DOI:
10.1039/C1RA01096D
(Paper)
RSC Adv., 2012,
2, 1856-1862
Effective recovery of some precious metals by using alternative quaternary amine types of anion-exchangers prepared from biofuel-extracted microalgal residues
Received
15th November 2011
, Accepted 17th November 2011
First published on 4th January 2012
Abstract
Trimethylamine- and triethylamine-immobilized adsorption gels were prepared from the oil-extracted microalgal residues. The FT/IR spectra and elemental analysis confirmed the immobilization of quaternary amine functional groups on the microalgal residue. Their adsorption behaviors for various metal ions in a hydrochloric acid solution were studied. Both adsorbents exhibited high selectivity for Pd(II) and Pt(IV) while showing no significant affinity for base metal ions. From the adsorption isotherms, the adsorption capacities for Pd(II) and Pt(IV) in 0.1 M HCl was found to be 2.95 and 1.33 mmol g−1 for trimethylamine, and 2.57 and 1.54 mmol g−1 for triethylamine type gels, respectively. These adsorbents are promising for the selective separation and recovery of Pd(II) and Pt(IV) and directly provide the sustainable means for the material development and benign environment.
Introduction
Presently, the global supply of liquid fuels is largely dependent on fossil fuels, which are limited for use in the next few decades. Furthermore, due to rapidly increasing fuel prices, as well as environmental and resource concerns, the exploration of new natural feedstocks for producing alternative fuels, especially renewable photosynthetic organisms, is being carried out extensively.1–3 Among various kinds of renewable photosynthetic organisms, microalgae has been expected to be a potential resource for the production of biofuel in place of petroleum due to their renewable properties, fast growing rate, high oil content, and CO2 sequestration benefit. Microalgae commonly produce carbohydrates and proteins as their cell components in addition to lipids, which is extracted to produce biofuel. During the extraction of such biofuel, large amounts of solid microalgal biomass residues consisting of carbohydrates and proteins are generated as by-products. However, the good option for their further utilization has not been established, which causes this residual biomass to be considered as waste requiring high disposal costs. From this context, our efforts have been made to reclaim some values from such residual biomass in the form of biomass based advanced functional materials by the effective use of their unique characteristics.
At the same time, with the growing fuel crisis, recycling and recovery of various metals from spent electronic appliances is an emerging research subject. This is due to the acute limitation of metal resources along with the fast growing rate of the development of advanced materials and devices such as cell phones, televisions, computers, etc.4,5 The recovery of valuable metals from such appliances is greatly beneficial from both an environmental and economic viewpoint. This is because the recycling of these metals reduces the cost of metallurgical steps associated with their mining activity, and sharply decreases the destruction to the environment. In the hydrometallurgical process of precious metal recovery, solvent extraction and electrochemical processes have been employed for recovery in case their concentrations are high enough.6 In contrast, in the case of solutions containing trace concentrations of precious metals, ion-exchange and adsorption techniques are more suitable for their recovery.7,8 The commercially available ion-exchange resins used in the metal separation processes are based on polymeric petrochemical products. In order to establish a sustainable technology, solvents and materials produced from petroleum should be replaced by environmental benign alternatives produced from naturally available biomaterials or biomasses.
In recent years, biomass waste has been used as a potential alternative for the separation and recovery of toxic and precious metal ions from industrial solutions.9 As unique adsorbents, high selectivity to a targeted metal ion is important, and the selection of a suitable ligand is essential for the design of specific adsorbents. To meet such requirements, one approach is to take the advantage of the immobilization of active functional groups onto the biomass matrices, so as to enhance the affinity as well as the selectivity of the original material. Consequently, a number of chemical modifications of biomasses and biomaterials have been reported in the literature.10–15
In our previous study, we developed some strongly basic adsorbents by immobilizing quaternary amine functional groups onto polymer matrices of lignophenol, which was prepared by interacting phenol with lignin in wood waste.16 These were found to be effective for adsorption of Pd(II) and Pt(IV) in hydrochloric acid solution. In this work, two types of strong base anionic adsorbents have been prepared from the residual waste of Pseudochoricystis ellipsoidea, which was generated in the extraction process of biofuel, by chemical modification with trimethylamine and triethylamine. In the same manner with the modification of cellulose in waste paper,17 these functional groups were introduced onto polymer matrices of cellulosic polysaccharides in microalgal residues giving strong base anion-exchange materials. The characteristics and adsorption behaviors of prepared adsorbents including selectivity, adsorption kinetics, adsorption isotherms, for precious and base metals in acidic chloride media were investigated.
Experimental
Raw material and reagents
A dry microalgal residue of Pseudochoricystis ellipsoidea obtained after the extraction of biofuel was provided by DENSO CORPORATION, Aichi, Japan, as a raw material for the preparation of adsorbents. Thionyl chloride (SOCl2) was purchased from Sigma-Aldrich, St Louis, MO, USA. Trimethylammonium chloride and triethylaminnonium chloride were purchased from Wako, Osaka, Japan. Analytical grade H2PtCl6·6H2O, PdCl2, RhCl3·3H2O, IrCl3·xH2O, FeCl3·6H2O (Wako, Osaka, Japan), ZnCl2 (Sigma-Aldrich, St Louis, MO, USA), CuCl2, and NiCl2·6H2O (Katayama Chemicals, Osaka, Japan) were used to prepare Pd(II), Pt(IV), Rh(III), Ir(III), Fe(III), Zn(II), Cu(II), and Ni(II) test solutions, respectively. The ion-exchange resins; DIAION SA11A and PA312, were kindly donated by Mitsubishi Chemical Corporation, Tokyo, Japan. All other chemicals used for the preparation of the adsorbent and for the adsorption tests were of analytical grade and were used without further purification.
Preparation of adsorbents
The microalgal residue employed in this work comprised polysaccharides and polypeptides as its main components. This biomass residue was interacted with SOCl2, for chlorination and subsequent amination with quaternary amine functional groups, to pursue the modification of this microalgal residue, as depicted in Scheme 1. In the typical preparation process, 5 g of dry microalgal residue was taken together with 200 mL pyridine in a 500 mL three-necked flask, which was kept in an ice bath. Thirty millilitres of SOCl2 was added dropwise to the mixture under a N2 atmosphere and it was further stirred at 70 °C for 5 h to obtain the intermediate product for further treatment. After filtration, the intermediate product was washed with distilled water and dried in a convection oven at 70 °C overnight. To immobilize the functional groups of trimethylamine, 5 g of this intermediate product was dispersed in 200 mL of ethanol, to which 100 mL trimethylamine was added under stirring. The mixture was further stirred at 65 °C for 4 days to obtain the quaternary amine-modified microalgal residue. After the mixture was allowed to cool down at room temperature, the final product was collected by filtration and washed with distilled water until a neutral pH was obtained. Finally, the product was dried in a convection oven at 70 °C for 24 h. The product was ground and sieved to uniform particle size ranging from 100 to 150 μm prior to the adsorption study. The microalgal residue immobilized with trimethylamine thus prepared is hereafter abbreviated as TMA-microalgae. The triethylamine-immobilized microalgal residue was prepared by the same method as for TMA-microalgae but using triethylamine in place of trimethylamine, and this product is hereafter abbreviated as TEA-microalgae.
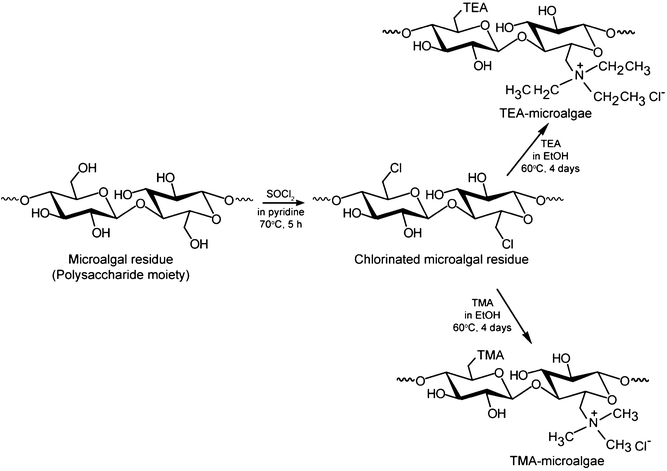 |
| Scheme 1 Preparation route of quaternary amine types of microalgal residue. | |
Characterizations
The Fourier-transform infrared (FT/IR) spectra of the microalgal residue and the adsorbent were recorded using a JASCO FT/IR-410 spectrophotometer (Tokyo, Japan). The C, H, and N contents were determined by elemental analysis. The surface morphology of the adsorbent was recorded using a JEOL (Tokyo, Japan) model JCM-5100 scanning electron microscope (SEM) at an acceleration energy of 20 kV. A Shimadzu model DTG 60H simultaneous DTA-TG analyzer, operated at a heating rate of 10 °C min−1 and under N2 atmosphere, was used to analyze the thermal decomposition of adsorbents. The metal concentration in the test solutions was measured using a Shimadzu model ICP-8100 inductively coupled plasma atomic emission spectrometer (ICP/AES).
The adsorption kinetics of Pd(II) and Pt(IV) were measured to evaluate the optimum time required to attain equilibrium, by shaking 50 mg of adsorbent in 50 mL of the test solution containing individual metal ions under constant temperature at a shaking speed of 150 rpm. A 2 mL aliquot of solution was taken at various time intervals and filtered immediately before measuring the metal concentration in the filtrate.
The adsorption tests for various metal ions, Pd(II), Pt(IV), Rh(III), Ir(III), Cu(II), Zn(II), Fe(III), and Ni(II), were carried out in batch mode to observe their adsorption behavior in varying concentrations of HCl from 0.1 to 5.0 M. This took into consideration that in commercial hydrometallurgical processes for precious metals, feed materials are treated in acidic chloride media such as aqua regia, and HCl containing chlorine. Ten milligrams of dried adsorbent and 10 mL of test solutions, containing mixed metal ions of 0.2 mM of Pd(II), Pt(IV) together with 1.0 mM of base metal ions, were mixed and shaken in a thermostated shaking incubator (THOMAS AT24R) at 30 °C, at 150 rpm for 48 h to attain equilibrium. Under the same conditions, DIAION SA11A and DIAION PA312, the commercially available anion-exchange resin containing quaternary ammonium groups produced and marketed by Mitsubishi Chemical Corporation, Japan, were tested for comparison. DIAION SA11A is classified as a gel type ion-exchange resin having a homogeneous matrix structure and micropores inside the beads, whereas DIAION PA312 has numerous macropores and micropores inside the beads.
The adsorption isotherms were measured to evaluate the maximum adsorption capacities for Pd(II) and Pt(IV), by shaking 10 mL of test solutions, ranged from 0.5 to 10 mM of individual metal ions in 0.1 M HCl, together with 10 mg of dried adsorbent for 4 days to attain adsorption equilibrium. The initial and remaining concentrations of metal ions in the filtrate were measured by the ICP/AES. The amount of adsorbed metal ions was calculated from the decrease in metal concentration in the filtrate and the dry weight of the adsorbent.
Results and discussion
Characteristics of prepared adsorbents
The recorded IR spectra of microalgal residue, TMA-microalgae, and TEA-microalgae, are shown in Fig. 1. The spectrum of the microalgal residue shows the presence of an O–H stretching vibration at 3313 cm−1 and C–H stretching vibrations at 2910 and 2842 cm−1. The sharp band at 1623 cm−1 indicates the presence of C
O groups. The IR fingerprints of the modified microalgal residue, TMA-microalgae and TEA-microalgae, display similar spectral patterns around 1038 cm−1. This represents the presence of a C–N stretching vibration, which indicates the immobilized quaternary amine moieties in the microalgal residue. The degree of immobilization of functional groups on the polymer matrices of the microalgal residue was also evaluated by means of elemental analysis of H, C, and N in the adsorbents. As shown in Table 1, the nitrogen content of the microalgal residue itself was evaluated as 4.04% or 2.89 mmol g−1, which is considered to exist as amino groups of proteins, one of the main components of this biomass residue. Although this microalgal residue contains a nitrogen moiety, it is not sufficient to facilitate the adsorption of precious metals such as Pd(II) and Pt(IV) on this biomass itself, as we reported in a previous study.18 In both modified microalgal residues, TMA-microalgae and TEA-microalgae, it is obvious that the nitrogen content was increased up to 4.29 and 4.08 mmol g−1, respectively. This result suggests the successful immobilization of quaternary amine functional groups on the microalgal residue. This immobilized nitrogen moiety on the microalgal residue is expected to play an important role in binding with precious metals like PdCl42− and PtCl62−.16
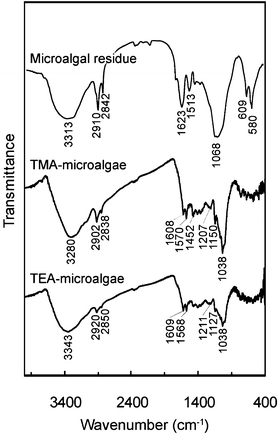 |
| Fig. 1 FT/IR spectra of microalgal residue and prepared adsorbents. | |
Table 1 Elemental content of adsorbents
|
Elemental content (%) |
N-density (mmol g−1) |
H |
C |
N |
Microalgal residue |
6.33 |
43.58 |
4.04 |
2.89 |
TMA-microalgae |
4.11 |
26.57 |
6.01 |
4.29 |
TEA-microalgae |
4.07 |
45.52 |
5.71 |
4.08 |
Fig. 2 shows the SEM images of TMA-microalgae and TEA-microalgae. As shown in this figure, the morphological surface of the prepared adsorbents was rather rough with random shape. In order to examine the thermal decomposition of the prepared adsorbents at elevated temperature, the thermal decomposition of TMA-microalgae and TEA-microalgae was investigated by thermogravimetric analysis. Fig. 3 shows the TG curves of TMA-microalgae and TEA-microalgae. It was obvious from this figure that the TG curves of both adsorbents consist of two main distinguished regions of weight loss or thermal decomposition. The weight loss in the first region below 200 °C corresponds to a volatilization process in which water molecules and light compounds were removed. It is suggested from the same figure that about 90% weight loss was observed between 200 and 600 °C for both TMA-microalgae and TEA-microalgae. This region corresponds to the liberation of volatile hydrocarbons of polysaccharide and protein moieties.19
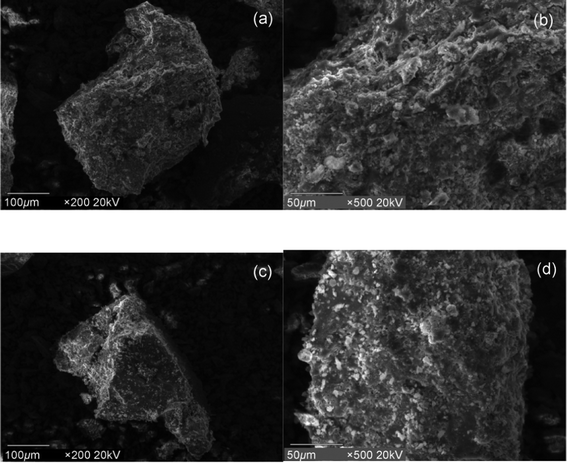 |
| Fig. 2
SEM images of adsorbents. | |
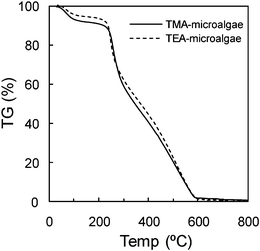 |
| Fig. 3
Thermogravimetric curves of adsorbents. | |
Effect of contact time on Pd(II) and Pt(IV) adsorption
Fig. 4(a) and (b) show the adsorption kinetics of Pd(II) and Pt(IV) on TMA-microalgae and TEA-microalgae, respectively, investigated at 30 °C in 0.1 M HCl. As the results show in these figures, the adsorption rate of Pd(II) and Pt(IV) was fast at the initial stage of adsorption, whereas a slow adsorption rate was observed after 12 h of contact time. From the plateau regions, the adsorption equilibrium of Pd(II) and Pt(IV) on TMA-microalgae and TEA-microalgae was attained within 48 h of shaking time. Hence, for further batch wise adsorption study, the test samples were shaken for 48 h to ensure equilibrium. The test samples for the measurement of adsorption isotherms were shaken for 4 days to attain equilibrium.
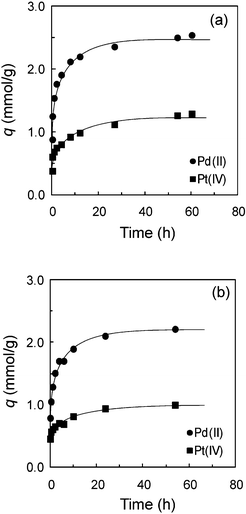 |
| Fig. 4 Adsorption kinetics of Pd(II) and Pt(IV) on (a) trimethylamine-microalgae and (b) triethylamine-microalgae. Conditions: concentration of metal ion = 2.2 mM, dry weight of adsorbent = 50 mg, volume of test solution = 50 mL, HCl concentration = 0.1 M, T = 30 °C. | |
Effect of HCl concentration on adsorption of metal ions
Fig. 5(a) and (b) show the % adsorption of Pd(II), Pt(IV), Rh(III), Ir(III), Zn(II), Cu(II), Ni(II), and Fe(III) on TMA-microalgae and TEA-microalgae, respectively, at varying HCl concentrations. Similar adsorption behaviors were observed for both TMA-microalgae and TEA-microalgae. The adsorption of Pd(II) on TMA-microalgae and TEA-microalgae was nearly quantitative over the whole HCl concentration region, which ranged from 0.1 to 5 M. However, a gradually decreasing trend was observed for Pt(IV) adsorption at higher HCl concentrations. In the same figures, nearly 40% adsorption is observed for Ir(III) at 0.1 M HCl though it suddenly decreases with increasing HCl concentration. About 20% adsorption was observed for Rh(III) on TMA-microalgae and TEA-microalgae, in which HCl concentration influenced adsorption insignificantly. The adsorption of base metal ions like Zn(II), Cu(II), Ni(II) and Fe(III) is insignificant under the conditions of this study. Based on the above-mentioned results, the selectivity pattern for the precious metals, as observed for TMA-microalgae and TEA-microalgae, is Pd(II)>Pt(IV), while the adsorption of Rh(III), Ir(III), and base metal ions is insignificant. In order to compare the efficiency of the adsorbents prepared in the present study with commercially available strong base anion-exchange resins, adsorption tests were carried out also by using DIAION SA11A and PA312 resins as representative resins. The characteristics of the DIAION SA11A and PA312 resins are presented in Table 2. Fig. 6(a) and (b) show the comparative plots of % adsorption of various metal ions on DIAION SA11A and PA312 resins as the function of HCl concentration, similar to Fig. 5. As shown in these figures, both strongly basic anion-exchange resins exhibit a gradual decrease in the adsorption for Pt(IV) and a sharp decrease for Pd(II) with increasing HCl concentration, contrary to the cases of TMA-microalgae and TEA-microalgae. Adsorption of Rh(III), Ir(III), Cu(II), Ni(II), Fe(III) was insignificant or negligible over the whole concentration range of HCl. However, quite considerable adsorption was observed for Zn(II) on both ion-exchange resins. From this comparative study, the different adsorption tendency of the order of selectivity among precious metal ions was observed as Pt(IV) > Pd(II) for ion-exchange resins.
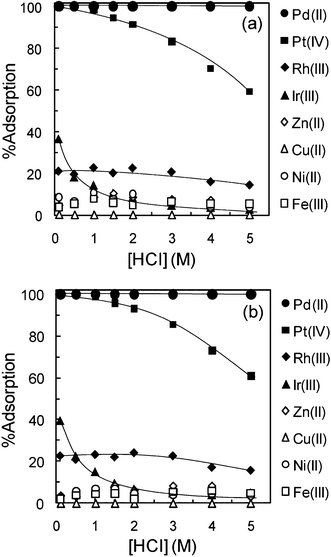 |
| Fig. 5 Effect of HCl concentration on adsorption of various metal ions on (a) trimethylamine-microalgae and (b) triethylamine-microalgae. Conditions: concentration of metal ion (mM) = Pd(0.2), Pt(0.2), Rh(0.2), Ir(0.2), Zn(1.0), Cu(1.0), Ni(1.0), and Fe(1.0), dry weight of adsorbent = 10 mg, volume of test solution = 10 mL, shaking time = 48 h, T = 30 °C. | |
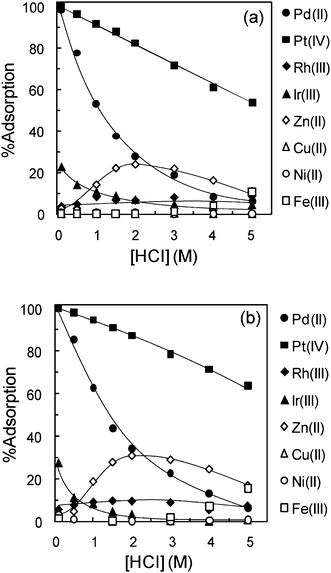 |
| Fig. 6 Effect of HCl concentration on adsorption of various metal ions on (a) DIAION SA11A and (b) DIAION PA312. Conditions: concentration of metal ion (mM) = Pd(0.2), Pt(0.2), Rh(0.2), Ir(0.2), Zn(1.0), Cu(1.0), Ni(1.0), and Fe(1.0), dry weight of adsorbent = 10 mg, volume of test solution = 10 mL, shaking time = 48 h, T = 30 °C. | |
Table 2 Characteristics of ion-exchange resins

|
Chemical characteristics |
SA11A
|
PA312
|
Structure |
Gel |
Porous |
Matrix |
Polystyrene
|
Polystyrene
|
Ionic form |
Cl
|
Cl
|
Ion-exchange capacity (meq/mL) |
>0.85 |
>1.2 |
Water content (%) |
55–65 |
49–55 |
Maximum temperature (°C) |
<80 |
<80 |
Because all tests were carried out in HCl, the predominant species of Pd(II) and Pt(IV) in such acidic chloride medium are considered as anionic chloro complexes of PdCl42− and PtCl62−, respectively.20 Meanwhile Zn(II), Cu(II), Ni(II), and Fe(III) exist mainly as cationic and neutral species. The anionic chloro complexes of these metals are significant only at high chloride concentration.21 Also, the quaternary amine functional groups of trimethylamine and triethylamine are characterized by positively charged moieties, which exhibit a strong ion-exchange capability for anionic species. Therefore, adsorption of anionic chloro complexes of metal ions on the cationic moiety is attributable to the electrostatic interaction through an anion-exchange mechanism, as expressed by the following equation:
nMicroalgae-NR3+Cl− + MClmn− = (Microalgae-NR3+)nMClmn− + nCl− |
where R is alkyl groups and M is Pd(
II) or Pt(
IV).
Adsorption isotherms for Pd(II) and Pt(IV)
In order to evaluate the adsorption capacity of the prepared adsorbents, the adsorption isotherm study for Pd(II) and Pt(IV) ions on TMA-microalgae and TEA-microalgae was conducted by varying the initial concentration of metal ions at 0.1 M HCl. The adsorption isotherms of Pd(II) and Pt(IV) on TMA-microalgae and TEA-microalgae are shown in Fig. 7(a) and (b), respectively. As evident from these figures, for both adsorbents the amount of metal adsorption (q) increases with increasing metal ion concentration in the low concentration region, and a plateau is observed at higher metal ion concentration, suggesting the typical Langmuir type adsorption. The maximum adsorption capacities of TMA-microalgae for Pd(II) and Pt(IV), as evaluated from the values in the plateau regions of Fig. 7(a), were 2.95 and 1.33 mmol g−1, respectively. Similarly, the maximum adsorption capacities of TEA-microalgae for Pd(II) and Pt(IV) were 2.57 and 1.54 mmol g−1, respectively. These values are high compared with those for other adsorbents, as shown in Table 3.
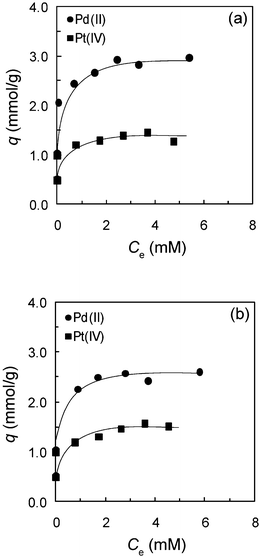 |
| Fig. 7 Adsorption isotherms of (a) trimethylamine-microalgae and (b) triethylamine-microalgae. Conditions: dry weight of adsorbent = 10 mg, volume of test solution = 10 mL, HCl concentration = 0.1 M, shaking time = 4 days, T = 30 °C. | |
Table 3 A comparative account of adsorption capacity of various adsorbents containing quaternary amine groups
Adsorbent |
Loading capacity (mmol g−1) |
Pd(II)
|
Pt(IV)
|
2-Mercaptobenzothiazole-bonded silica gel22 |
0.17 |
0.03 |
Amberite IRC 71823 |
0.55 |
0.34 |
Poly(vinyl benzyl chloride-acrylonitrile-divinylbenzene) modified with tris(2-aminoethyl)amine24 |
2.63 |
1.26 |
CHMAC-lignin16 |
0.77 |
1.77 |
GTA-lignin
16 |
1.02 |
2.79 |
TMA-microalgae |
2.95 |
1.33 |
TEA-microalgae |
2.57 |
1.54 |
Conclusions
This work demonstrated that residual biomass waste of microalgae after extraction of biofuel is promising to be used as the raw material for the preparation of adsorption materials. The prepared adsorbents; TMA-microalgae and TEA-microalgae, exhibited high affinity and remarkable capacity for the recovery of Pd(II) and Pt(IV), even though large amounts of base metal ions were coexisting in the solution. In comparison with commercially available resins, prepared adsorbents exhibited favorable efficiency. From the environmental viewpoint, prepared adsorbents are environmentally benign and prospective for future applications.
Acknowledgements
The present work was financially supported by the Ministry of Agriculture, Forestry, and Fisheries, Japan, for the research project on Development of Innovative Total Technologies for Carbon Sequestration, Biomass Production, and Biomass Utilization.
References
- A. Demirbras, Energy Policy, 2007, 35, 4661–4670 CrossRef.
- Y. Li, M. Horsman, N. Wu, C. Q. Lan and N. Dubois-Calero, Biotechnol. Prog., 2008, 24, 815–820 CAS.
- A. Satoh, M. Kato, K. Yamato, M. Ishibashi, H. Sekiguchi, N. Kurano and S. Miyachi, J. Jpn. Inst. Energy, 2010, 89, 909–913 CrossRef CAS.
- J. Li, H. Lu, J. Guo, Z. Xu and Y. Zhou, Environ. Sci. Technol., 2007, 41, 1995–2000 CrossRef CAS.
- Y. J. Park and D. J. Fray, J. Hazard. Mater., 2009, 164, 1152–1158 CrossRef CAS.
-
M. Cox, Solvent Extraction in Hydrometallurgy, ed. J. Rydberg, C. Musicas, and G. R. Shoppin, Marcel Dekker, 2004, 455–505 Search PubMed.
- J. L. Cortina, E. Meinhardt, O. Roijals and V. Marti, React. Funct. Polym., 1998, 36, 149–165 CrossRef CAS.
- J. Kramer, W. L. Driessen, K. R. Koch and J. Reedjik, Hydrometallurgy, 2002, 64, 59–68 CrossRef CAS.
-
G.W. Bedell and D.W. Darnall, Immobilization of nonviable, biosorbent, algal biomass for the recovery of metal ions, ed. B. Volesky, CRC Press, 1990, 313–326 Search PubMed.
- E. Guibal, N. V. O. Sweeney, T. Vincent and J. T. Tobin, React. Funct. Polym., 2002, 50, 149–163 CrossRef CAS.
- P. Chassary, T. Vincent, J. S. Marcano, L. E. Macaskie and E. Guibal, Hydrometallurgy, 2005, 76, 131–147 CrossRef CAS.
- M. Minamisawa, H. Minamisawa, S. Yoshida and N. Takai, Green Chem., 2005, 7, 595–601 RSC.
- K. Fujiwara, A. Ramesh, T. Maki, H. Hasegawa and K. Ueda, J. Hazard. Mater., 2007, 146, 39–50 CrossRef CAS.
- A. Ramesh, H. Hasegawa, W. Sugimoto, T. Maki and K. Ueda, Bioresour. Technol., 2008, 99, 3801–3809 CrossRef CAS.
- Y. Kanai, T. Ohshima and Y. Baba, Ind. Eng. Chem. Res., 2008, 47, 3114–3120 CrossRef CAS.
- K. Khunathai, D. Parajuli, K. Ohto, H. Kawakita, H. Harada, K. Inoue, K. Hirota and M. Funaoka, Solvent Extr. Ion Exch., 2010, 28, 403–414 CrossRef CAS.
- C. R. Adhikari, D. Parajuli, K. Inoue, K. Ohto, H. Kawakita and H. Harada, New J. Chem., 2008, 32, 1634–1641 RSC.
- K. Khunathai, Y. Xiong, B. K. Biswas, B. B. Adhikari, H. Kawakita, K. Ohto, K. Inoue, H. Kato, M. Kurata and K. Atsumi, J. Chem. Technol. Biotechnol., 2011, 86 DOI:10.1002/jctb.2733.
- S. S. Abdullah, S. Yusup, M. M. Ahmad, A. Ramli and L. Ismail, Int. J. Chem. Bio. Eng., 2010, 3, 137–141 CAS.
-
L. D. Petit and K. J. Powell, IUPAC Stability Constant Database, IUPAC and Academic Software, New York, 1999 Search PubMed.
- D. M. Borrok, R. B. Wanty, W. I. Ridly, R. Wolf, P. J. Lamothe and M. Adams, Chem. Geol., 2007, 242, 400–414 CrossRef CAS.
- Q. Pu, Z. Su, Z. Hu, X. Chang and M. Yang, J. Anal. At. Spectrom., 1998, 13, 249–253 RSC.
- C. Park, J. S. Chung and K. W. Cha, Bull. Korean Chem. Soc., 2000, 21, 121–124 CAS.
- D. Jermakowicz-Bartkowiak, B. N. Kolarz and A. Serwin, React. Funct. Polym., 2005, 65, 135–142 CrossRef CAS.
|
This journal is © The Royal Society of Chemistry 2012 |