DOI:
10.1039/C1RA00769F
(Paper)
RSC Adv., 2012,
2, 546-551
Fast reflux synthesis of multi-armed PbS dendrites, influencing factors and optical properties
Received
21st September 2011
, Accepted 27th September 2011
First published on 15th November 2011
Abstract
Multi-armed PbS dendrites have been successfully synthesized via a simple reflux route at 120 °C for 10 min, employing ethylene glycol (EG) as the solvent, (CH3COOH)2Pb and thiourea as the starting reactants, and cetyltrimethylammonium bromide (CTAB) as the surfactant. The phase and morphology of the as-prepared product was characterized by X-ray powder diffraction (XRD), scanning electron microscopy (SEM), (high-resolution) transmission electron microscopy (HR/TEM), selected area electron pattern (SAED) and energy dispersive spectroscopy (EDS). Some factors influencing the formation of multi-armed PbS dendrites were systematically investigated, including the reaction temperature and time, the original S2−ion sources, the original amount of CTAB and the sorts of surfactants, the Pb2+/S2− molar ratio, and the volume ratio of EG/water. The UV-Vis-near IR spectrum of the product showed that multi-armed PbS dendrites possessed a wide range absorption from ∼230 nm to 1100 nm. Simultaneously, a strong emission peak centered at 343 nm with two shoulder peaks centered at 413 nm and 437 nm, respectively, was presented in the emission spectrum of the product under the excitation of 288 nm light.
1. Introduction
In the past two decades, nanostructured semiconductor materials have attracted much research interest because of their fundamental significance for explaining some basic issues of the quantum confine effect and fascinating properties.1–4 Among various semiconductor nanomaterials, PbS with the direct band gap (0.41 eV at 300 K) has been paid much attention due to its relatively large exciton Bohr radius of 18 nm,5–6 high dielectric constant (18) and carrier mobility (0.44 cm2 V−1 S−1),7 as well as wide applications in many fields such as Pb2+ ion selective sensors, photography, IR detector and solar absorber.8–11 Furthermore, it has been found that PbS nanoparticles exhibited an exceptional third-order nonlinear optical property, which may be used in an optical switch.12 Also, it is predicted that the nonlinear optical properties of PbS will be the best compared with CdS and GaAs with the same particle sizes.13
With the progress of the synthesis technology in materials science, PbS micro-/nanocrystals with various morphologies and sizes have been successfully prepared, including nanoparticles,14nanorods,15nanowires,16 nanocubes17 and star-shaped, clover-like, magic-square-like and dendritic micro-/nanostructures.18–21 It is noteworthy that some common surfactants, such as sodium dodecylbenzenesulfonate (SDBS),22 polyvinyl alcohol (PVA),23cetyltrimethylammonium bromide (CTAB)24 and polyvinylpyrrolidone (PVP),25 are often employed as soft templates during controllable syntheses of PbS micro/nanostructures with certain morphology via wet chemistry routes. The hydrothermal and solvothermal syntheses are popular wet chemistry technologies since they can easily control the morphology of the final product and are environment-friendly methods. However, the longer reaction time is often needed in the process of hydrothermal/solvothermal synthesis, which is unfavorable for saving energy. Hence, it is still a challenge to search a simple and rapid route for controlled synthesis of PbS micro-/nanostructures with certain morphologies.
In this paper, we design a simple reflux route to successfully prepare PbS dendrites with multi-armed structures, employing (CH3COO)2Pb and thiourea as original reactants, ethylene glycol (EG) as the medium in the presence of CTAB. Some factors influencing the morphology of the final product are systematically investigated, including the reaction temperature and time, the original S2−ion sources, the original amount of CTAB and the sorts of surfactants, the Pb2+/S2− molar ratio, and the volume ratio of EG/water. Compared with previous literature, the present method exhibits some marked differences: First, it is cost-effective since PbS dendrites with multi-armed structures can be obtained at the lower temperature range from 80 °C to 160 °C at a shorter time (1–10 min); second, the morphology of PbS can be easily tuned by varying experimental parameters. More interesting, multi-armed PbS dendrites obtained by the present method present a strong and wide UV-Vis-near IR absorption in the ranges from ∼230 nm to 1100 nm. This probably provides new applications of PbS materials in optical devices.
2. Experimental section
All reagents were analytically pure, purchased from Shanghai Chemical Company and used without further purification.
2.1 Preparation of multi-armed PbS dendrites
In a typical experimental procedure, the appropriate amounts of (CH3COOH)2Pb (2 mmol, 0.7585 g) and thiourea (2 mmol, 0.1520 g) were dissolved in 10 mL EG to form solution A and B, respectively. Then, 0.05 g of CTAB was added into a three-neck flask with 10 mL EG under magnetic stirring. After heating the flask to 120 °C, 2 mL EG solution of (CH3COOH)2Pb and 2 mL EG solution of thiourea were simultaneously injected into the above three-neck flask with two syringes. The system was kept 120 °C for 10 min. After that, the system was cooled to room temperature naturally. The precipitates were collected, washed with distilled water and absolute ethanol several times to remove soluble ions, and dried in vacuum at 60 °C for 6 h.
2.2 Characterization
X-ray powder diffraction pattern of the product was carried out on a Shimadzu XRD-6000 X-ray diffractometer equipped with Cu-Kα radiation (λ = 0.154060 nm), employing a scanning rate of 0.02°s−1 and 2θ ranges from 20° to 70°. SAED pattern and TEM image of the product were carried out on a JEOL-2010 high resolution transmission electron microscope, employing an accelerating voltage of 200 kV. SEM images and EDS of the product were obtained on Hitachi S-4800 field emission scanning electron microscope, employing the accelerating voltage of 5 kV and 15 kV, respectively. The UV-Vis absorption spectrum of the product was recorded on a Hitachi U-4100 spectrophotometer (Tokyo, Japan), employing the distilled water as the reference. The PL spectra of the product were measured with an F-4500 spectrofluorometer (Hitachi) with a quartz cell of 1 cm.
3. Results and discussion
3.1 Structure and morphology of the products
The phase of the product is determined by XRD analysis. Fig. 1a shows the XRD pattern of the product prepared from the EG solution via the simple reflux method at 120 °C for 10 min. By comparison with the data of the JCPDS standard card files no.65-0692, all peaks could be indexed as the cubic PbS form, indicating that the product is pure. The formation of PbS is confirmed by EDS analysis. As shown in Fig. 1b, Cu, Pb and S peaks can be easily found. The Cu peaks are ascribed to the Cu substrate.
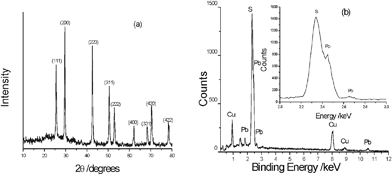 |
| Fig. 1 (a) The XRD pattern and (b) EDS analysis of the product prepared by the reflux technology at 120 °C for 10 min. | |
Fig. 2a shows a representative low-magnification SEM image of the product obtained under the current conditions. Abundant dendrites with multi-armed structures are clearly exhibited. Further enlargement shows that each arm is consisted of plentiful nanorods, which are crossed over each other and present X-shaped spatial-structures (see Fig. 2b). A typical TEM image is given in Fig. 2c. Multi-armed microstructures can be easily seen and hierarchically dendritic structures are exhibited in every arm. The subbranches are parallel to each other and meet at right angles with the trunk in each arm. The electron diffractions of the subbranches prove the good crystallinity of the product (see the inset in Fig. 2c). Fig. 2d depicts a typical HRTEM image of the subbranch. The clear stripes further confirm the single-crystalline nature of the subbranches and the distance of the neighbouring planes is measured to be 0.295 nm, which is very close to 0.297 nm of (200) plane of the cubic PbS form.
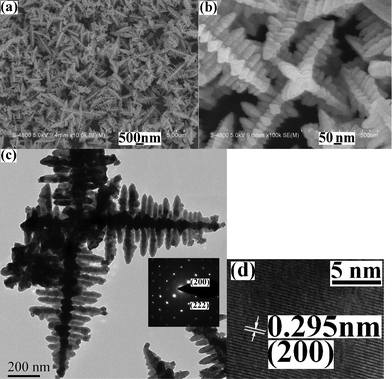 |
| Fig. 2
Electron micrographs of the product by the reflux technology at 120 °C for 10 min: (a) a low magnification SEM image, (b) a high magnification SEM image, (c) a typical TEM image (the inset: SAED pattern) and (d) a HRTEM image. | |
3.2 The influences of the reaction temperature and time
Usually, the temperature is one of the most important factors in a chemical reaction. The higher the temperature is, the faster the chemical reaction rate is. In the present work, when the reaction was carried out at 80 °C for 10 min, SEM observations showed that the product was still multi-armed structures (see Fig. 3a). However, the yield of the product was low, the size was small and the dendritic structures were undeveloped (see the inset in Fig. 3a). After raising the reaction temperature to 100 °C or 120 °C, the yield and size of the product markedly increased and the multi-armed dendrites gradually completed (see Fig. 3b and Fig. 2a). However, upon further enhancement of the temperature, for example to 140 °C and 160 °C, the yield, size and morphology of the product hardly changed (see Fig. 3c and Fig. 3d). The above experiments clearly show that lifting the reaction temperature within the proper scope avails to promote the growth and improvement of the dendrites with multi-armed structures.
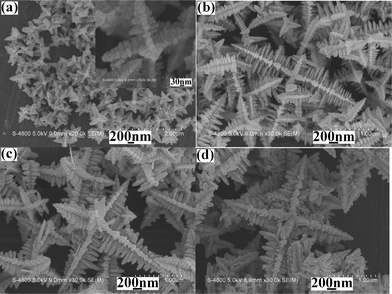 |
| Fig. 3
SEM images of the products prepared from the same systems at different reaction temperatures for 10 min: (a) 80 °C, (b) 100 °C, (c) 140 °C and (d) 160 °C. | |
The time is another important factor influencing a chemical reaction. In the current work, to investigate the influence of the reaction time on the formation of PbS dendrites with multi-armed structures, we prepared the products from the same system at 120 °C for 1, 3, 5 and 7 min. Although the yield of the product markedly increased with the prolonging of the reaction time from 1 min to 7 min, the morphology of PbS dendrites hardly changed (see Fig. 4a; owing to the similarity of shapes, only the product prepared for 1 min was exhibited here). After 10 min, the yield seldom increased, implying that the reaction to produce PbS completed. Moreover, no obvious change was found in the size of PbS dendrites after reacting for 3 min, even for 160 min (see Fig. 4b). The above factors indicate that the formation of PbS dendrites with multi-armed structures is unrelated to the reaction time.
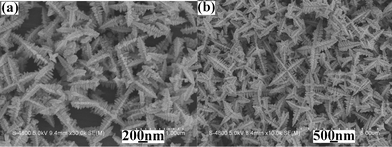 |
| Fig. 4
SEM images of the products prepared from the same systems at 120 °C for different durations: (a) 1 min and (b) 160 min. | |
3.3 The influences of S2− sources and the molar ratios of original Pb2+/S2− sources
Experiments showed that no PbS dendrites with multi-armed structures were synthesized when thiourea was replaced by other S2−ion sources. As shown in Fig. 5, the products are respectively comprised of aggregated nanoparticles with some nanosheets, and near-spherical nanoparticles with the size of 50–100 nm when Na2S and CH3CSNH2 are used as the original sulfur sources. Distinctly, thiourea is indispensable in the formation of PbS dendrites with multi-armed structures. The above results should be ascribed to the nature of the S2−ion sources. When Na2S was used as the sulfur source, Pb2+ and S2− rapidly reacted to produce PbS. This led to the formation of a great deal of PbS nuclei. Finally, we could only obtain PbS nanoparticles. CH3CSNH2 is a compound easily decomposed to free H2S at the lower temperature. Under the present conditions, it acted in a role similar to Na2S. Thus, PbS dendrites cannot be formed. While thiourea is a stable matter, it can be decomposed at the higher temperature. Thus, the smaller PbS nuclei were produced, which led to the final formation of PbS dendrites. Furthermore, we investigated the influence of the amount of thiourea on the formation of PbS dendrites with multi-armed structures. A series of products was synthesized via changing the molar ratio of original Pb2+/S2− sources under the same experimental conditions (see Fig. 6). As shown in Fig. 6a, the product is a mixture consisting of abundant dendrites with multi-armed structures and some spherical nanoparticles with ∼200 nm in diameter when the original Pb2+/S2− molar ratio of 5
:
1 is employed. With the decrease of the molar ratio of original Pb2+/S2− sources, for example to 4
:
1 and 2
:
1, the amounts of spherical nanoparticles gradually reduce (Fig. 6b). Simultaneously, the sizes of dendrites with multi-armed structures also reduce. Upon further decreasing the molar ratio of original Pb2+/S2− sources from 1
:
1 to 1
:
4, the morphology and size of the final product hardly change (see Fig. 2a and Fig. 6c). The above facts imply that the amount of thiourea slightly affects the formation of multi-armed dendrites.
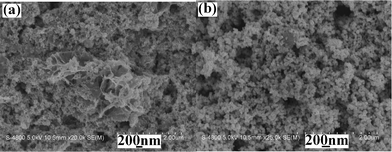 |
| Fig. 5
SEM images of the products prepared from the systems containing various S2−ion sources: (a) Na2S and (b) CH3CSNH2. | |
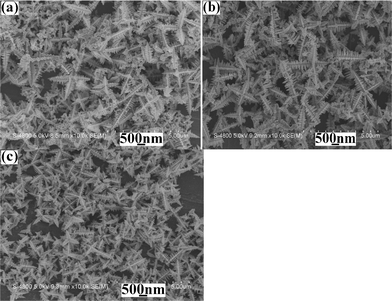 |
| Fig. 6
SEM images of as-prepared products from the systems with various original Pb2+/S2− ratios at 120 °C for 10 min: (a) 5 : 1, (b) 4 : 1 and (c) 1 : 4. | |
3.4 The influences of the amount of original CTAB and surfactant sorts
It was found that CTAB played an important role in the formation of PbS dendrites with multi-armed structures. When no surfactant was employed, the product was composed of flowerlike microstructures (see Fig. 7a). After 0.025 g of CTAB was used, abundant PbS dendrites with multi-armed structures appeared (Fig. 7b). Upon further increasing the amount of CTAB to 0.05 g and 0.1 g, PbS dendrites hardly varied (see Fig. 2a and Fig. 7c). However, after more CTAB was employed, dendritic structures decreased and microflakes increased. The above factors exhibit the proper amounts of CTAB act as the structure-directing reagent in the formation of PbS dendrites with multi-armed structures. After more CTAB was added, some active sites of PbS nuclei were occupied by CTAB, which slowed down or prevented the growth of PbS crystals along these directions. Thus, some flake-like microstructures appeared. Moreover, when CTAB was replaced by other common surfactants with the same amount, such as sodium dodecylbenzenesulfonate (SDBS), sodium dodecyl sulfate (SDS) and polyvinylpyrrolidone (PVP), SEM observations showed that the morphologies of the products obtained under the same experimental conditions markedly varied (see Fig. 8). Although dendritic PbS microstructures could also be obtained under the assistance of PVP, every arm was made of microrods with four edges (see Fig. 8c), which obviously differed from the morphology of PbS prepared in the presence of CTAB. The above experiments further confirm that CTAB is indispensable in the formation of PbS dendrites with multi-armed structures. In the present work, Pb(CH3COO)2 was used as the Pb2+ ion source. It is well known that CH3COO− ions bear strong affinity to Pb2+ ions. It was probable that CH3COO− ions were adsorbed by the surrounding PbS nuclei after PbS had been produced, which led to the negative charge on the surfaces of PbS nuclei. CTAB is a cationic surfactant, so it can markedly affect the morphology of the final product due to the static interaction. Reversely, SDBS and SDS are anionic surfactants. They are away from PbS nuclei owing to the repulsive action of connatural charges. Thus, no PbS dendrites were obtained. As a non-ionic surfactant, PVP can appear surrounding the PbS nuclei, which resulted in the formation of PbS dendrites. However, the different combining fashions of PVP and CTAB to PbS nuclei caused the morphology change of the final product.
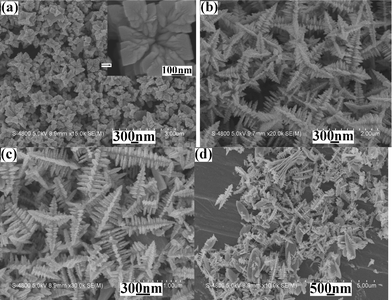 |
| Fig. 7
SEM images of the products prepared from the systems containing various amounts of CTAB at 120 °C for 10 min: (a) 0.0 g, (b) 0.025 g, (c) 0.10 g and (d) 0.40 g. | |
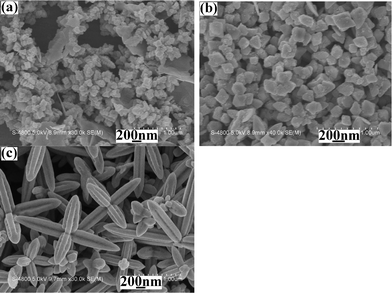 |
| Fig. 8
SEM images of the products prepared from the systems containing different surfactants at 120 °C for 10 min: (a) SDBS, (b) SDS and (c) PVP. | |
3.5 The influence of the solvent
It was noteworthy that EG was also a crucial factor influencing the formation of PbS dendrites with multi-armed structures. Experiments showed that the morphology of the final product could be remarkably changed when the mixture of EG and water with a certain volume ratio was selected as the solvent. Fig. 9 depicts SEM images of the products prepared at 120 °C for 10 min from the systems containing the EG/water volumes of 1
:
9, 5
:
5, 6
:
4 and 9
:
1, respectively. Obviously, when the EG/water volume ratio was below 5
:
5, the products were mainly comprised of microrods (see Fig. 9a). With the increase of the EG/water volume ratio, the size of the microrods decreased. After the EG/water volume ratio of 5
:
5 was used as the solvent, microrods and dendrites with multi-armed structures coexisted in the product (Fig. 9b). Upon further increase in the EG/water volume ratio, microrods rapidly reduced (see Fig. 9c), even completely disappearing (Fig. 9d). The above phenomena further confirm that EG is indispensable during the formation of PbS dendrites with multi-armed structures, too. At the same time, the yield of PbS gradually increased with the enhancement of the EG/water volume ratio. This is understandable. In the present reflux system, it was difficult that H2S produced by thiourea at lifted temperature escaped from the system due to the bigger viscidity of EG. Hence, PbS with the high yield was obtained. However, with the introduction of water, the viscidity of the system rapidly decreased. Thus, the escape rate of H2S out of the system quickened. As a result of the reduction of the H2S amount, the yield of the final product decreased.
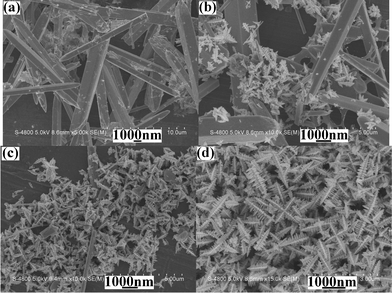 |
| Fig. 9
SEM images of the products prepared under the same experimental conditions from the systems with various EG/water volume ratios: (a) 1 : 9, (b) 5 : 5, (c) 6 : 4 and (d) 9 : 1. | |
3.6 Possible formation mechanism of PbS dendrites with multi-armed structures
Based on the above investigations, one can find that thiourea, CTAB and EG are indispensable in the formation of PbS dendrites with multi-armed structures under the current experimental conditions. In the present work, thiourea could release H2S at greater temperatures due to the reaction with EG, which should be similar to the hydrolysis of thiourea.19 The produced H2S could rapidly react with Pb2+ ions to produce PbS. Generally, the cubic PbS phase easily forms cubes. However, when {111} planes have a faster growth rate than other planes, flowerlike structures can be prepared.18 As shown in Fig. 8a, when no surfactant was employed, some immature flowerlike structures could be prepared, indicating that {111} planes have the faster growth rate. After a certain amount of CTAB was introduced, the growth rates of some planes were restrained. As a result, PbS dendrites with multi-armed structures were formed.
3.7 Optical properties of PbS dendrites with multi-armed structures
It is found that the as-prepared multi-armed PbS dendrites exhibit good optical properties. Fig. 10a gives the UV-Vis-near IR absorption spectrum of the product. A wide absorption peak ranged from ∼230 nm to 1100 nm can be clearly seen and the biggest absorption position is located at ∼800 nm, indicating that multi-armed PbS dendrites obtained under the present experimental conditions can absorb the complete light spectrum from near-UV to near IR. Compared with Liu's report,17 our product possesses a wider absorption range. This will provide new applications of multi-armed PbS dendrites in optical devices. Furthermore, a weak absorption peak is found at ∼259 nm, which is in good agreement with our previous report.19 The PL spectra of the product are depicted in Fig. 10b. A strong exciting peak centered at 288 nm and a weak exciting one at 233 nm can be seen in the exciting spectrum of the product. When the light of 288 nm was employed as the exciting wavelength, a strong emission peak centered at 343 nm with two shoulder peaks centered at 413 nm and 437 nm, respectively, was presented in the emission spectrum of the product. While 233 nm light was selected as the exciting wavelength, the emission peak situation did not change, but the peak intensity markedly decreased. The above PL spectra are distinctly different from those reported in ref. 19, confirming that the performances of nanomaterials can be tuned by their morphologies.
4. Conclusions
In summary, multi-armed PbS dendrites were successfully synthesized via the simple EG reflux method at 120 °C for 10 min in the presence of proper amounts of CTAB, employing (CH3COOH)2Pb and thiourea as starting materials. The systematic investigations showed that CTAB, thiourea and EG were indispensable in the formation of PbS dendrites with multi-armed structures. Moreover, the sorts of surfactants and the volume ratio of EG/water could markedly affect the morphology of the final product. The as-obtained PbS dendrites with multi-armed structures exhibited novel optical properties, which probably provided new applications of PbS materials in optical devices.
Acknowledgements
The authors thank the National Natural Science Foundation of China (21171005) and the Key Foundation of Chinese Ministry of Education (210098) for the fund support.
References
- L. H. Dong, Y. Chu, Y. Liu, M. Y. Li, F. Y. Yang and L. L. Li, J. Colloid Interface Sci., 2006, 301, 503 CrossRef CAS.
- X. F. Shen and X. P. Yan, J. Mater. Chem., 2008, 18, 4631 RSC.
- J. H. Xiang, H. Q. Cao, Q. Z. Wu, S. C. Zhang and X. R. Zhang, Cryst. Growth Des., 2008, 8, 3935 CAS.
- C. Zhang, Z. H. Kang, E. H. Shen, E. B. Wang, L. Gao, F. Luo, C. G. Tian, C. L. Wang, Y. Lan, J. X. Li and X. J. Cao, J. Phys. Chem. B, 2006, 110, 184 CrossRef CAS.
- C. W. Wang, H. G. Liu, X. T. Bai, Q. B. Xue, X. Chen, Y. Lee, J. C. Hao and J. Z. Jiang, Cryst. Growth Des., 2008, 8, 2660 CAS.
- G. Q. Xin, H. P. Ding, Y. G. Yang, S. L. Shen, Z. C. Xiong, X. Chen, J. C. Hao and H. G. Liu, Cryst. Growth Des., 2009, 9, 2008 CAS.
- J. Akhtar, M. A. Malik, P. O'Brien, K. G. U. Wijayantha, R. Dharmadasa, S. J. O. Hardman, D. M. Graham, B. F. Spencer, S. K. Stubbs, W. R. Flavell, D. J. Binks, F. Sirotti, M. El. Kazzi and M. Silly, J. Mater. Chem., 2010, 20, 2336 RSC.
- H. Hirata and K. Higashiyama, Bull. Chem. Soc. Jpn., 1971, 44, 2420 CrossRef CAS.
- P. K. Nair, O. Gomezdaza and M. T. S. Nair, Adv. Mater. Opt. Electron., 1992, 1, 139 CrossRef CAS.
- P. Gadenne, Y. Yagil and G. Deutscher, J. Appl. Phys., 1989, 66, 3019 CrossRef CAS.
- T. K. Chaudhuri and S. Chatterjes, Proc. Int. Conf. Thermoelectron., 1992, 11, 40 Search PubMed.
- R. S. Kane, R. E. Cohen and R. Silbey, J. Phys. Chem., 1996, 100, 7928 CrossRef CAS.
- M. A. Olshavasky, A. N. Goldstein and A. P. Alivisatos, J. Am. Chem. Soc., 1990, 112, 9438 CrossRef.
- Z. P. Qiao, Y. Xie, J. G.Xu, Y. J. Zhu and Y. T. Qian, J. Colloid Interface Sci., 1999, 214, 459 CrossRef CAS.
- Y. Y. Feng, J. Zhang, P. Zhou, G. F. Lu, J. C. Bao, W. Wang and Z. Xu, Mater. Res. Bull., 2004, 39, 1999 CrossRef CAS.
- D. B. Yu, D. B. Wang, Z. Y. Meng, J. Lu and Y. T. Qian, J. Mater. Chem., 2002, 12, 403 RSC.
- Q. Liu, Y. H. Ni, G. Yin, J. M. Hong and Z. Xu, Mater. Chem. Phys., 2005, 89, 379 CrossRef CAS.
-
(a) Y. H. Ni, H. J. Liu, F. Wang, Y. Y. Liang, J. M. Hong, X. Ma and Z. Xu, Cryst. Growth Des., 2004, 4, 759 CrossRef CAS;
(b) Y. H. Ni, F. Wang, H. J. Liu, Y. Gui, J. M. Hong, X. Ma and Z. Xu, J. Cryst. Growth, 2004, 262, 399 CrossRef CAS.
- Y. H. Ni, H. J. Liu, F. Wang, Y. Y. Liang, J. M. Hong, X. Ma and Z. Xu, Cryst. Res. Technol., 2004, 39, 198 CrossRef.
- Y. H. Ni, X. W. Wei, J. M. Hong and X. Ma, Mater. Res. Bull., 2007, 42, 17 CrossRef CAS.
- D. B. Wang, D. B. Yu, M. W. Shao, X. M. Liu, W. C. Yu and Y. T. Qian, J. Cryst. Growth, 2003, 257, 384 CrossRef CAS.
- Z. P. Qiao, Y. Zhang, L. T. Zhou and Q. Xire, Cryst. Growth Des., 2007, 7, 2394 CAS.
- M. S. Ghamsari, S. Radiman, M. A. Yarmo, M. A. Abdul-Hamid, A. Bananej and I. A. Rahman, Mater. Lett., 2011, 65, 1381 CrossRef CAS.
- N. Wang, X. Cao, L. Guo, S. H. Yang and Z. Y. Wu, ACS Nano, 2008, 2, 184 CrossRef CAS.
- Z. P. Peng, Y. S. Jiang, Y. H. Song, C. Wang and H. J. Zhang, Chem. Mater., 2008, 20, 3153 CrossRef CAS.
|
This journal is © The Royal Society of Chemistry 2012 |