DOI:
10.1039/C2LC90022J
(Focus)
Lab Chip, 2012,
12, 1412-1416
Microfluidic diagnostics for the developing world
Received
16th February 2012
, Accepted 16th February 2012
First published on 12th March 2012
Abstract
For more than a decade, it has been expected that microfluidic technology would revolutionize the healthcare industry with simple, inexpensive, effective, and ubiquitous miniature diagnostic devices. To date, however, microfluidics has not yet been able to live up to these expectations. This fact has led to the recent development of new philosophies and methodologies for microfluidic diagnostics. In this Focus article, we will discuss some of the latest breakthroughs that could significantly impact medical diagnostics in the developing world.
Introduction
Cost-effective, high-performance diagnostic methods are vital to providing citizens with accessible, affordable, and high-quality healthcare in both developed and developing countries. It was estimated that diagnostics comprises only 3–5% of healthcare spending, yet it impacts about 70% of healthcare decisions. Therefore, a relatively modest investment in diagnostic technology could potentially lead to drastic improvement of the overall healthcare outcome. This is particularly attractive for developing countries where financial resources are extremely limited. As a result, an important question has been presented to biomedical researchers: how to develop and leverage innovative diagnostic technologies to create exponential impact for healthcare in developing countries?
Obviously there is a major difference in terms of the needs of diagnostics between developing countries and developed countries. While the top killer diseases in developed countries such as the US are heart diseases and cancers, the leading cause of death in developing countries are infectious diseases, the majority of which could be prevented with proper diagnosis and treatment.1 Therefore, infectious diseases have been identified as the top-priority in terms of the development of cost-effective diagnostic methods. Fig. 1 demonstrates the impact of various infectious diseases in terms of disability-adjusted life years (DALYs), which is the years of life lost by a person's premature death. Among the infectious diseases listed, lower respiratory infections, HIV/AIDS, malaria, measles and tuberculosis contribute the highest burden to developing countries. Others such as syphilis also cause significant capital and economical losses. Common diagnostic procedures for these diseases include chemical/biological assays such as enzyme-linked immunosorbent assay (ELISA) and assays that heavily rely on instruments such as flow cytometry.
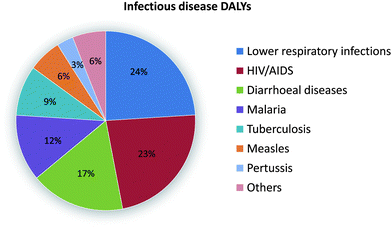 |
| Fig. 1 Disability-adjusted life years (DALYs) for infectious and parasitic diseases. Image reproduced from ref. 1 with permission from Nature publishing. | |
For a diagnostic technology to be successful in the developing world, it is important to follow the guidelines provided by World Health Organization (WHO) which include a set of criteria such as affordability, accessibility, sensitivity, specificity, ease-of-use, speed, and robustness.2 In light of these requirements, it is natural for researchers to turn to microfluidics, because microfluidic techniques inherently use compact, low-cost, low-power, mass-producible fluidic chips designed for sensitive, rapid analysis of minute sample sizes. In addition, various on-chip diagnostic modules have been integrated within the same fluidic chips, providing devices with excellent flexibility and functionality. Therefore, microfluidics could be an extremely attractive platform for providing diagnostic solutions for the developing world.
The expectation that microfluidic technology could revolutionize the healthcare industry with simple, inexpensive, effective, and ubiquitous miniature biomedical diagnostic devices has existed since the conception of microfluidics. Despite tremendous progress in the field in the past decade, it seems that microfluidics has yet to live up to these expectations. Significant challenges still exist. For example, while many research teams have demonstrated complex bio/chemical processes that are necessary for various diagnostic procedures within a single microfluidic chip, in practice these on-chip processes are not possible without the support of bulky and expensive external components, such as pumps and switches for fluid actuation and optical detectors for signal measurement. Therefore, new philosophies for simplified microfluidics are required to make microfluidic-based systems more practical. In addition, transformative technologies are needed to provide solutions for medical diagnostic applications which currently seem unobtainable.
New philosophies in microfluidics
Microfluidics started out with the concept to manipulate minute volumes of sample reagents within a monolithic fluidic chip. Traditionally, most research efforts have been focused on realizing fluidic control within the chip, while fluid-driving mechanisms (such as pumps, switches, and pressure sources) largely existed outside of the chip. This is rather “convenient” for demonstrating technologies in the laboratories; however, it has become increasingly obvious that the entire microfluidic community could suffer from the inability to launch these technologies into a practical setting. Many devices developed so far remain as the “Chip-in-a-Lab” systems rather than the “Lab-on-a-Chip” systems which many of us have hoped for. Fortunately this issue has been seriously examined and several new and quite intriguing philosophies have emerged in the past few years.
Paper microfluidics
Paper microfluidics, pioneered by the Whitesides group, is a perfect example of how innovative low-cost fluidic manipulation methods can free microfluidic devices from the limitation of active pumping and enable extremely low-cost microfluidic diagnostics. In a paper-based microfluidic device, the flow is driven by capillary forces (paper wicking). Hydrophobic barriers such as wax can be easily printed onto the paper to define a microfluidic channel network (Fig. 2A). Besides the benefits of pump-free fluid manipulation, paper is advantageous in many other aspects, such as its light weight, biocompatibility, disposability, compatibility with various biological assays, all of which are ideal for a diagnostic platform.3 Paper is particularly suitable for colorimetric tests which can be detected by the naked eye or simple devices such as a cellular phone. As the smart devices (such as smart phones) equipped with high-resolution cameras become more readily accessible and affordable, paper microfluidics could become a powerful tool to perform many diagnostic tests in the developing world. So far only a few relatively simple laboratory tests have been demonstrated in paper microfluidics such as glucose or protein detection; however a plethora of existing colorimetric technologies used in various test-strips can be leveraged to expand the portfolio of diagnostics using paper microfluidics. In addition, three-dimensional fluidic networks were fabricated in paper4 by patterning multiple layers of paper with fluidic networks (Fig. 2B). It is worth noting that creating a 3D fluidic network in paper is much more convenient than in traditionally monolithic microfluidic devices. This 3D configuration has further expanded the capabilities of paper microfluidics with multiple functional zones and multiplexing tests.
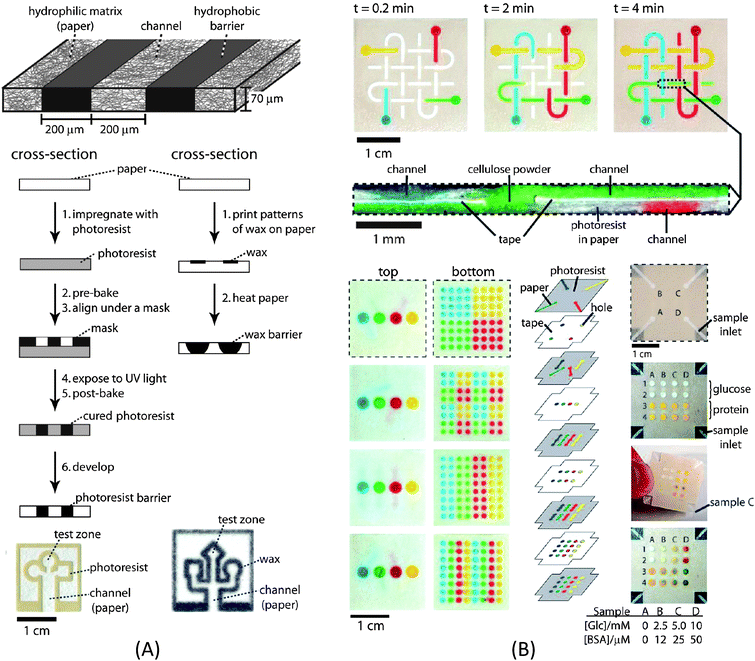 |
| Fig. 2 (A) Schematic of the fabrication process for a paper-based microfluidic channel. (B) Three-dimensional (3D) paper microfluidic channel network and multiplex detection. Image reproduced from ref. 3 and 4 with permission from ACS and the National Academy of Sciences. | |
Creating microfluidic diagnostic devices with a practical mind-set
Solving the current issues of microfluidic diagnostic devices for the developing world does not always mean that one needs to make drastic changes in the technology platform as in paper microfluidics. There is still a great potential in “traditional” microfluidics, if the technology can be implemented with a practical mind-set. For example, the Sia group recently developed a microfluidic platform for the diagnosis of infectious diseases specifically for developing countries.5 In this device (Fig. 3), they demonstrated how new manufacturing, fluid handling, and signal detection procedures can be leveraged to overcome the challenges that we are facing in real-world applications. The authors present a series of innovations in chip development. Firstly, a cost-efficient, high-throughput manufacturing process for microfluidic cassettes (material cost: ∼ 1 US dollar) was developed. Secondly, a “meandering channel” design together with a silver-reduction amplification method were used to amplify the ELISA signal yielded by gold nanoparticle-conjugated antibodies to the extent that it can be easily read by the naked eye or lost-cost optical components such as LEDs and photodetectors (cost per unit: several US dollars). Finally, a simple, elegant, and cost-effective method to deliver multiple reagents into the chip for ELISA tests was achieved by preloading various reagents into a tube as discrete droplets. The sequential loading of samples was as easy as pulling the reagents through the fluidic chip using the vacuum generated manually with a disposable syringe. Such a method is simple yet reliable, and effectively eliminates the need for external pumps and switches, resulting in a dramatic reduction in cost and complexity. These three measures result in a low-cost diagnostic device for ELISA detection of HIV and syphilis that is simple to operate and provides results that can be easily interpreted. The chip has been field-tested in Rwanda on hundreds of locally collected human samples for HIV and syphilis detection, and the results are very promising—with sensitivities and specificities that “rival those of reference bench-top assays.”5
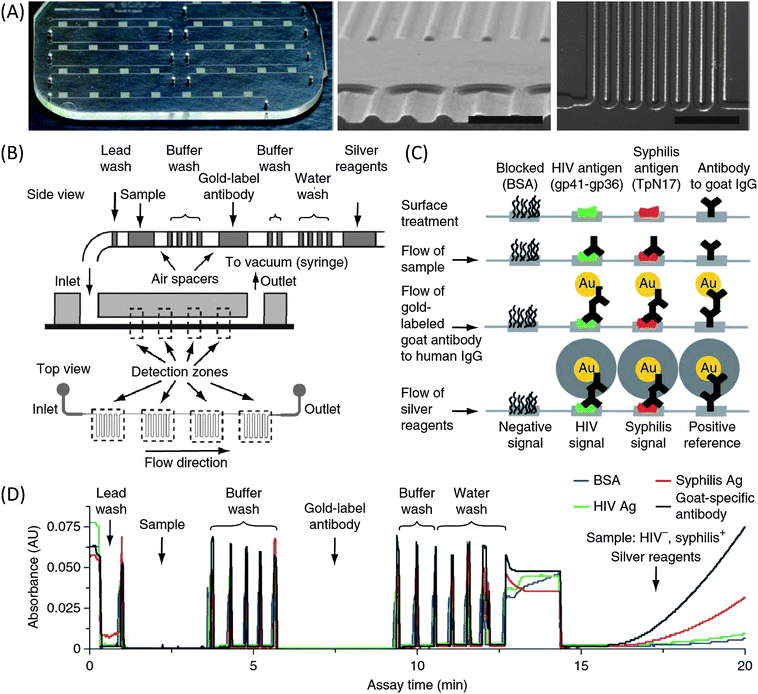 |
| Fig. 3 (A) Images of the microfluidic chip. (B) Schematic diagram of passive delivery of multiple reagents. (C) Illustration of biochemical reactions in detection zones at different immunoassay steps. (D) Absorbance traces of a complete HIV-syphilis duplex test as reagent plugs pass through detection zones. Image reproduced from ref. 5 with permission from Nature Publishing. | |
New technologies by fusing microfluidics with optoelectronics
Examples shown in the previous section were mainly focused on the chemical/biological assays. Another area of particular interest is the development of affordable and miniature diagnostic instruments by combining microfluidic and optoelectronic technologies. By utilizing the unique properties of fluids and piggybacking on the latest optoelectronic advancements, these technologies make it possible to provide cost-effective diagnostic instruments for the developing world.
Recently the Ozcan group shed some light into how novel instrument design can take advantage of both microfluidics and optoelectronic technology to perform diagnosis in the developing world.6 In a recent study, they invented a new technology route that is significantly different from that of traditional flow cytometry. Their device is composed of a fairly simple microfluidic device and a camera system from a cellular phone. By pairing the microfluidic device with the cellular phone, the device is capable of performing fluorescent cell imaging cytometry. Fig. 4 demonstrates the principle of the device. A microfluidic channel is positioned above the cell phone camera. During the operation, fluorescently labeled cells are injected through the channel. Within the fluidic channel, cell suspension is sandwiched between the glass/polymer substrates. Due to the refractive index difference, the cell suspension and glass/polymer effectively form an optofluidic slab-waveguide structure that confines the light within the fluid layer. An inexpensive LED provides the illumination light that is coupled from the sidewalls of the channel and excites the fluorescently labeled cells. Due to the light confinement of the optofluidic slab-waveguide, the illumination light from the LED only propagated laterally along the microfluidic channel, producing an almost perfectly dark background for fluorescent imaging of the cells. Due to the low intensity of the background excitation light, only an inexpensive plastic adsorption filter was needed in front of the cell phone camera lens to completely eliminate the background noise for fluorescent imaging. The fluorescent movies of the cells are captured by the cell phone CMOS and the data can be further processed via a cell phone application to determine the counts of cells in the sample. This cell phone-based imaging cytometer weighs about half an ounce and costs less than five dollars (excluding the cell phone). It has been tested with white blood cells in human blood and the results are comparable to those from a commercial hematology analyzer. Further work is ongoing to evaluate the potential of this system for HIV monitoring in resource-limited countries.
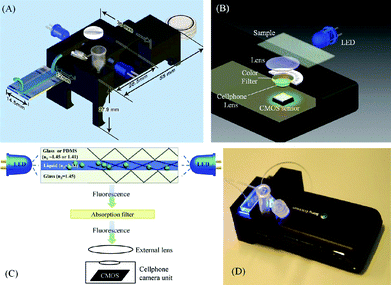 |
| Fig. 4 (A)–(C) Various schematic diagrams of the designed optical attachment for optofluidic fluorescent imaging cytometry on a cell phone are illustrated. (D) The picture of the optofluidic fluorescent imaging cytometer on a cell phone. Image reproduced from ref. 6 with permission from ACS publishing. | |
Conclusions
In summary, the microfluidics community is in need of transformative solutions to dramatically impact the healthcare systems in the developing world. The field of microfluidics is no longer young enough to continue boasting about potential without delivering truly meaningful solutions. In this aspect, recently many innovative researchers have been stepping up to this challenge. We have seen that drastically rethinking what we consider microfluidic technology, as in paper microfluidics, can push the field into new directions. However, approaches that are less dramatic can also go a long way, as we have seen through the integration of microfluidics with everyday/common devices such as cell phones and syringes. The recent advances outlined in this article are intriguing and will continue to inspire others in the field to take bold steps to move microfluidics into a new era in which we produce realistic devices that could truly impact the world.
All opinions are those of the authors and do not reflect the views of Lab on a Chip or the Royal Society of Chemistry.
Acknowledgements
This research was supported by National Institutes of Health (NIH) Director's New Innovator Award (1DP2OD007209-01). The authors thank Michael Lapsley, Sz-Chin Steven Lin, and Brian Kiraly for helpful discussions.
References
- P. Yager, T. Edwards, E. Fu, K. Helton, K. Nelson, M. R. Tam and B. H. Weigl, Microfluidic diagnostic technologies for global public health, Nature, 2006, 442, 412–418 CrossRef CAS
.
- D. Mabey, R. W. Peeling, A. Ustianowski and M. D. Perkins, Diagnostics for the developing world, Nat. Rev. Microbiol., 2004, 2, 231–240 CrossRef CAS
.
- W. W. Martinez, S. T. Phillips, G. M. Whitesides and E. Carrilho, Diagnostics for the developing world: microfluidic paper-based analytical devices, Anal. Chem., 2010, 82(1), 3–10 CrossRef
.
- W. W. Martinez, S. T. Phillips and G. M. Whitesides, Three-dimensional microfluidic devices fabricated in layered paper and tape, Proc. Natl. Acad. Sci. USA, 2008, 19(105), 606–611 Search PubMed
.
- C. D. Chin, T. Laksanasopin, Y. K. Cheung, D. Steinmiller, V. Linder, H. Parsa, J. Wang, H. Moore, R. Rouse, G. Umviligihozo, E. Karita, L. Mwambarangwe, S. L. Braunstein, J. van de Wijgert, R. Sahabo, J. E. Justman, W. El-Sadr and S. K. Sia, Microfluidics-based diagnostics of infectious diseases in the developing world, Nat. Med., 2011, 17(8), 1015–9 CrossRef CAS
.
- H. Zhu, S. Mavandadi, A. F. Coskun, O. Yaglidere and A. Ozcan, Optofluidic Fluorescent Imaging Cytometry on a Cell Phone, Anal. Chem., 2011, 83(17), 6641–7 CAS
.
|
This journal is © The Royal Society of Chemistry 2012 |