DOI:
10.1039/C1NJ20532C
(Paper)
New J. Chem., 2012,
36, 168-172
A tunable warm-white-light Sr3Gd(PO4)3:Eu2+,Mn2+ phosphor system for LED-based solid-state lighting†
Received
(in Victoria, Australia)
17th June 2011
, Accepted 3rd November 2011
First published on 17th November 2011
Abstract
A novel tunable full-color-emitting Sr3Gd(PO4)3:Eu2+,Mn2+ phosphor system was successfully developed. Its excitation wavelength ranging from 230 to 450 nm fits well with the characteristic emission of UV light-emitting diode (LED) chips. The color emission of these phosphors can be tuned across almost the entire visible light spectrum resulting in white light emission with varied hue and correlated color temperature (CCT) by varying the molar ratios of Eu2+ and Mn2+. Moreover, the efficient energy transfer from Eu2+ to Mn2+ was demonstrated via a dipole–quadrupole mechanism by the luminescence spectra and the fluorescence decay dynamics based on the Inokuti–Hirayama theoretical model. These results indicate that the developed phosphor may be promising as a single-component white-light-emitting phosphor for white LEDs.
1. Introduction
In recent years, solid-state lighting based upon a combination of UV/blue LED chips and down-conversion phosphors has attracted enormous commercial interest. Compared with the incandescent or fluorescent lamps, LED-based solid-state lighting can provide significant power saving, higher luminous efficiency, longer lifetime and environmentally friendly characteristics.1–3 The most dominant way to create a white LED is by combining a blue InGaN chip with a yellow-emitting Y3Al5O12:Ce3+ phosphor. However, its color rendering index is limited due to color deficiency in the red spectral region.4–6 Current lighting technology employs UV LED chips with red, green, and blue phosphors to improve this problem. Recently, single-component full-color emitting phosphors have attracted more attention for near-UV chip based white LEDs because they can facilitate high color rendering index and prevent the loss of luminous efficiency which often exists in phosphor blend systems owing to the strong reabsorption of the blue light by the red and green phosphors. Compared to white LEDs with multi-component emitters, white LEDs based on a single-component emitter have definite advantages, such as improved stability, better reproducibility, and simplified fabrication process.7 Some new single-component white-light-emitting phosphors such as Sr3Al2O5Cl2:Ce3+,Eu2+,8CaAl2Si2O8:Eu2+,Mn2+,9 and Ca9Gd(PO4)7:Eu2+,Mn2+10 have been reported in the literature. However, until now, few single-component materials have been reported to emit satisfactory white-light emission with both good color rendering index and luminous efficiency. Therefore, the search for new single-component white-light phosphors with perfect stability and color reproducibility for use in white LEDs is of obvious interest and importance.
Herein, we firstly report on the synthesis and photoluminescence properties of a novel material, Sr3Gd(PO4)3:Eu2+,Mn2+, which can act as an excellent single-component warm-white-light phosphor for use in UV-based white LEDs. The emission covers the full spectral range of the visible region leading to a warm white emission. We have also investigated its luminescent properties as well as the energy transfer between the Eu2+ and Mn2+ ions. Moreover, the fluorescence decay curves have been fitted and analyzed using the Inokuti–Hirayama theoretical model in order to estimate the Eu–Mn interaction mechanism in the host lattice.
2. Experimental section
2.1. Materials and synthesis
Powder samples Sr3(1−m−n)Gd(PO4)3:mEu2+,nMn2+ (SGP:mEu2+,nMn2+) were synthesized by a conventional solid state reaction method. The stoichiometric amounts of raw materials SrCO3 (A.R. (Analytical Reagent)), Gd2O3 (99.99%), NH4H2PO4 (A.R.), Eu2O3 (99.99%) and MnCO3 (A.R.) were first well grounded in an agate mortar. They were then put into crucibles and subsequently heated at 1350 °C for 2 h in an activated carbon reducing atmosphere. After regrinding, they were sintered at 1200 °C under a 10% H2–90%N2 gas mixture for 2 h. Finally the as-synthesized samples were slowly cooled to room temperature inside the tube furnace under H2–N2 flow.
2.2. Measurements and characterization
The phase purity of SGP:mEu2+,nMn2+ phosphors was carefully checked by using powder X-ray diffraction (XRD) analysis (Bruker AXS D8) in the 2θ range from 10° to 80°, with graphite monochromatized Cu Kα radiation (λ = 0.15405 nm) operating at 40 kV and 40 mA. A step size of 0.02° (2θ) was used with a scanning speed of 9° per min in the 2θ range. The photoluminescence emission (PL) and photoluminescence excitation (PLE) spectra of the obtained powders were recorded with a Hitachi F-4500 spectrophotometer equipped with a 150 W xenon lamp as the excitation source. The luminescence decay curve was obtained from a Lecroy Wave Runner 6100 digital oscilloscope (1 GHz) using a tunable laser (pulse width = 4 ns, gate = 50 ns) as the excitation source (Continuum Sunlite OPO). The quantum efficiency (QE) was analyzed with a PL quantum-efficiency measurement system (C9920-02, Hamamatsu Photonics, Shizuoka) by a 150 W xenon lamp. All the measurements were performed at room temperature.
3. Results and discussion
3.1.
Phase identification of SGP:0.01Eu2+,nMn2+ phosphors
The Sr3Gd(PO4)3 structure is described in the cubic I43d space group with lattice constant a = 10.114 Å, and Sr and Gd occupy the same site with a C3 symmetry.11,12Fig. 1a shows the XRD pattern of the SGP:0.01Eu2+,nMn2+ powder samples with increasing Mn2+ concentration from n = 0 to 0.08. The results show that all peaks can be indexed based on a eulytite-type structure of Sr3Gd(PO4)3 and match well with JCPDS file No. 29-1301, indicating that the obtained samples are single phase and Eu2+/Mn2+ ions can be easily introduced to substitute the Sr2+ lattice site. The diffraction peaks shift to higher angles with increasing Mn2+ concentration, which may be ascribed to the substitution of the larger Sr2+ by the smaller Mn2+. In addition, the lattice constants of SGP:0.01Eu2+,nMn2+ samples were calculated by refining the powder XRD data according to the Rietveld method using General Structure Analysis System (GSAS) programming. From Fig. 1b, we can see that the lattice constant a for SGP:0.01Eu2+,nMn2+ samples decreases linearly with increasing Mn2+ concentration, which is consistent with Vegard's law.13 The XRD analysis and change in lattice constant a can confirm that the Eu2+ and Mn2+ ions have been effectively built into the Sr3Gd(PO4)3 host lattice.
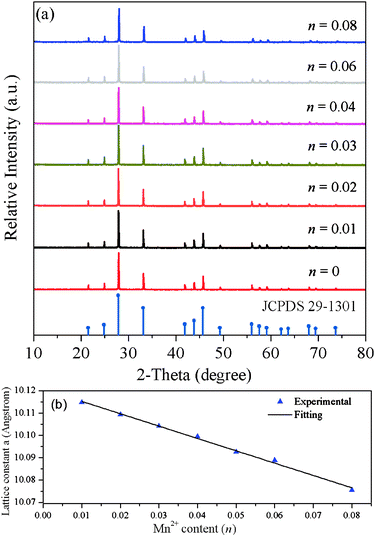 |
| Fig. 1 (a) XRD patterns of SGP:0.01Eu2+,nMn2+ samples. The standard data for Sr3Gd(PO4)3 (JCPDS card no. 29-1301) are shown as a reference. (b) The relationship between the lattice constant a and Mn2+ content (n). | |
3.2. Photoluminescence properties
Fig. 2a depicts the PLE and PL spectra of SGP:0.01Eu2+ phosphor. The PLE spectrum monitored at 500 nm exhibits a broad band from 230 to 450 nm, which can be assigned to the electron transition from the 4f energy level to different 5d sub levels of Eu2+ ions. Upon 355 nm excitation, the PL spectrum consists of several broad bands which are ascribed to the 4f65d1–4f7 transition of the Eu2+ ions. As shown in Fig. 2b, this broad emission band can be decomposed into three well separated Gaussian components on an energy scale with maxima at 2.806 eV, 2.538 eV, and 2.315 eV corresponding to 442 nm, 489 nm, and 536 nm, respectively. Therefore, these suggest that there are three luminescent Eu2+ centers in the SGP host lattice with different local coordination environments, which is also supported by the crystal structure of the host lattice. The Sr3Gd(PO4)3 host contains three possible different orientations of the [PO4] tetrahedron within the (Sr,Gd)8 bisdisphenoid corresponding to the three sets of partially occupied oxygen positions. The Gd3+/Sr2+ pairs of cations are disordered on a single crystallographic site (C3 point group symmetry) while the oxygen atoms of the phosphate groups are distributed over three partially occupied sites.11,12 That is to say, Sr2+ ions occupy three different C3 crystallographic sites and have different crystal field strengths. Because the doping Eu2+ ions substitute the sites of the Sr2+, the Eu2+ ions occupy three different sites, which results in three well separated Gaussian bands.
The PLE and PL spectra of SGP:0.06Mn2+ sample are shown in Fig. S1 (ESI†). The PLE spectrum consists of several peaks centered at 363, 407, 508 and 543 nm, corresponding to Mn2+ transitions from ground level 6A1 (6S) to 4T2 (4D), [4A1 (4G), 4E (4G)], 4T2 (4G) and 4T1 (4G) levels. The PL spectrum shows a broad red emission band centered at 630 nm ascribed to the spin-forbidden 4T1(4G) → 6A1(6S) transition of the Mn2+ ions. Mn2+-doped luminescent materials have been known to show wide-ranging emission from 500 to 700 nm which is strongly affected by the strength of the crystal field and coordination number. Tetrahedrally coordinated Mn2+ in the host usually gives a green to yellow emission, whereas it emits red light in octahedral coordination.14–16,20 In our case, the phosphors emit a broad red emission band peaked at 630 nm indicating that the Mn2+ ion occupies an octahedral site.
As seen in Fig. 3, we have observed a significant spectral overlap between the Eu2+ PL and Mn2+ PLE spectra, indicating that the emission spectrum of the Eu2+ ions matches with the excitation spectrum of the Mn2+ ions. Therefore, an effective resonance-type energy transfer from the Eu2+ to Mn2+ ions was expected. Moreover, as shown in Fig. 4, another evidence for energy transfer in SGP:0.01Eu2+,nMn2+ samples is that the PLE spectra monitoring the emission of the Mn2+ and the emission of Eu2+ are similar. The PLE spectrum shows a broad band ranging from 230 to 450 nm which matches well with the emission of commercially UV LED chips. After codoping Eu2+ and Mn2+ ions in the Sr3Gd(PO4)3 host, the sample exhibits a tunable color emission under UV excitation. In Fig. 4, the PL spectrum of the SGP:0.01Eu2+,0.02Mn2+ sample shows a multicolor emission under excitation at 355 nm, which consists of a blue-green band corresponding to the f–d transition of the Eu2+ ions and a red band attributed to the 4T1–6A1 transition of the Mn2+ ions, respectively. Thus, we can adjust the relative intensity of the emission bands by tuning the amounts of the activator and pure white light can be obtained in a single host.
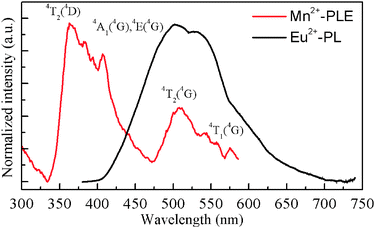 |
| Fig. 3 Spectral overlap between the normalized PL spectrum of SGP:Eu2+ and the PLE spectrum of SGP:Mn2+. | |
In order to understand the energy transfer process, a series of samples were prepared. The Eu2+ content was fixed at 0.01 and the doping content of Mn2+ was varied from 0.005 to 0.08. Fig. 5 depicts the PL of SGP:0.01Eu2+,nMn2+ samples with varying Mn2+ content under excitation at 355 nm. With increasing Mn2+ content, it is found that the relative emission intensity of the Eu2+ ions decreases remarkably from n = 0.005 to 0.08 assigned to the enhancement of energy transfer from the Eu2+ ions to Mn2+ ions. Meanwhile, the emission intensity of the Mn2+ ions increases initially and reaches a maximum at n = 0.05, beyond which it decreases ascribed to the Mn2+–Mn2+ internal concentration quenching. Furthermore, the emission peak of the Mn2+ ions shows a red-shift from 600 nm to 630 nm as the content increases from 0.005 to 0.08. Since a smaller Mn2+ ion substitution on Sr2+ sites causes a shrinking of bond lengths, as confirmed by the XRD pattern shift to higher angles and the lattice constant a for SGP:0.01Eu2+,nMn2+ samples decreases linearly with increasing Mn2+ concentration (Fig. 1), this leads to an increase of the crystal field strength which is inversely proportional to the bond length, and consequently a red-shift of the emission peak.14 In addition, the Mn2+ ions occupying different coordination environments may also contribute to this red shift.15,16
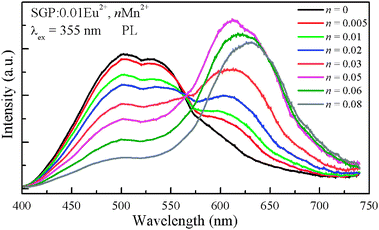 |
| Fig. 5
PL spectra for SGP:0.01Eu2+,nMn2+ phosphors on Mn2+ doping content (n). | |
The PL decay curves of the Eu2+ emission in SGP:0.01Eu2+,nMn2+ samples excited at 355 nm and monitored at 500 nm are shown in Fig. 6. We can see that the decay curves of the Eu2+ emission deviate slightly from a single exponential rule at lower Mn2+ content and the deviations become more evident with an increase of the Mn2+ concentration. The effective lifetime of the decay curves for Eu2+ emission was evaluated using the equation
|  | (1) |
The effective lifetime values were determined to be 0.688, 0.646, 0.602, 0.533, 0.471, 0.419, 0.365, 0.332, and 0.310 μs for SGP:0.01Eu
2+,
nMn
2+ samples with
n = 0, 0.005, 0.01, 0.02, 0.03, 0.04, 0.05, 0.06, and 0.08, respectively. The decay time decreases monotonically as the Mn
2+ concentration increases, which strongly demonstrated the energy transfer from Eu
2+ to Mn
2+.
2,17 The energy transfer efficiency
ηT from the Eu
2+ to Mn
2+ ions can be expressed by
18where
τ and
τ0 is the lifetime of the Eu
2+ ions with and without the Mn
2+ ions. The energy transfer efficiency was calculated as a function of Mn
2+ content and is shown in
Fig. 7. The energy transfer efficiency is observed to increase gradually with increasing Mn
2+ doping concentration. The maximum value of
ηT is estimated to be 54.9%. The results confirm that the energy transfer process from the Eu
2+ to Mn
2+ ions is efficient.
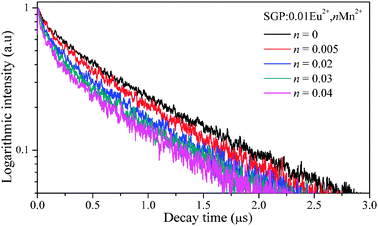 |
| Fig. 6 Photoluminescence decay curves of Eu2+ in SGP:0.01Eu2+,nMn2+ (excited at 355 nm, monitored at 500 nm). | |
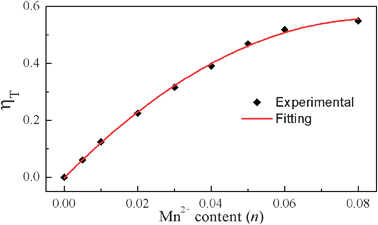 |
| Fig. 7 Dependence of the energy transfer efficiency ηT on Mn2+ content (n). | |
Generally, the energy transfer from Eu2+ to Mn2+ ions occurs through multipolar interactions. When the donor and acceptor ions are uniformly distributed in the host and the migration process is negligible compared to the energy transfer between donors and acceptors, then the donor's decay curves following the Inokuti–Hirayama (I–H) model equation for multipolar interactions;19
| 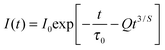 | (3) |
where
τ0 is the intrinsic decay time of the donors in the absence of acceptors. The value
S = 6, 8, and 10 corresponds to dipole–dipole, dipole–quadrupole, and quadrupole–quadrupole interactions, respectively. The energy transfer parameter
Q is defined as
| 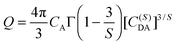 | (4) |
where
CA is the concentration of acceptors,
Γ(
x) is the gamma function, and
C(S)DA is the microscopic energy transfer parameter that characterizes the process. In order to characterize the energy transfer process, the luminescence decay curves have been fitted using the I–H theoretical model. The decay curves of the SGP:0.01Eu
2+,0.005Mn
2+ sample and theoretical fit are illustrated in
Fig. 8. The best agreement between PL decay curves of the Eu
2+ emission and
eqn (4) was achieved for
S = 8, indicating that the dominant interaction mechanism for the energy transfer is based on the dipole–quadrupole interaction.
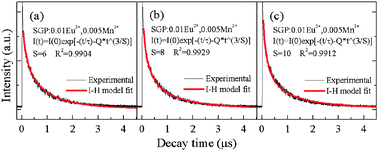 |
| Fig. 8 Photoluminescence decay curves of Eu2+ in SGP:0.01Eu2+,0.005Mn2+ (excited at 355 nm, monitored at 500 nm). The solid lines correspond to the fits to the Inokuti–Hirayama model (a) S = 6, (b) S = 8, (c) S = 10. | |
Considering the dipole–quadrupole interaction mechanism, the critical distance RC from a sensitizer to an accepter is given by the spectral overlap method. Hence, RC can be obtained from the following formula20
|  | (5) |
where
fq is the oscillator strength of the involved absorption transition of the acceptor (Mn
2+),
λS (in angstroms) is the wavelength position of the sensitizer's emission,
E is the energy involved in the transfer (in eV), and ∫
FS(
E)
FA(
E)d
E/
E4 represents the spectral overlap between the normalized shapes of the Eu
2+ emission
FS(
E) and the Mn
2+ excitation
FA(
E), and in our case it is calculated to be about 0.03726 eV
−5. Using the above equation with
fq = 10
−10, the critical distance
RC was estimated to be 11.5 Å.
The quantum efficiency of the obtained phosphors was also measured. For SGP:0.01Eu2+,0.03Mn2+ white-light phosphor, the quantum efficiency (QE) was determined to be 16.7% upon excitation at 355 nm. Therefore, the experimental conditions and the composition of the phosphor must be optimized so that the QE will be further enhanced. Fig. 9 depicts the Commission Internationale de L'Eclairage (CIE) chromaticity coordinates of SGP:0.01Eu2+,nMn2+ phosphors with different doping contents of Mn2+ excited at 355 nm, which were calculated based on the corresponding PL spectrum. Interestingly, in the SGP:0.01Eu2+,nMn2+ phosphors system, as the value of n increases from 0 to 0.08, the photoluminescence color can be easily modulated from green to green-yellow, warm-white, yellow, and eventually to orange, which is due to the different emission composition of the Eu2+ and Mn2+ ions. In particular, at n = 0.03, a warm white light emission with CIE coordinates of (0.401, 0.405) and correlated color temperatures of 3671 K can be obtained in a single host, as shown in point 5 (warm-white light region) in Fig. 9. From the above results, we can clearly see that the emission of the Eu2+ and Mn2+ ions occurs simultaneously and yields a white light emission as a result of partial energy transfer. Hence, this material would be potentially used as a white light emitting source to meet the needs of illumination application.
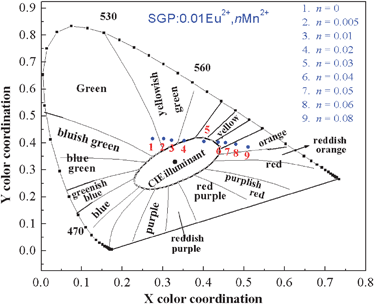 |
| Fig. 9
CIE chromaticity diagram for SGP:0.01Eu2+,nMn2+ phosphors (point 1 to 9) excited at 355 nm. | |
4. Conclusion
In summary, we have succeeded in the synthesis of a warm-white-light Sr3Gd(PO4)3:Eu2+,Mn2+ phosphor system for white LEDs. The efficient energy transfer from the Eu2+ to Mn2+ ions was demonstrated via a dipole–quadrupole mechanism by the fluorescence decay dynamics based on the Inokuti–Hirayama theoretical model. Moreover, the photoluminescence color can be easily modulated from green to green-yellow, warm-white, yellow, and eventually to orange by simply adjusting the content of Mn2+. In particular, a warm-white-light emission with CIE coordinates of (0.401, 0.405) and correlated color temperatures of 3671 K was realized in SGP:0.01Eu2+,0.03Mn2+ phosphor. There results indicate that the developed phosphor exhibits a potential to act as a single-component white-light-emitting phosphor for white LEDs.
Acknowledgements
This work is financially supported by the National Natural Science Foundation of China (Grant No. 20771098) and the Fund for Creative Research Groups (Grant No. 20921002), and the National Basic Research Program of China (973 Program, Grant No. 2007CB935502).
References
- R. J. Xie, N. Hirosaki, T. Suehiro, F. F. Xu and M. Mitomo, Chem. Mater., 2006, 18, 5578 CrossRef CAS.
- Z. Hao, J. Zhang, X. Zhang, X. Sun, Y. Luo, S. Lu and X. Wang, Appl. Phys. Lett., 2007, 90, 261113 CrossRef.
- W. Ding, J. Wang, Z. Liu, M. Zhang, Q. Su and J. Tang, J. Electrochem. Soc., 2008, 155, J122 CrossRef CAS.
- T. S. Chan, R. S. Liu and I. Baginskiy, Chem. Mater., 2008, 20, 1215 CrossRef CAS.
- A. A. Setlur, W. J. Heward, Y. Gao, A. M. Srivastava, R. G. Chandran and M. V. Shankar, Chem. Mater., 2006, 18, 3314 CrossRef CAS.
- Y. Q. Li, A. C. A. Delsing, G. D. With and H. T. Hintzen, Chem. Mater., 2005, 17, 3242 CrossRef CAS.
- M. S. Wang, S. P. Guo, Y. Li, L. Z. Cai, J. P. Zou, G. Xu, W. W. Zhou, F. K. Zheng and G. C. Guo, J. Am. Chem. Soc., 2009, 131, 13572 CrossRef CAS.
- Y. H. Song, G. Jia, M. Yang, Y. J. Huang, H. P. You and H. J. Zhang, Appl. Phys. Lett., 2009, 94, 091902 CrossRef.
- W. J. Yang, L. Luo, T. M. Chen and N. S. Wang, Chem. Mater., 2005, 17, 3883 CrossRef CAS.
- N. Guo, H. P. You, Y. H. Song, M. Yang, K. Liu, Y. H. Zheng, Y. J. Huang and H. J. Zhang, J. Mater. Chem., 2010, 20, 9061 RSC.
- J. Barbier, J. E. Greedan and T. Asaro, Eur. J. Solid State Inorg. Chem., 1990, 27, 855 CAS.
- H. B. Liang, Y. Tao, J. H. Xu, H. He, H. Wu, W. X. Chen, S. B. Wang and Q. Su, J. Solid State Chem., 2004, 177, 901 CrossRef CAS.
-
W. D. Kingery, H. K. Bowen and D. R. Uhlmann, Introduction to Ceramics, Wiley, New York, 1976 Search PubMed.
- C. Chartier, C. Barthou, P. Benalloul and J. M. Frigerio, J. Lumin., 2005, 111, 147 CrossRef CAS.
- S. Ye, J. H. Zhang, X. Zhang, S. Z. Lu, X. G. Ren and X. J. Wang, J. Appl. Phys., 2007, 101, 033513 CrossRef.
- L. A. Shi, Y. L. Huang and H. J. Seo, J. Phys. Chem. A, 2010, 114, 6927 CrossRef CAS.
- H. Jiao, F. Liao, S. Tian and X. Jing, J. Electrochem. Soc., 2003, 150, H220 CrossRef CAS.
- P. I. Paulose, G. Jose, V. Thomas, N. V. Unnikrishnan and M. K. R. Warrier, J. Phys. Chem. Solids, 2003, 64, 841 CrossRef CAS.
- M. Inokuti and F. Hirayama, J. Chem. Phys., 1965, 43, 1978 CrossRef CAS.
- H. P. You, J. L. Zhang, G. Y. Hong and H. J. Zhang, J. Phys. Chem. C, 2007, 111, 10657 CAS.
Footnote |
† Electronic supplementary information (ESI) available. See DOI: 10.1039/c1nj20532c |
|
This journal is © The Royal Society of Chemistry and the Centre National de la Recherche Scientifique 2012 |