, Biomacromolecules, 2004, 5, 1714–1719 CrossRef CAS.
- I. M. Banat, Bioresour. Technol., 1995, 51, 1–12 CrossRef CAS.
- W. de Jong and P. Borm, Int. J. Nanomed., 2008, 3, 133–149 CrossRef CAS.
- N. Vlachy, C. Merle, D. Touraud, J. Schmidt, Y. Talmon, J. Heilmann and W. Kunz, Langmuir, 2008, 24, 9983–9988 CrossRef CAS.
- G. C. Kalur and S. R. Raghavan, J. Phys. Chem. B, 2005, 109, 8599–8604 CrossRef CAS.
- K. Mannhardt, L. L. Schramm and J. J. Novosa, Colloids Surf., 1992, 68, 37–53 CrossRef CAS.
-
L. L. Wesson and J. H. Harwell, in Surfactants: Fundamentals and Applications in the Petroleum Industry, ed. L. L. Schramm, Cambridge University Press, 2000, pp. 121–158 Search PubMed.
- A. Khatory, F. Kern, F. Lequeux, J. Appell, G. Porte, N. Morie, A. Ott and W. Urbach, Langmuir, 1993, 9, 933–939 CrossRef CAS.
- P. A. Hassan, S. J. Candau, F. Kern and C. Manohar, Langmuir, 1998, 14, 6025–6029 CrossRef CAS.
- M. E. Cates and S. J. Candau, J. Phys.: Condens. Matter, 1990, 2, 6869–6892 CrossRef CAS.
- P. A. Hassan, S. R. Raghavan and E. W. Kaler, Langmuir, 2002, 18, 2543–2548 CrossRef CAS.
- S. Kumar, S. L. David, V. K. Aswal, P. S. Goyal and Kabir-ud-Din, Langmuir, 1997, 13, 6461–6464 CrossRef CAS.
- L. Ziserman, L. Abezgauz, O. Ramon, S. R. Raghavan and D. Danino, Langmuir, 2009, 25, 10483–10489 CrossRef CAS.
- L. J. Magid, J. Phys. Chem. B, 1998, 102, 4064–4074 CrossRef CAS.
- K. Nakamura and T. Shikata, Langmuir, 2006, 22, 9853–9859 CrossRef CAS.
- S. R. Raghavan and E. W. Kaler, Langmuir, 2001, 17, 300–306 CrossRef CAS.
- V. Croce, T. Cosgrove, C. A. Dreiss, S. King, G. Maitland and T. Hughes, Langmuir, 2005, 21, 6762–6768 CrossRef CAS.
- R. Kumar, G. C. Kalur, L. Ziserman, D. Danino and S. R. Raghavan, Langmuir, 2007, 23, 12849–12856 CrossRef CAS.
- Z. Chu, Y. Feng, X. Su and Y. Han, Langmuir, 2010, 26, 7783–7791 CrossRef CAS.
- Z. Chu and Y. Feng, Soft Matter, 2010, 6, 6065–6067 RSC.
- Z. Chu, Y. Feng, H. Sun, Z. Li, X. Song, Y. Han and H. Wang, Soft Matter, 2011, 7, 4485–4489 RSC.
- Z. Chu and Y. Feng, Chem. Commun., 2010, 46, 9028–9030 RSC.
- Y. Han, Y. Feng, H. Sun, Z. Li, Y. Han and H. Wang, J. Phys. Chem. B, 2011, 115, 6893–6902 CAS.
-
A. W. Hayes, Principles and Methods of Toxicology, Taylor and Francis, Boston, 5th edn, 2007 Search PubMed.
-
J. Steber and H. Berger, in Biodegradability of Surfactants, ed. D. R. Karsa and M. R. Porter, Blackie Academic & Professional, Glasgow, UK. 1995 Search PubMed.
-
J. Dewulf and H. V. Langenhove, Renewables-Based Technology: Sustainability Assessment, Wiley-VCH, New York, 2006 Search PubMed.
- R. I. Murahata and M. P. Aronson, J. Soc. Cosmet. Chem., 1994, 45, 239–246 CAS.
-
L. Dussault and A. Caudet, US Pat., 5 496 489, 1996 Search PubMed.
- T. Hrobárik, L. Vrbka and P. Jungwirth, Biophys. Chem., 2006, 124, 238–242 CrossRef.
- E. Kutluay, B. Roux and L. Heginbotham, Biophys. J., 2005, 88, 1018–1029 CrossRef CAS.
- L. C. Mokrasch, Mol. Cell. Biochem., 1990, 92, 85–89 CrossRef CAS.
- W. H. Meck and C. L. Williams, Dev. Brain Res., 1999, 118, 51–59 CrossRef CAS.
- J. K. Blusztajn, Science, 1998, 281, 794–795 CrossRef CAS.
- M. G. de Mul, H. T. Davis, D. F. Evans, A. V. Bhave and J. R. Wagner, Langmuir, 2000, 16, 8276–8284 CrossRef CAS.
-
J. N. Israelachvili, Intermolecular and Surface Forces, Academic Press, San Diego, CA, 1991 Search PubMed.
- V. Croce, T. Cosgrove, G. Maitland, T. Hughes and G. Karlsson, Langmuir, 2003, 19, 8536–8541 CrossRef CAS.
- P. A. Hassan and J. V. Yakhmi, Langmuir, 2000, 16, 7187–7191 CrossRef CAS.
- A. Khatory, F. Lequeux, F. Kern and S. J. Candau, Langmuir, 1993, 9, 1456–1464 CrossRef CAS.
- R. Granek and M. E. Cates, J. Chem. Phys., 1992, 96, 4758–4767 CrossRef CAS.
- F. Kern, R. Zana and S. J. Candau, Langmuir, 1991, 7, 1344–1351 CrossRef CAS.
- R. Klein, D. Touraud and W. Kunz, Green Chem., 2008, 10, 433–435 RSC.
- D. P. Acharya, D. Varade and K. Aramaki, J. Colloid Interface Sci., 2007, 315, 330–336 CrossRef CAS.
- R. Oda, L. Bourdieu and M. Schmutz, J. Phys. Chem. B, 1997, 101, 5913–5916 CrossRef CAS.
- R. Abdel-Rahem, Adv. Colloid Interface Sci., 2008, 141, 24–36 CrossRef CAS.
- B. Novales, L. Navailles, M. Axelos, F. Nallet and J. P. Douliez, Langmuir, 2008, 24, 62–68 CrossRef CAS.
- X. Pei, J. Zhao and X. Wei, J. Colloid Interface Sci., 2011, 356, 176–181 CrossRef CAS.
- S. Kumar, A. Z. Naqvi, V. K. Aswal, P. S. Goyal and Kabir-ud-Din, Curr. Sci., 2003, 84, 1346–1349 CAS.
- J. Emsley, Chem. Soc. Rev., 1980, 9, 91–124 RSC.
- O. Markovitch and N. Agmon, J. Phys. Chem. A, 2007, 111, 2253–2256 CrossRef CAS.
- P. Fischer and H. Rehage, Langmuir, 1997, 13, 7012–7020 CrossRef CAS.
-
S. Nayak and V. Nayak, US Pat., 6 120 779, 2000 Search PubMed.
-
J. R. Geary, US Pat., 5 124 061, 1992 Search PubMed.
Footnote |
† Electronic supplementary information (ESI) available. See DOI: 10.1039/c2ra20136d |
|
This journal is © The Royal Society of Chemistry 2012 |