DOI:
10.1039/C2RA00905F
(Communication)
RSC Adv., 2012,
2, 812-815
Enhancement of electron field emission properties of TiO2−x nanoplatelets by N-doping
Received
17th October 2011
, Accepted 19th November 2011
First published on 12th December 2011
Abstract
Thin films of TiO2−x and TiO2−x−yN2y nano-platelets having better field emission properties compared to one dimensional nanostructures reported in literature were synthesized by spray pyrolysis. Low threshold field of 8 V μm−1 and 4.1 V μm−1, for 1 mA cm2, was observed for TiO2−x and TiO2−x−yN2y respectively, implying enhanced field emission upon N-doping.
1. Introduction
TiO2 exhibits outstanding chemical stability and is of great interest in applications such as photovoltaics, photocatalysis, self cleaning coatings and sensors.1–3 Most of the research activities are directed towards the synthesis of pure and doped one dimensional TiO2 nanoarchitectures for applications in photocatalysis and photovoltaics. The field emission (FE) properties of TiO2 were rarely investigated prior to 2004, though it shows a low work function (4.5 eV) compared to other popular FE materials such as ZnO (5.3 eV), ZnS (7.0 eV) etc.4 The important factors for high emission currents at low applied fields are low work function and high aspect ratio microstructure.5,6 Taking this into account, one dimensional nanoarchitectures of TiO2 were synthesized and their field emission properties were studied by several groups.7–13 Field emission properties of aligned single crystalline TiO2 nanowires grown on Ti, Si and Au doped TiO2/Si substrates as well as those of TiO2 nanotube arrays are reported in ref. 7–9 and 10–13 respectively. Compared to TiO2, Miyauchi et al.,10 Liu et al.,12 and Wang et al.13 observed enhancement in FE properties in TiO2−x, N-doped TiO2 and Fe-doped TiO2 respectively. Developing emitters with low turn on field and high current density, at low fabrication cost is challenging but desired. To the best of our knowledge FE studies of TiO2 and N-doped TiO2 two dimensional nanostructures are rare. The single step synthesis of N-doped TiO2 powders by an open atmosphere pyrolysis technique and their visible light photocatalytic properties have been reported by Liet al.3 In the present study, we report the synthesis of aligned TiO2−x and N-doped TiO2−x nanoplatelets in thin film form by a simple, cost effective ultrasonic spray pyrolysis technique and their electron field emission properties. X-Ray photoelectron spectroscopy was used to understand the electronic structure responsible for the enhanced field emission. The effect of oxygen deficiency and N-doping on the FE properties is explained.
2. Experimental
Self standing TiO2 triangular nanoplatelets were synthesized by an ultrasonic spray pyrolysis technique. In this technique, 0.5 to 3 μm size aerosols of titanium oxy-acetyl acetonate dissolved in methanol (0.05 M) generated with an ultrasonic nebulizer are directed onto the Si(100) substrate fixed on a flat heater maintained at 500 °C, through a quartz column, using air as the carrier gas. On reaching the hot zone the aerosols underwent solvent evaporation followed by solute sublimation. On reaching the substrate the solute vapors decomposed reactively to give TiO2−x thin films. For the synthesis of N-doped TiO2, a titanium oxy-acetyl acetonate and hexamethylene tetramine (HMTA) mixture dissolved in methanol was used as the precursor.
3. Results and discussion
GIXRD and micro Raman spectroscopy revealed that both TiO2 and N-doped TiO2 nanoplatelets are of anatase phase (Fig. 1). The ratio of the intensities of diffraction, I[101]/I[200], was found to be 2.77 and 6.18 for TiO2 and N-doped TiO2 respectively, implying more preferred (101) orientation in N-doped TiO2. The Raman spectra corresponding to pure and doped films show an intense band at 143 cm−1 (Eg(1)), a weak band at 198 cm−1 (Eg(2)) and three medium intensity bands at 397 cm−1 (B1g(1)), 514 cm−1 (A1g+ B1g(2)) and 635 cm−1 (Eg(3)) in agreement with the reported values of the anatase phase.14FESEM images suggest that the pure and N-doped TiO2 films (Fig. 2a and 2b) consist of densely packed self standing triangular nanoplatelets of ∼10 to 15 nm thickness and ∼90 and ∼110 nm side lengths for pure and doped films respectively.
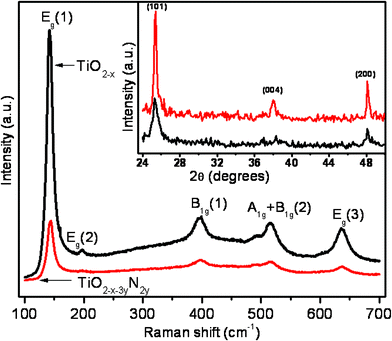 |
| Fig. 1
Raman spectra of TiO2−x and N-doped TiO2−x nanoplatelets, inset shows the corresponding XRD pattern. | |
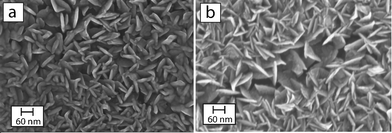 |
| Fig. 2
FESEM images of (a) TiO2−x nanoplatelets and (b) N-doped TiO2−x nanoplatelets, depicting self standing triangular nanoplatelets, image taken near a deliberately damaged area, to visualize the shape and orientation of the nanoplatelets. | |
The chemical states of the various species present in the films were examined with XPS. The binding energies obtained are corrected for specimen charging by referencing the observed C 1s binding energy to 284.5 eV. The Ti 2p3/2 binding energies of pure and N-doped TiO2 nanoplatelets, obtained from XPS, are 459.4 and 459.2 eV respectively, as shown in Fig. 3a. Shoulders at 457.5 eV for pure and 457.3 eV for N-doped TiO2 indicate the presence of Ti having a lower oxidation state (+3). Because of the presence of reducing species (C and H) in the precursor, titanium oxide synthesized under the present experimental conditions is likely to be oxygen deficient (TiO2−x). To maintain charge neutrality the presence of oxygen vacancies will lead to the formation of lower valent Ti cations.
In the case of N-doped TiO2, when two N atoms are incorporated one oxygen vacancy is created in addition to the oxygen vacancies created because of the presence of reducing species in the precursor. The small red shift in the Ti 2p binding energies of N-doped TiO2 with respect to TiO2−x can be attributed to the increase in electron cloud density on Ti because of the presence of nitrogen in the anion sub-lattice, which has lower electronegativity compared to oxygen. The N 1s spectrum of N-doped TiO2 was recorded from the surface and after sputtering for 30, 60 and 90 s. Since not much change in peak intensity was observed between the spectra recorded after 60 and 90 s of sputtering, the one after sputtering for 90 s is taken and is given in Fig. 3b. The peak position (396.9 eV) corresponds to β-N, which is directly bonded to the central Ti cation.15 The formula of N-doped TiO2 can therefore be written as TiO2−x−yN2y. The nitrogen content estimated from the peak area and sensitivity factor using the equation:
XN = (IN/SN)/[(ITi2p/STi) + (IO/SO) + (IN/SN)] |
is 3.7%, where
IN is the peak area of the N 1s peak corresponding to β-N,
ITi2p is the sum of the areas of the Ti
2p deconvoluted peaks and
IO is the area of the O 1s peak corresponding to bonded oxygen.
SN,
STi and
SO are the sensitivity factors of
nitrogen, titanium and oxygen respectively.
Field emission measurements were carried out in a home built tunable parallel plate capacitor setup, in a high vacuum chamber at 10−7 m bar. A molybdenum rod of 3 mm diameter with a mirror polished flat surface was used as the anode. The separation between the flat surface and the film was measured using a digital micrometer and optical microscope. The variation of FE current density with electric field for TiO2−x and TiO2−x−yN2y films is shown in Fig. 4. The turn on field, defined as the extraction field at 1 mA cm−2, is estimated to be 8.0 V μm−1 for TiO2−x and 4.1 V μm−1 for TiO2−x−yN2y nanoplatelets. The threshold field corresponding to an emission current density of 10 mA cm−2 is 10 and 6 V μm−1 for TiO2−x and TiO2−x−yN2y respectively. These clearly depict the enhancement of FE properties, of TiO2−x, on N-doping.
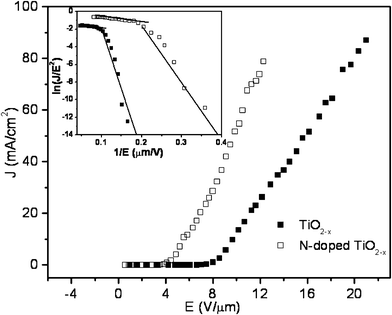 |
| Fig. 4 Field emission characteristics of pure TiO2−x and N-doped TiO2−x nanoplatelets. Inset shows the F–N plot of pure TiO2−x and N-doped TiO2−x nanoplatelets. | |
The FE properties can be analyzed by the Fowler–Nordheim model16 given by the equation:
ln(J/E2) = ln(Aβ2/Φ) + (−BΦ3/2/β)(1/E) |
where
J is the current density,
E is the applied electric field,
Φ is the work function and
β is the field enhancement factor associated with the magnitude of the electric field at the emitting surface and is given by
β =
Elocal/
E, where
Elocal is the local electric field at the emitting surface.
17A and
B are constants with values of 1.54 × 10
−6 (AeV V
−2) and 6.83 × 10
9 (V m
−1eV
−3/2) respectively. The work function
Φ of TiO
2 is 4.5 eV.
18 Once
Φ is known,
β can be calculated from the slope of a plot of ln(
J/
E2)
versus 1/
E (inset of
Fig. 4). The F–N plot depicts two slopes, one at high and the other at low field. Different slopes are related to the electron emission at different states where electrons can only be emitted beyond their critical fields.
12 The calculated high and low field slopes for TiO
2−x are −6.74 ± 0.87 corresponding to
β = 10
![[thin space (1/6-em)]](https://www.rsc.org/images/entities/char_2009.gif)
997, and −180 ± 26 corresponding to
β = 356, respectively. For TiO
2−x−yN
2y the high and low field slopes are −5.68 ± 0.36 corresponding to
β = 11
![[thin space (1/6-em)]](https://www.rsc.org/images/entities/char_2009.gif)
563, and −60.87 ± 8.23 corresponding to
β = 1071, respectively. From this we can infer that TiO
2−x−yN
2y has large
β values compared to TiO
2−x. A comparison of the threshold fields at 1 mA cm
−2 obtained in the present study with those reported in the literature is tabulated in
Table 1. The
extraction field of 4.1 V μm
−1 is much lower compared to reported values. It is interesting to note that the FE performance of aligned TiO
2−x triangular nanoplatelets synthesized by the pyrolysis technique is superior to vertically aligned wires
7,8 and
nanotubes10–13 though the one-dimensional architectures are more favorable for FE. Hence, the properties determined by electronic structure modification may be responsible for the enhanced FE.
Table 1 Comparison of extraction field corresponding to a field emission current density of 1 mA cm−2 (present study and those calculated from the literature)
It is well known that in TiO2−x, oxygen vacancies form localized donor states 0.75–1.18 eV below the conduction band minimum (CBM).19,20 In the present study the existence of oxygen vacancies and related Ti3+ in TiO2−x was confirmed by XPS. In the case of TiO2−x−yN2y, compared to TiO2−x, there are additional oxygen vacancies due to N-doping. This increases the band width of the localized donor states, and the energy gap between the localized donor states and CBM reduces to values below 0.75 eV (0.75 − Δ). It has been shown from experimental results and first-principle calculations that substitution of oxygen by nitrogen atoms in TiO2 creates isolated localized N 2p state above the O 2p dominant valence band.21,22 From photoelectrochemical studies it has been shown that the localized N 2p state is located at ∼0.75 eV above the valence band maximum (VBM).23 The presence of localized donor states located at (0.75 − Δ) eV below the CBM and acceptor state at ∼0.75 eV above the VBM will shift the Fermi level to higher energies compared to TiO2−x. Since electron emission from a material into a vacuum under the influence of an electric field takes place by the tunneling of electrons lying below the Fermi level through the potential barrier24 the rise in the position of the Fermi level will improve the FE properties as well as lower the work function.
Conclusions
In summary, self standing triangular nanoplatelets of anatase TiO2−x and TiO2−x−yN2y with enhanced FE properties compared to the reported ones were synthesized using a simple ultrasonic spray pyrolysis technique. The enhanced FE properties observed in TiO2−x are attributed to the presence of oxygen vacancies. The field emission properties were further enhanced by N-doping and this is related to the presence of localized donor states, with higher band width, located at energy levels close to CBM compared to that of TiO2−x and an acceptor state above the VBM leading to a shift in Fermi level to higher energies. It should be noted that, in view of adaptability for large scale production and large surface area coating, the spray pyrolysis technique is simple, cost effective and efficient compared to the techniques used for the fabrication of any one dimensional nanoarchitectures.
Acknowledgements
The authors thank Dr C. S. Sundar, Director, Materials Science Group, for the interest and sustained encouragement throughout the course of this work.
References
- K. Y. Cheung, C. T. Yip, A. B. Djurisic, Y. H. Leung and W. K. Chan, Adv. Funct. Mater., 2007, 17, 555 CrossRef CAS.
- O. K. Varghese and C. A. Grimes, J. Nanosci. Nanotechnol., 2003, 3, 277 CrossRef CAS.
- D. Li, H. Haneda, S. Hishita and N. Ohashi, Mater. Sci. Eng., B, 2005, 117, 67 CrossRef.
- X. S. Fang, Y. Bando, G. Z. Shen, C. H. Ye, U. K. Gautam, P. M. F. J. Costa, C. Y. Zhi, C. C. Tang and D. Golberg, Adv. Mater., 2007, 19, 2593 CrossRef CAS.
- T. Sugino, T. Hori, C. Kimura and T. Yamamoto, Appl. Phys. Lett., 2001, 78, 3229 CrossRef CAS.
- J. Zhou, S. Z. Deng, N. S. Xu, J. Chen and J. C. She, Appl. Phys. Lett., 2003, 83, 2653 CrossRef CAS.
- K. Huo, X. Zhang, J. Fu, G. Qian, Y. Xin, B. Zhu, H. Ni and P. K. Chu, J. Nanosci. Nanotechnol., 2009, 9, 3341 CrossRef CAS.
- B. Xiang, Y. Zhang, Z. Wang, X. H. Luo, Y. W. Zhu, H. Z. Zhang and D. P. Yu, J. Phys. D: Appl. Phys., 2005, 38, 1152 CrossRef CAS.
- J. M. Wu, H. C. Shih and W. T. Wu, Chem. Phys. Lett., 2005, 413, 490 CrossRef CAS.
- M. Miyauchi, H. Tokudome, Y. Toda, T. Kamiya and H. Hosono, Appl. Phys. Lett., 2006, 89, 43114 CrossRef.
- J. B. Chen, C. W. Wang, B. H. Ma, Y. Li, J. Wang, R. S. Guo and W. M. Liu, Thin Solid Films, 2009, 517, 4390 CrossRef CAS.
- G. Liu, F. Li, D. W. Wang, D. M. Tang, C. Liu, X. Ma, G. Qing Lu and H. M. Cheng, Nanotechnology, 2008, 19, 025606 CrossRef.
- C. C. Wang, K. W. Wang and T. P. Perng, Appl. Phys. Lett., 2010, 96, 143102 CrossRef.
- A. C. Ferrari and J. Robertson, Phys. Rev. B: Condens. Matter, 2001, 64, 075414 CrossRef.
- R. Asahi, T. Morikawa, T. Ohwaki, K. Aoki and Y. Taga, Science, 2001, 293, 269 CrossRef CAS.
- R. H. Fowler and L. W. Nordheim, Proc. R. Soc. London, Ser. A, 1928, 119, 173 CrossRef CAS.
- S. Das, S. F. Ahmed, M. K. Mitra and K. K. Chottopadhyay, Appl. Phys. A: Mater. Sci. Process., 2008, 91, 429 CrossRef CAS.
- J. Pascual, J. Camassel and H. Mathieu, Phys. Rev. B, 1978, 18, 5606 CrossRef CAS.
- I. Justicia, P. Ordejon, G. Canto, J. L. Mojos, J. Fraxedas, G. A. Battiston, R. Gerbasi and A. Figueras, Adv. Mater., 2002, 14, 1399 CrossRef CAS.
- T. Ihara, M. Miyoshi, Y. Iriyama, O. Matsmoto and S. sugihara, Appl. Catal., B, 2003, 42, 403 CrossRef CAS.
- Z. Lin, A. Orlov, R. M. Lambert and M. C. Payne, J. Phys. Chem. B, 2005, 109, 20948 CrossRef CAS.
- C. D. Valentin, G. Pacchioni and A. Selloni, Phys. Rev. B: Condens. Matter Mater. Phys., 2004, 70, 085116 CrossRef.
- R. Nakamura, T. Tanaka and Y. Nakato, J. Phys. Chem. B, 2004, 108, 10617 CrossRef CAS.
- E. L. Murphy and R. H. Good, Phys. Rev., 1956, 102, 1464 CrossRef CAS.
|
This journal is © The Royal Society of Chemistry 2012 |