DOI:
10.1039/C1FO10068H
(Review Article)
Food Funct., 2012,
3, 10-21
Development of oral food-grade delivery systems: Current knowledge and future challenges
Received
16th May 2011
, Accepted 15th September 2011
First published on 12th October 2011
Abstract
In recent years there has been an increasing interest in the development of new and efficient oral food delivery systems as tools to prevent disease and promote human health and well-being. Such vehicles are sought to protect bioactive ingredients added to food while controlling and targeting their release as they pass through the human gastrointestinal tract (GIT). This review aims to summarize the key concepts of food delivery systems, their characterization and evaluation. Particularly, evaluation of their performance within the human GIT is discussed. To this end an overview of several in vivo and in vitro methods currently applied for the study of such systems is given. Although considered to be still in its infancy, this promising field of research is likely to infiltrate into real products through rational design. In order for such efforts to materialize into real products some challenges still need to be met and are discussed herein. Overall, it seems that adopting a comprehensive pharmacological approach and relevant cutting edge tools are likely to facilitate innovations and help elucidate and perhaps tailor delivery systems' behavior in the human GIT.
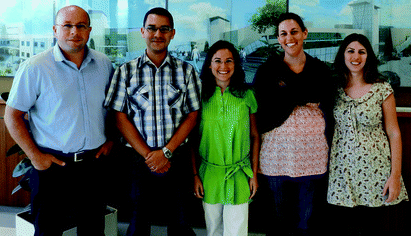 Eyal Shimoni, Uri Lesmes, Revital Cohen Benshitrit, Carmit Shani Levi, Sharon Levi Tal | Revital Cohen Benshitrit is now completing her Ph.D. in the Dept. of Biotechnology & Food Engineering at the Technion – Israel Institute of Technology. Her current research is looking into the delivery of phenolic bioactives, e.g. genistein, to the intestine using starch based delivery systems. She received her B.Sc. (with high honors) in Biotechnology & Food Engineering from the Technion in 2006 and was recently acknowledged as one of the top PhD students in Europe by EFFOST and EFCE. |
| Carmit Shani Levi is currently a Ph.D. candidate in the Dept. of Biotechnology & Food Engineering at the Technion – Israel Institute of Technology. Her current research is looking into the application and development of in vitro gastrointestinal models to study food grade emulsion-based formulations. She obtained her B.Sc.nutr. and R.D from the Hebrew University (Jerusalem, Israel) in 1999. Until 2009, she was a clinical nutritionist in Rambam Medical Center (Haifa, Israel) during that time she also obtained her M.Sc. in Biotechnology from the Technion. |
| Sharon Levi Tal is currently the manager of the Laboratory of Chemistry of Foods and Bioactives in the Dept. of Biotechnology & Food Engineering at the Technion – Israel Institute of Technology. She obtained her B.Sc. in Life Sciences from the Ben Gurion University (Beer-Sheva, Israel) in 2004. She joined the Technion in 2005 and is now completing her PhD on the morphological characterization and structure–function relationship of different biological colloids. |
| Eyal Shimoni is Assoc. Prof. and head of the Laboratory of Functional Foods, Nutraceuticals, and Food Nanoscience in the Dept. of Biotechnology & Food Engineering at the Technion – Israel Institute of Technology. Since 2010 he is also the CTO and Technology VP of Strauss group. He obtained his D.Sc. from the Technion in 1998 and was a post doctoral associate with Prof. Labuza in the Dept. of Food Science & Nutrition at the University of Minnesota, St. Paul, USA. |
| Uri Lesmes is senior lecturer and head of the Laboratory of Chemistry of Foods and Bioactives in the Dept. of Biotechnology & Food Engineering at the Technion – Israel Institute of Technology since 2010. In the past he was a visiting scholar in the Food Biosciences Dept. at Reading University (UK), and a post doctoral research fellow with Prof. McClements in the Food Science Dept. at the University of Massachusetts (Amherst, USA) where he also held a lecturer position. He obtained his Ph.D. in Biotechnology and Food Engineering studying edible starch-based nanocapsules. |
Introduction
Today there is an increasing body of evidence supporting the efficiency and cost-effectiveness of diet and nutrition as tools to promote human health and decrease morbidity. This has generated numerous attempts to develop functional foods that carry added value beyond their mere nutritional value. Such attempts include the enrichment and fortification of foods with bioactive ingredients, such as polyphenols, carotenoids, fatty acids and their derivatives, amino acids, peptides, proteins, vitamins, minerals and even live probiotic bacteria.1 However, the addition of nutraceuticals and micronutrients is a major scientific and technological challenge. Many of these bioactive ingredients are chemically and physically labile to processing conditions as well as digestive reactions. These may lead to deleterious effects on food properties, particularly hindering food sensory properties and limiting the bioaccessibility and bioavailability of the bioactive compounds.
Adapting a pharmacological approach, various efforts have looked in the development of food-grade delivery systems, which offer the possibility to fabricate products that protect bioactives through processing and storage while controlling and targeting their release in the human gastrointestinal tract (GIT).2–5 Recent publications also provide an overview of the links between the structure and functionality of various food delivery systems, highlighting the possibility to harness scientific insights for their rational design and manufacture.2,3,6,7 Furthermore the use of simple and low-cost processes and ingredients in the manufacture of such delivery systems has also been of interest.8 This article will provide an overview of the current knowledge on various food delivery systems, their characterization and functional evaluation.
In general, food grade delivery systems for bioactive ingredients can be classified in diverse ways. Such classifications include classification according to production method (e.g. high energy processing, low energy processing or spontaneous), their physicochemical stability (kinetically or thermodynamically stable, physically or chemically stable), their intended site of delivery (mouth, stomach, small intestine or colon), or their functional sensory properties (e.g.rheology or appearance). To date, size and the main nutritional component of the system appear to be the most commonly used classifications and some main examples are summarized in Fig. 1. Size is frequently used to classify food delivery systems as it is linked to the systems functionality, particularly to the bioactive's bioavailability. Changes in colloid size generally modulate the release of the bioactive, its stability and solubility, as well as its retention in the human GIT, altering the bioavailability of the encapsulated molecules.7,9 Changes in colloid size have also been shown to facilitate transport phenomena, e.g. absorption, and various physiological reactions, such as pepsinolysis of proteins or lipase digestion of lipids.3,10–12 Concomitantly, classification according to the main nutritional component has become very common amongst researchers and has led to four main groups of delivery systems: protein-based, carbohydrate-based, lipid-based, or mixed systems.13–18 Each system has its own benefits and limitations, thus, presenting researchers with a myriad of possibilities and challenges.
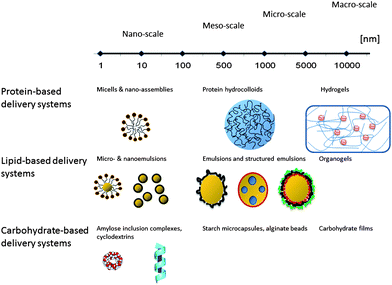 |
| Fig. 1 An outline of currently described food-grade delivery systems. | |
Lipid based delivery systems
One of the most plausible platforms for the delivery of lipophilic bioactives is through lipid-based delivery systems, and particularly emulsion-based delivery systems are suitable for such purposes.3,19,20 Moreover, the use of multiple emulsions in which water droplets are entrapped within oil droplets broaden the scope of lipid delivery systems to even the delivery of water soluble bioactives. Overall, lipid delivery systems are believed to improve bioactive wetting and dissolution properties, modulate bioactive absorption and chemical stability as well as enable targeting of specific tissues.3,19–21 The production conditions and formulation of such systems may influence bioactive solubility and formulation colloidal stability, as well as its behavior in the GIT, which in turn affect the bioactive's bioaccessibility.2,3,20,22,23
In this respect, the development of lipid delivery systems is a highly active field of research. The various insights and accomplishments in this vivid field of research have been recently reviewed in detail by others.3,20,23–28 Particularly, structured emulsion systems have drawn much attention, as those are commonly produced through physical modifications, considered by many to be mild and harmless compared to chemical modifications.3,20,23,26 Such formulations have been shown to have a wide range of sizes (e.g. 5–50 nm for microemulsions or 20–100 nm for nano-emulsions), physical states (e.g. organogels, solid lipid particles or oil droplets), structural and spatial organizations (e.g. liposomes, colloidosomes, multiple or nano-laminated emulsions) and thermodynamic stabilities.
In the case of emulsions, recent studies show that modification of droplet coatings have varying effects not only on emulsion physical and chemical stability but potentially also on its responsiveness to relevant digestive processes, e.g. emulsifier displacement by bile and pancreatic lipase digestion of lipids.10,29–31 For more detailed information on the potential and current understanding of structure–function relationships of emulsion delivery systems targeting the human GI readers are referred to recent reviews.3,23,26,28
Protein based delivery systems
Food
proteins are a versatile group of biopolymers that carry an important nutritional value along with considerable functionality.32,33 Their chemical and structural versatility makes them appropriate candidates for the delivery of bioactive molecules in a wide range of platforms, including molecular coacervates, hydrocolloid particles, emulsion droplet stabilization, hydrogels and films. Indeed, protein based delivery systems have been reported to be relatively simple to prepare, cheap and offer the possibility to deliver hydrophobic or hydrophilic bioactives.32,33 Common proteins investigated for food delivery systems are from animal origin and include gelatin, collagen, casein, albumin and various whey proteins. Also, plant proteins, such as soy glycinin and wheat gliadin, have been reported to be suitable carriers and are preferred by the food industry as they are considered safer.32,33 A wide range of techniques have been reported to be useful in the production of protein-based hydrocolloids, these include extrusion, emulsification, spray drying and coacervation and other processes.9
The ability to control the size of the particles is important both for the functionality of the delivery system and to the products texture, appearance and taste.33 Therefore, various studies have reported the formation of protein-based colloids of varying dimensions.34 For instance, the self-assembly of some milk proteins has been reported to be successful in forming nano-sized delivery vehicles loaded with lipophilic bioactives, such as vitamin D and ω-3 polyunsaturated fatty acids in casein micelles or epigalloctechingallate (EGCG) and resveratrol in β-lactoglobulinnano-vehicles.35–37 Such platforms have been shown to retard the oxidation of the labile ω-3 polyunsaturated fatty acid DHA.38 These versatile delivery vehicles have even been demonstrated to be suitable carriers for hydrophilic bioactives, such as riboflavin.39
Protein hydrogels are used widely in food applications and have received considerable attention as potential delivery systems. The responsiveness of proteins to environmental stressors, e.g. pH, ionic strength and temperature, makes protein-based hydrogels good candidates for responsive systems in which the release of the entrapped bioactive is triggered through stimuli generated by the unique conditions along the human GIT.40 For example, the acidic gastric conditions or the relatively mild conditions of the intestine can affect protein charge and structure depending on the isoelectric point of the protein. Such changes can alter the protein–protein and protein–bioactive interactions and consequently affect the release of the bioactive. Some of the mechanisms underlying the controlled and triggered release of bioactives from protein hydrogels include hydrogel swelling, biodegradation, and bioactive binding properties.9 An example for responsive hydrogels used as delivery systems in the food industry are the soy proteins, which are commonly used due to their functional properties, low cost, availability and high nutritional value.33,41 Overall, the variety of physicochemical properties of proteins, their complex interactions with bioactives, high nutritional value and relative safety offer a myriad of possibilities to develop food grade delivery systems. However, protein delivery systems raise various concerns, particularly regarding issues of allergenicity and off-flavors arising from peptides. Thus, many technological and scientific challenges are yet to be met, as will be discussed later on.
Carbohydrate based delivery systems
Carbohydrates are a significant fraction of various foods, accounting for many of their sensory properties and caloric value. These nutritional components comprise of mono-, oligo- and polysaccharide fractions. Mono and oligosaccharides are often used as constituents of the encapsulating matrix while the structural versatility and site-specific digestion of polysaccharides make them suitable carriers for the targeted and controlled release along the GIT.42 Such studies have shown that release of the bioactive in the intestine can be controlled by pH changes, transit time, the pressure created by peristaltic motion and even enzymatic degradation. Commonly used polysaccharides include plant-derived carbohydrates (e.g.starch, pectin and guar gum), animal-derived carbohydrates (e.g.chitosan, chondroitin sulfate) and carbohydrates derived from other sources (e.g.alginate derived from algae).42,43
Starch and its derivatives have been widely used in a number of processes to fabricate delivery systems for the protection and controlled release of various bioactives.44 High amylose corn starch (HACS) was suggested to be useful as an enteric coating for the microencapsulation of bioactives, protecting them against dissolution in the stomach while releasing them in the small intestine.45 Recently, there have been a growing number of studies looking into the possibility of using carbohydrates to form nano-sized delivery vehicles. Such studies involve the use of amylose or cyclodextrin for the molecular inclusion of hydrophobic bioactives, such as fatty acids,46–51 aroma compounds,52–55 and polyphenolic molecules such as genistein.56,57 Such complexes have been shown to be stable to various processing temperatures, the acidic conditions of the stomach and release their cargo due to pancreatic amylase digestion, which is typical to the small intestine.47,48,51,56,58,59 Additionally, these delivery vehicles have been demonstrated to be efficient in retarding the oxidation of sensitive unsaturated fatty acids.60Cyclodextrins have also been shown to reduce the volatility of aroma compounds, increase solubility, protect against photodegradation and improve sensory quality via masking of bitterness.61 Also, starch molecular complexes with ω-3 fatty acids were reported to be successfully incorporated into breads with minimal adverse effects on the products' sensorial properties and reducing lipid oxidation during baking compared to controls containing free oil.62 To the best of our knowledge, scant data exists on the ability of food-grade carbohydrate delivery systems to exert beneficial effects in vivo. One such example used in vivo rat models to demonstrate that amylose–genistein molecular complexes increase genistein bioavailability compared to non-entrapped genistein.57
To date, attempts to improve the functionality of carbohydrate delivery systems have focused on chemically modifying carbohydrates. One recent study used succinylation of potato starch to retain and stabilize D-limonene.63 Another study used sodium octenylsuccinated starch to form complexes with curcumin leading to an increased curcumin solubility in simulated gastric fluids and limiting its sensitivity to simulated pancreatic fluid, light, oxidation and heat treatments.64 Similarly, hydrophobically modified starch was used to increase water solubility and in vitro anti-carcinogenic activity of curcumin.65 Overall, there seems to be a need for further research into the in vivo performance of carbohydrate delivery systems, their product compatibility and their functionalization through food-grade modifications.
Mixed delivery systems
Many food-grade delivery systems are hindered by over simplification of foods’ compositional and structural complexity, as well as limited by the properties of the carrier substance. In order to improve and broaden the use of delivery systems and potentially tailor their functionality, various efforts have used a more comprehensive approach harnessing various ingredient interactions to form and functionalize delivery systems in realistic settings. In this respect, protein–polysaccharide physical interactions have been widely studied. Some recent studies demonstrate that electrostatic biopolymer interactions can be used to form sub-micron sized hydrocolloids, encapsulate bioactives and modulate delivery systems' susceptibility to digestion.66–68 Such studies show such interactions can be harnessed to modulate emulsion physical and chemical stability.3,20,69–73 Other studies have looked at harnessing natural ‘food-compliant’ chemical reactions in the development of food-grade delivery systems. Particularly, the Maillard reaction has gained much attention as it is wide spread in many foods and considered to be natural, non-extraneous and generally safe.74,75 Relevant studies in the field have shown that Maillard reaction products can be used to enhance and alter the functionality of food proteins and carbohydrates.75,76 Particularly, the application of food-grade Maillard reaction products has been demonstrated to affect emulsion functionality and responsiveness to in vitro digestive events, such as exposure to gastric pepsin.68,75–79 Altogether, formulation, development, and characterization of such and other multi-component systems are more challenging than single component systems. However, today's technology and science make the realization of such ideas within the reach of food scientists and manufacturers.
Physicochemical characterizations
Characterization of delivery systems is imperative for understanding and predicting their functionality, behavior during processing, compatibility with the food matrix, and overall sensory quality of the product. Various structure–function relationships have been described in literature for particulate delivery systems.2,80 These include equations that link structural attributes to functional outcomes such as appearance, rheology and even the controlled release of bioactives. Gathering of relevant structural information is also imperative in this respect, thus, leading to the application of numerous techniques and approaches.7,32 In general, these techniques target specific properties of delivery systems at different structural strata, ranging from molecular level up to micro and macro levels.
Molecular characterizations look into the intra and intermolecular physicochemical properties. Intramolecular properties, such as enthalpies, crystallization events and glass transition have been successfully monitored through differential scanning calorimetry (DSC). Chemical structure and identification has been done through various methods, such as Fourier transform infrared spectroscopy (FTIR), nuclear magnetic resonance (NMR) and mass spectrometry (MS) with crystallinity also studied through various X-ray diffraction technologies (SAXS, WXRD). For example, WXRD, DSC,13C CP/MAS NMR, and SAXS were used to demonstrate how fatty acid chemistry affects the structure of V-amylose nano-capsules and potentially its functionality as a delivery system.47,49 Others used techniques such as FTIR and MS to study molecular level characteristics of food grade delivery systems.81–83 Practically, such molecular analyses are commonly limited to high purity samples or to the use of a reliable separation/purification system. Thus, parallel use of different techniques or more recently application of tandem techniques, e.g.LC-MS, are increasingly being used by researchers.
Microscopic and nano-scopic characterizations are mainly based on light scattering techniques and advanced microscopies. Some available reviews provide discussions on their applicability to study food systems.32,84,85 These techniques are commonly used to study particle size, morphology and colloid instabilities (e.g.flocculation, aggregation), as recently reported for fatty acids–amylose inclusion complexes.47,49,50 Other types of characterizations look more into macro-level properties that are relevant to the consumer, such as product rheology, appearance and other sensory properties. For example, spatial measurement of turbidity, using Turbiscan™ instrumentation, has been successfully implemented to study phase separation and emulsion creaming. Recently, this technique was used during the development of filled hydrogel particles loaded with emulsion droplets.86 Others have described the use of sensory evaluation panels to test the effect of fat viscosity on the in-mouth textural sensory perception of liquid emulsions.87
To put the development of food-based delivery systems on a more quantitative and mechanistic basis attempts have been made to link physicochemical data to various functional properties using different mathematical models and theories, e.g. Mie theory.2,5 Many of the established qualitative structure function relationships (QSFR) address various aspects of product functionality. For example, theoretical stability maps have been described to facilitate the formulation of nano-laminated emulsions.88 This led to the publication of a free downloadable interactive software on The Wolfram Demonstrations Project (http://demonstrations.wolfram.com/ModelForTheFormulationOfMultilayeredEmulsions/), which enable users to enter and modify the model parameters using on-screen sliders and watch their effect on the emulsion stability map almost instantaneously. This contribution is one of the many free downloadable applications in the constantly growing Wolfram demonstrations project, as recently reviewed.89
Although highly relevant, established QSFR mainly focus on functionalities of food hydrocolloids, which are relevant up to their consumption, e.g. appearance, texture and physical stability during production, processing and storage. Overall, it seems that a wide variety of techniques have been successfully implemented to study different structural strata of food delivery systems and establish QSFR for such systems.2,5,7,32 However, we have found scarce data linking findings from different length scales of specific food delivery systems that, for example, will allow predicting macroscopic properties from meso-scopic information. Moreover, delivery systems are more complicated to characterize when they are introduced into a food matrix, since real foods are obviously complex in structure and composition. Thus, it seems that amongst the current challenges faced by food scientists is to establish links between structure and function of delivery systems throughout different length scales, establish their physicochemical and biological fate in the human GI, and to develop new methods to characterize highly complex systems, e.g. application of metabolomic and chemometric analyses.90,91
Evaluating the functionality of food-grade delivery systems
Food grade delivery systems are generally considered to be natural and safe, as they comprise of food grade ingredients, having at least GRAS status, and are produced through relatively mild manufacturing conditions. However, these innovative food-grade delivery vehicles and variable structures raise concerns regarding their proper functionality after ingestion and overall safety, e.g. bioavailability and intestinal permeability.92–95 Therefore, various research efforts have focused on understanding the mechanisms and underlying principles of controlled release systems through a variety of methods. Such methods need to take into account the human gastrointestinal (GI) system, the physiological mechanisms participating in digestion and inter human variability arising from sex, age and other physiological conditions.
Important anatomical and physiological considerations
The overall function of the human GI system is to process ingested materials into molecular species that can be uptaken into the body's circulatory system from the luminal space, which per se is external to the body. This system includes the GIT, i.e. mouth, eosophagus, stomach, intestine and rectum as well as accessory organs, i.e. the salivary glands, lingual glands, liver, gallbladder and exocrine pancreas. Fig. 2 and Table 1 (based on ref. 96–99) summarize the structure of the GI system, its main secretions, functions and relevant anatomical and physiological considerations. From the engineering perspective, food and any orally ingested formulation undergo a series of mechanical and chemical processes in different organs with varying times of retention therein. These processes include chewing, swallowing, peristalsis, mixing, as well as chemical breakdownvia enzymatic reactions in the mouth, stomach and small intestine.100 Also, indigested food reaching the colon can be fermented and further digested by the colon microflora. This metabolism and the prosperity of the colon microflora have been shown to affect humans in health and disease.101,102 Ultimately, bowel contents are excreted viadefecation. This process is important not only to the comfort and well-being of humans but also to their potential compliance to ingest food grade delivery systems.
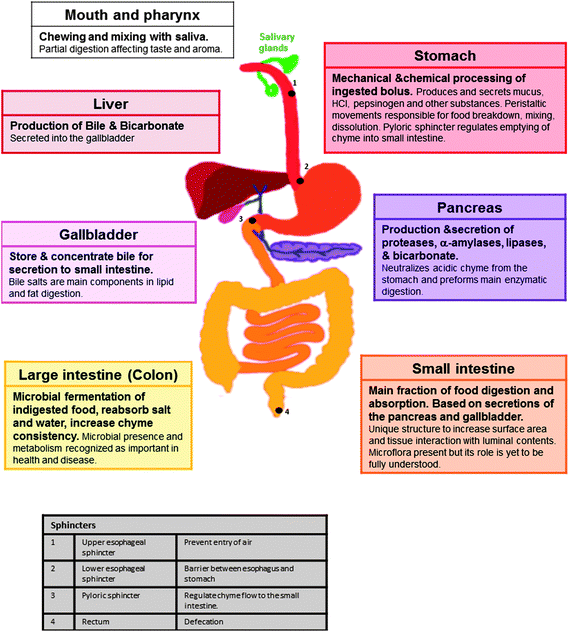 |
| Fig. 2 A summary of the structure of the human gastrointestinal system, its main secretions and functions. Passage of chyme is controlled by peristaltic movements and four sphincters: upper esophageal (1), lower esophageal (2), pyloric (3) and rectum (4). | |
Table 1 Important anatomical and physiological parameters of the human gastrointestinal tract, see ref. 96–99
|
Anatomical dataa |
Daily secretion volume (L) |
Transit time[h] for solid mealb |
pH |
Microflora [CFU ml−1 or CFU g−1] |
Anatomical data relate to a living person when the muscles are in a state of tonus. It is longer in a cadaver.
Transit times may vary between individuals depending on physiological conditions, sex, age, mental state, time of day, and food properties, e.g. total amount, composition, water content, see refs.
|
Mouth |
|
1–1.5 |
|
6.35–6.85 |
N/A |
Stomach |
Capacity 1–1.6 L |
2 |
3.0–3.6 |
2–3 |
0–103 |
Small intestine |
Length 3m |
Bile 0.8–1 |
3.8–4.6 |
6–7.7 |
105–107 |
Diameter 2.5 cm |
Pancreatic 1.2–1.5 |
|
|
|
Surface area ∼600m2 |
Intestinal 1–2 |
|
|
|
Large intestine |
Lengths 1.5 m |
N/A |
18–22 |
5.5–7.6 |
109–1012 |
Diameter 6.5 cm |
|
|
|
|
Total |
6–7 m |
|
|
N/A |
|
In respect to food delivery systems, it is also important to understand that absorption of nutrients and nutraceuticals may occur along different parts of the GIT. Thus, it is important to take note that absorption sites and absorption capabilities may vary according to physiological and chronicle age and might even be affected by the consumption of various food components.103–106 In general, medical literature107,108 indicates that small molecular species (e.g.water, ions and alcohol) are taken up in the stomach while higher molecular species liberated from food (e.g.monosaccharides, monoglycerides, cholesterol, medium and long chain fatty acids and various vitamins) and re-absorption of GI secretions (i.e. bile components) occur through various active and passive mechanisms along the small intestine. The colonic role in adsorption is considered to be limited but not restricted to the absorption of water, electrolytes, vitamin K, biotin and microbial fermentation metabolites, e.g. short chain fatty acids.
From ingestion to defecation, food delivery systems passing through the GI are expected to encounter a variety of environmental stressors, which affect their physicochemistry and, consequently, their functionality.107,109 These changes may have deleterious effects on the delivery of the cargo or through rational design be harnessed for efficient and targeted delivery. In general, delivery vehicles are expected to undergo four sequential digestive processes, which are also spatially divided to specific organs, as described in detail elsewhere.107,109 The first process is mastication, which involves shearing, mixing and dilution with saliva to form a mixture that passes through the esophagus into the stomach. This processing step exposes food to α-amylases, lingual lipase and other substances.110 This stage is important both to the perception of food in the mouth and to the later processes as it reduces the food to a soft, flexible, easily swallowed mass called bolus. The second process is gastric digestion, occurring in the four regions of the stomach: cardia, fundus, body and pylorus. Each region performs different functions, however, overall gastric processing is considered to be a result of highly acidic conditions, enzymatic secretions and distinct mechanical processing. A muscular sphincter controls the passing of partially digested food, now termed chyme or digesta, into the small intestine where the third digestion process occurs. Herein the chyme is mixed with secretions of the gall bladder and the exocrine pancreas, which elevate luminal pH, deactivate gastric enzymes and introduce new digestive components, e.g. enzymes and bile salts. The small intestine comprises of three structurally distinct loci: duodenum, jejunum and ileum all, with unique structures that provide a large surface area for efficient absorption of substances to the liver via the hepatic portal circulation. Unabsorbed chyme is then transferred into the colon where it undergoes the fourth and last digestive process. This process involves reuptake of water and minerals as well as fermentation of indigested chyme by the rich colon microflora leading to an increase in the consistency of colonic contents until voluntary defecation.
The chemical processes of digestion are performed by a variety of substances secreted by accessory organs and specialized cells lining the digestive tract. A summary of the main secreted enzymes involved in digestion and their localization are given in Table 2 (based on ref. 107,108,111). All along the GIT, mucin is secreted to varying extents. This substance is a major component of mucus that modulates the rheological behavior of solutions and the mucus gel layer lining the GIT. This is considered to be an important feature of the mucosal layer that acts as a protective barrier, e.g. protection against proteolytic digestion of the underlying epithelium by pepsin in the stomach. Also, secretion of bicarbonate into this layer appears to play an important role in damping rapid changes of luminal pH.112,113 Gallbladder secretions contain bile, which plays a significant role in lipid digestion and absorption with bile salts and lecithin being two significant components. The pancreatic secretions contain various digestive enzymes, which perform further enzymatic digestion of chyme, and bicarbonate, which neutralizes the acidity of gastric chyme and deactivates gastric enzymes.100 It is also important to note that digestive enzymes can also be found in foodper se affecting the processes of digestion. Thus, evaluation of food grade delivery systems needs to properly address the variety of physical and chemical processes of the human GI. For this purpose, studies have looked at the functionality of such systems both in vivo and in vitro.
Table 2 Main enzymatic secretions and their function in the human gastrointestinal tract, see ref. 107,108,111
In vivo methods
The ultimate test for delivery systems is in vivo performance, which makes such studies and particularly human trials a true necessity. To date, in vivo studies have used various animal models and human trials based on various experimental designs, e.g. double blind and placebo controlled. Such studies have been mainly used to evaluate the efficacy of delivery systems and understand the various factors that influence the rate and extent of bioactive absorption after oral intake.114 Pharmacological studies have already shown that inter human variability complicates studies looking into the availability and digestibility of drugs in the human digestive system, leading to the common use of animal models.114–116 These illustrate the differences between GITs of various laboratory animals and the implications of these differences on the availability and digestibility of drugs in the human digestive system. Accordingly, small animals, e.g. rats, mice, guinea pigs, rabbits, are most commonly used for determining the mechanisms of drug absorption and bioavailability values from powder or solution formulations while larger animals, e.g. dogs, pigs, monkeys, are used to assess absorption from real formulations. For example, rats were recently used to demonstrate that the molecular inclusion of genistein in amylose complexes increased its bioavailability.57 Moreover, there are a variety of methods that have been used to evaluate bioavailability including: radioisotope labeling, stable isotopes, metabolic balance, rate of repletion, and plasma appearance.117 Despite the necessity of in vivo experiments, they are complicated, time consuming, costly, have low throughput of formulations and raise significant ethical issues. For these and other reasons much effort has been invested in the development and application of in vitro models.
In vitro methods
Various in vitro models of parts or the entire human GIT accounting for many of the parameters given in Tables 1 and 2 have been described in the literature.10,118–121 These models, focused mainly on luminal processes, enable researchers and drug formulators to address the physicochemical changes a product undergoes during digestion. Recently, such and other models have been applied and developed in food research and development. This section will provide an overview of such in vitro models.
In the early 1990s a comprehensive in vitro GIT model, termed the simulator of the human intestinal microbial ecosystem (SHIME), was developed by Molly et al.122 This computer controlled multi-chamber set of reactors simulates the conditions of the stomach, duodenum and jejunum, ileum, caecum and ascending colon, transverse colon and descending colon. Operators can control the concentration of enzymes and bile, pH, temperature, feed composition, transit time and anaerobic environment in each reactor, thus enabling modeling of the kinetics of chyme passage through the GIT. The SHIME can be applied for various studies, including evaluating the efficacy and behavior of controlled release systems in the human GIT.
Another comprehensive model was developed during the 1990s by Havenaar and Minekus.123 This model is actually comprised of two separate models, the TIM1 and TIM2 models. TIM1 simulates the conditions of stomach, duodenum, jejunum, and ileum and TIM2 simulates the large intestine. As with the SHIME, this model is also a computer controlled and validated in vitro model with minimal ethical issues and relatively easy operation. Unlike the SHIME, this model can simulate peristaltic movements, controlled squeezing, nutrients and water absorption. Similarly, a three stage continuous fermentation system simulating the proximal, transverse and distal colon was developed and validated against sudden death victims in the late 1990s.124
Despite the potential and the comprehensive nature of the SHIME and TIM models, their limited throughput and accessibility to food researchers and manufacturers around the globe limit their use. Moreover, these models have their share of limitations. One being the fact they do not include an oral processing phase, which is critical for the close imitation of real chyme entering the stomach. Another drawback has been pointed out by Mattila-Sandholm et al.125 who found that both the TIM and SHIME models did not successfully predict survival of some probiotic bacteria with known clinical survival. Thus, other simpler, high throughput, more targeted and specific models for food applications have been sought and described in the literature.118
The role of oral processing of food delivery systems is vital to the product's sensorial perception, its acceptability by the consumer and functionality after swallowing. Such systems are based on pharmacological concepts of drug, delivery; however they have to stand up to different sensorial and regulatory standards. In the field of pharmacology, various models have been described to evaluate the performance of aerosolized drugs. Although a standard USP (United States Pharmacopeia) mouth–throat model exists, other models have been shown to have improved capabilities to characterize particle bounce and predict in vivo deposition of aerosolized drug formulations.126,127 These models do not replicate mastication, saliva secretion and swallowing, thus making them less suitable for food-related studies. In this respect, an in vitro mouth model was recently developed and used to study diffusion phenomena in model hydrogel food systems.128 In this study, researchers successfully applied mathematical modeling and MATLAB programming to fit experimental and theoretical values to calculate the oral diffusivity of salts based on the diffusion eqn (1)
| 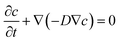 | (1) |
This and other results led to the conclusion that compression and fracture of food hydrogels are key oral events affecting the release of salts that may influence taste perceptions. There still seems to be a significant gap in the development and application of in vitro oral models for food research. As the stomach is a major site for food digestion, recently two unique in vitro models closely mimicking gastric anatomy and peristaltic movementshave been described.119,120 A stomach model developed at the Institute of Food Research (Norwich, UK) is the Dynamic Gastric Model (DGM). This model converts bolus into chyme and is composed of a main body (dynamic addition of gastric juices), antrum (peristaltic movements) and a valve that delivers the chyme into the “duodenum”.120 Another similar model is the Human Gastric Simulator (HGS) developed at UC-Davis.119 These models have been successfully applied to study emulsion behavior under in vitro gastric conditions.120,129 The HGS was also used to study the digestion of solid food matrices, for example the digestion of carrots.130 Moreover, recent efforts have used computerized fluid dynamics to elucidate the rheological behavior of various hydrocolloids in the stomach.131,132 Such simulations have shed light into the effects of colloid size and viscosity on the behavior of chyme in the stomach. Following a similar approach, a simulated small intestinal model was devised to enable controlled motion and segmentation.133 This model enabled researchers to study luminal mass transfer and demonstrate that segmentation motion significantly increases the mass transfer coefficient in the luminal side.
Besides the various apparatus available for in vitrodigestion studies, various biochemical models have also been used for mechanistic studies of specific physiological reactions, e.g. gastric pepsinolysis or small intestinal lipolysis.118 Based on such and other models, we now know quite a bit on the colloidal aspects of protein and lipid digestion, as reviewed elsewhere.11,23,26 For example, simulated gastric fluid has been used to study the various colloidal aspects of protein digestion.11 Such studies have shown that emulsification might alter protein digestibility and certain whey proteins may even be digested differently in infants compared to adults.134,135 Such biochemical models have also been used to elucidate protein-stabilized emulsions' responsiveness to GI conditions.23,30,31,136,137 Similarly, a pH-stat biochemical model was developed and applied to the study how various interfacial modifications of emulsion droplets, e.g. altering charge, composition, structure and even interfacial crosslinking, can modulate the susceptibility of lipid droplets to pancreatic lipase in simulated small intestinal fluid.10,29,30,70,78
In spite of the valuable scientific insights gained through in vitro models of the GI, all of these models are hindered by their lack of ability to fully mimic the GI system, particularly the dynamics of feedback mechanisms and mucosal uptake/absorption, peristaltic movements and involvement of immune components. In vitro models provide an adequate and flexible platform for mechanistic studies of delivery systems' functionality; however, none of these can truly replace in vivo experiments. Overall, in vitro GIT models are relatively cheap, high throughput, raise minimal ethical issues and provide researchers controllable settings for understanding the principles underlying the functionality of delivery systems. Moreover, they emphasize the great potential of adapting the knowledge and established expertise of pharmacological models to help develop and materialize the field of food delivery systems into effective and realistic food products.
Future challenges
Food grade delivery systems are gaining increasing attention as potential natural and cost-effective tools for the promotion of human health as well as the prevention, relief, and treatment of various diseases, such as obesity, cancer, diabetes, neurodegenerative disease (Alzheimer and Parkinson) and others. Adopting a cutting edge pharmacological approach, the development of food delivery systems will heavily rely on researchers' ability to cope with their complexity and that of the corresponding experimental data sets. In this respect, it seems that the application of food delivery systems to realistic food products is still in its infancy. To the best of our understanding, fulfilling the promise encompassed in the concept of rationally designing food delivery systems to optimize human health and well-being still needs to overcome at least three hurdles:
1. Establishing structure–function relationships of
food
delivery systems, particularly looking into the fate of delivery systems within the human
GIT
. Recent work has provided valuable insights linking structure to the various functional aspects of delivery systems, e.g.rheology and appearance. However, limited work has been published on basic principles underlying the functionality of such systems within the GIT. Particularly, such studies should look into important aspects, such as bioaccesibility, bioactivity, bioavailability and overall safety, as recently advocated.2
2. Deciphering the secrets behind sensory properties. In order for food delivery systems to be truly adopted by the consumer they should be tasty and even pleasurable. Future research should continue current efforts to elucidate the principles that link the properties of food delivery systems to their product compatibility and overall effect on the product's sensorial properties.
3. Coping with the human variance and complexity of the digestive process. Development of tools for personalized nutrition, identification of relevant biomarkers and better understanding of the digestive processes is needed to help cope with the known genetic and phenotypic variance of the human population. This may be achievable using chemometric and metabolomic analyses, as suggested by others.90,91
In conclusion, promoting human health and prevention of disease seems to be attainable through rational design and personalization of functional foods and food delivery systems. In order to exploit the great potential of food delivery systems, further research is needed to address their complex and heterogeneous nature as well as their physicochemical and biological fate inside the human GIT.
References
- L. Sagalowicz and M. E. Leser, Delivery systems for liquid food products, Curr. Opin. Colloid Interface Sci., 2010, 15(1–2), 61–72 CrossRef CAS.
- U. Lesmes and D. J. McClements, Structure–function relationships to guide rational design and fabrication of particulate food delivery systems, Trends Food Sci. Technol., 2009, 20(10), 448–457 CrossRef CAS.
- D. J. McClements and Y. Li, Structured emulsion-based delivery systems: Controlling the digestion and release of lipophilic food components, Adv. Colloid Interface Sci., 2010, 159(2), 213–228 CrossRef CAS.
- Y. Shoji and H. Nakashima, Nutraceutics and delivery systems, J. Drug Targeting, 2004, 12(6), 385–91 CrossRef CAS.
- K. P. Velikov and E. Pelan, Colloidal delivery systems for micronutrients and nutraceuticals, Soft Matter, 2008, 4(10), 1964–1980 RSC.
- D. J. McClements, E. A. Decker and Y. Park, Controlling Lipid Bioavailability through Physicochemical and Structural Approaches, Crit. Rev. Food Sci. Nutr., 2009, 49(1), 48–67 CrossRef.
- J. Ubbink, A. Burbidge and R. Mezzenga, Food structure and functionality: a soft matter perspective, Soft Matter, 2008, 4(8), 1569–1581 RSC.
-
G. A. Reineccius, Controlled release techniques in the food industry. In Encapsulation and controlled release of food ingredients, Risch, S. J.; Reineccius, G. A., ed. American Chemical Society: Washington, DC, 1995; pp 8–25 Search PubMed.
-
M. Subirade; L. Chen, Food-protein-derived materials and their use as carriers and delivery systems for actives food components, in Delivery and controlled release of bioactives in foods and nutraceuticals, ed. N. Gart, Woodhead Publishing, Cambridge, 2008, pp 251–278 Search PubMed.
- Y. Li, M. Hu and D. J. McClements, Factors affecting lipase digestibility of emulsified lipids using an in vitro digestion model: Proposal for a standardised pH-stat method, Food Chem., 2011, 126(2), 498–505 CrossRef CAS.
- A. Mackie and A. Macierzanka, Colloidal aspects of protein digestion, Curr. Opin. Colloid Interface Sci., 2010, 15(1–2), 102–108 CrossRef CAS.
- D. J. McClements, E. A. Decker, Y. Park and J. Weiss, Designing food structure to control stability, digestion, release and absorption of lipophilic food components, Food Biophys., 2008, 3(2), 219–228 CrossRef.
- A. Benichou, A. Aserin and N. Garti, Protein-polysaccharide interactions for stabilization of food emulsions, J. Dispersion Sci. Technol., 2002, 23(1–3), 93–123 CAS.
- P. Burey, B. R. Bhandari, T. Howes and M. J. Gidley, Hydrocolloid gel particles: Formation, characterization, and application, Crit. Rev. Food Sci. Nutr., 2008, 48(5), 361–377 CrossRef CAS.
- Y. Y. Xiao, Y. M. Song, Z. P. Chen and Q. N. Ping, Preparation of silymarin proliposome: A new way to increase oral bioavailability of silymarin in beagle dogs, Int. J. Pharm., 2006, 319(1–2), 162–168 CAS.
- S. L. Kosaraju, R. Weerakkody and M. A. Augustin, In vitro evaluation of hydrocolloid-based encapsulated fish oil, Food Hydrocolloids, 2009, 23(5), 1413–1419 CrossRef CAS.
- Y. Li and D. J. McClements, Controlling lipid digestion by encapsulation of protein-stabilized lipid droplets within alginate-chitosan complex coacervates, Food Hydrocolloids, 2011, 25(5), 1025–1033 CrossRef CAS.
- T. M. Taylor, P. M. Davidson, B. D. Bruce and J. Weiss, Liposomal nanocapsules in food science and agriculture, Crit. Rev. Food Sci. Nutr., 2005, 45(7–8), 587–605 CrossRef CAS.
- D. J. McClements, E. A. Decker and J. Weiss, Emulsion-based delivery systems for lipophilioc bioactive components, J. Food Sci., 2007, 72(8), R109–R124 CrossRef CAS.
- D. J. McClements, Design of Nano-Laminated Coatings to Control Bioavailability of Lipophilic Food Components, J. Food Sci., 2010, 75(1), R30–R42 CrossRef CAS.
- P. M. Bummer, Physical chemical considerations of lipid-based oral drug delivery-Solid lipid nanoparticles, Crit. Rev. Ther. Drug Carrier Syst., 2004, 21(1), 1–19 CrossRef CAS.
- N. C. Borges, A. Mazuqueli, R. A. Moreno, R. B. Astigarraga, C. E. Sverdloff, P. A. R. Galvinas, M. R. D. Sampaio and W. M. da Silva, Cyproterone Acetate Quantification in Human Plasma by High-performance Liquid Chromatography Coupled to Atmospheric Pressure Photoionization Tandem Mass Spectrometry Application to a comparative pharmacokinetics study, Arzneim. Forsch., 2009, 59(7), 335–344 CAS.
- H. Singh, A. Q. Ye and D. Horne, Structuring food emulsions in the gastrointestinal tract to modify lipid digestion, Prog. Lipid Res., 2009, 48(2), 92–100 CrossRef CAS.
- E. Dickinson, Food emulsions and foams: Stabilization by particles, Curr. Opin. Colloid Interface Sci., 2010, 15(1–2), 40–49 CrossRef CAS.
- N. E. Hughes, A. G. Marangoni, A. J. Wright, M. A. Rogers and J. W. E. Rush, Potential food applications of edible oil organogels, Trends Food Sci. Technol., 2009, 20(10), 470–480 CrossRef CAS.
-
D. J. McClements, Emulsion Design to Improve the Delivery of Functional Lipophilic Components, in Annual Review of Food Science and Technology, Vol 1, Annual Reviews, Palo Alto, 2010; Vol. 1, pp 241–269 Search PubMed.
-
N. Garti and I. Y. Amar, Micro- and nano-emulsions for delivery of functional food ingredients, in Delivery and controlled release of bioactives in foods and nutraceuticals, ed. N. Garti, Woodhead Publishing, Cambridge, 2008, pp 149–183 Search PubMed.
- D. J. McClements and J. Rao, Food-Grade Nanoemulsions: Formulation, Fabrication, Properties, Performance, Biological Fate, and Potential Toxicity, Crit. Rev. Food Sci. Nutr., 2011, 51(4), 285–330 CrossRef CAS.
- M. Hu, Y. Li, E. A. Decker, H. Xiao and D. J. McClements, Influence of Tripolyphosphate Cross-Linking on the Physical Stability and Lipase Digestibility of Chitosan-Coated Lipid Droplets, J. Agric. Food Chem., 2009 Search PubMed.
- U. Lesmes, P. Baudot and D. J. McClements, Impact of Interfacial Composition on Physical Stability and In Vitro Lipase Digestibility of Triacylglycerol Oil Droplets Coated with Lactoferrin and/or Caseinate, J. Agric. Food Chem., 2010, 58(13), 7962–7969 CrossRef CAS.
- A. Sarkar, D. S. Horne and H. Singh, Interactions of milk protein-stabilized oil-in-water emulsions with bile salts in a simulated upper intestinal model, Food Hydrocolloids, 2010, 24(2–3), 142–151 CrossRef CAS.
- D. M. A. M. Luykx, R. J. B. Peters, S. M. van Ruth and H. Bouwmeester, A review of analytical methods for the identification and characterization of nano delivery systems in food, J. Agric. Food Chem., 2008, 56(18), 8231–8247 CrossRef CAS.
- L. Y. Chen, G. E. Remondetto and M. Subirade, Food protein-based materials as nutraceutical delivery systems, Trends Food Sci. Technol., 2006, 17(5), 272–283 CrossRef CAS.
- Y. D. Livney, Milk proteins as vehicles for bioactives, Curr. Opin. Colloid Interface Sci., 2010, 15(1–2), 73–83 CrossRef CAS.
- L. Liang, H. A. Tajmir-Riahi and M. Subirade, Interaction of β-Lactoglobulin with Resveratrol and its Biological Implications, Biomacromolecules, 2007, 9(1), 50–56 CrossRef.
- E. Semo, E. Kesselman, D. Danino and Y. D. Livney, Casein micelle as a natural nano-capsular vehicle for nutraceuticals, Food Hydrocolloids, 2007, 21(5–6), 936–942 CrossRef CAS.
- A. Shpigelman, G. Israeli and Y. D. Livney, Thermally-induced protein-polyphenol co-assemblies: beta lactoglobulin-based nanocomplexes as protective nanovehicles for EGCG, Food Hydrocolloids, 2010, 24(8), 735–743 CrossRef CAS.
- P. Zimet, D. Rosenberg and Y. D. Livney, Re-assembled casein micelles and casein nanoparticles as nano-vehicles for [omega]-3 polyunsaturated fatty acids, Food Hydrocolloids, 2011, 25(5), 1270–1276 CrossRef CAS.
- L. Chen and M. Subirade, Alginate-whey protein granular microspheres as oral delivery vehicles for bioactive compounds, Biomaterials, 2006, 27(26), 4646–4654 CrossRef CAS.
- P. Gupta, K. Vermani and S. Garg, Hydrogels: from controlled release to pH-responsive drug delivery, Drug Discovery Today, 2002, 7(10), 569–579 CrossRef CAS.
- A. Maltais, G. E. Remondetto and M. Subirade, Soy protein cold-set hydrogels as controlled delivery devices for nutraceutical compounds, Food Hydrocolloids, 2009, 23(7), 1647–1653 CrossRef CAS.
- S. L. Kosaraju, Colon targeted delivery systems: review of polysaccharides for encapsulation and delivery, Crit. Rev. Food Sci. Nutr., 2005, 45(4), 251–8 CrossRef CAS.
- M. A. Augustin and Y. Hemar, Nano- and micro-structured assemblies for encapsulation of food ingredients, Chem. Soc. Rev., 2009, 38(4), 902–912 RSC.
-
E. Shimoni, Starch as an encapsulation material to control digestion rate in the delivery of active food components, in Delivery and controlled release of bioactives in foods and nutraceuticals, ed. N. Garti, Woodhead Publishing, Cambridge, 2008, pp 279–293 Search PubMed.
- A. Dimantov, M. Greenberg, E. Kesselman and E. Shimoni, Study of high amylose corn starch as food grade enteric coating in a microcapsule model system, Innovative Food Sci. Emerging Technol., 2004, 5(1), 93–100 CrossRef CAS.
- M. C. Godet, V. Tran, P. Colonna, A. Buleon and M. Pezolet, Inclusion/exclusion of fatty acids in amylose complexes as a function of the fatty acid chain length, Int. J. Biol. Macromol., 1995, 17(6), 405–408 CrossRef CAS.
- U. Lesmes, S. H. Cohen, Y. Shener and E. Shimoni, Effects of long chain fatty acid unsaturation on the structure and controlled release properties of amylose complexes, Food Hydrocolloids, 2009, 23(3), 667–675 CrossRef CAS.
- J. A. Putseys, L. Lamberts and J. A. Delcour, Amylose-inclusion complexes: Formation, identity and physico-chemical properties, J. Cereal Sci., 2010, 51(3), 238–247 CrossRef CAS.
- S. Zabar, U. Lesmes, I. Katz, E. Shimoni and H. Bianco-Peled, Studying Different Dimensions of Amylose-Long Chain Fatty Acid Complexes: Molecular, Nano and Micro Level Characteristics, Food Hydrocolloids, 2009, 23(7), 1918–1925 CrossRef CAS.
- S. Zabar, U. Lesmes, I. Katz, E. Shimoni and H. Bianco-Peled, Structural characterization of amylose-long chain fatty acid complexes produced via the acidification method, Food Hydrocolloids, 2010, 24(4), 347–357 CrossRef CAS.
- F. Tufvesson, M. Wahlgren and A. C. Eliasson, Formation of amylose lipid complexes and effect of temperature treatment. Part 2: Fatty acids, Starch/Staerke, 2003, 55, 138–149 CrossRef CAS.
- B. Biais, P. Le Bail, P. Robert, B. Pontoire and A. Buléon, Structural and stoichiometric studies of complexes between aroma compounds and amylose. Polymorphic transitions and quantification in amorphous and crystalline areas, Carbohydr. Polym., 2006, 66(3), 306–315 CrossRef CAS.
- C. Jouquand, V. Ducruet and P. Le Bail, Formation of amylose complexes with C6-aroma compounds in starch dispersions and its impact on retention, Food Chem., 2006, 96(3), 461–470 CrossRef CAS.
- G. Decock, S. Fourmentin, G. G. Surpateanu, D. Landy, P. Decock and G. Surpateanu, Experimental and theoretical study on the inclusion compounds of aroma components with beta-cyclodextrins, Supramol. Chem., 2006, 18(6), 477–482 CrossRef CAS.
- G. Decock, D. Landy, G. Surpateanu and S. Fourmentin, Study of the retention of aroma components by cyclodextrins by static headspace gas chromatography, J. Inclusion Phenom. Macrocyclic Chem., 2008, 62(3–4), 297–302 CrossRef CAS.
- R. Cohen, I. Oriova, M. Kovalev, Y. Ungar and E. Shimoni, Structural and Functional Properties of Amylose Complexes with Genistein, J. Agric. Food Chem., 2008, 56(11), 4212–4218 CrossRef CAS.
- R. Cohen, B. Schwartz, I. Peri and E. Shimoni, Improving Bioavailability and Stability of Genistein by Complexation with High-Amylose Corn Starch, J. Agric. Food Chem., 2011, 59(14), 7932–7938 CrossRef CAS.
- B. Conde-Petit, F. Escher and J. Nuessli, Structural features of starch-flavor complexation in food model systems, Trends Food Sci. Technol., 2006, 17(5), 227–235 CrossRef CAS.
- G. G. Gelders, J. P. Duyck, F. Goesaert and J. A. Delcour, Enzyme and acid resistance of amylose-lipid complexes differing in amylose chain length, lipid and complexation temperature, Carbohydr. Polym., 2005, 60(3), 379–389 CrossRef CAS.
- I. Lalush, H. Bar, I. Zakaria, S. Eichler and E. Shimoni, Utilization of amylose-lipid complexes as molecular nanocapsules for conjugated linoleic Acid, Biomacromolecules, 2005, 6(1), 121–30 CrossRef CAS.
- H. M. C. Marques, A review on cyclodextrin encapsulation of essential oils and volatiles, Flavour Fragrance J., 2010, 25(5), 313–326 CrossRef.
- V. Gökmen, B. A. Mogol, R. B. Lumaga, V. Fogliano, Z. Kaplun and E. Shimoni, Development of functional bread containing nanoencapsulated omega-3 fatty acids, J. Food Eng., 2011, 105(4), 585–591 CrossRef.
- S. W. Lee, S. Y. Kang, S. H. Han and C. Rhee, Influence of modification method and starch concentration on the stability and physical properties of modified potato starch as wall materials, Eur. Food Res. Technol., 2009, 228(3), 449–455 CrossRef CAS.
- E. I. Paramera, S. J. Konteles and V. T. Karathanos, Stability and release properties of curcumin encapsulated in Saccharomyces cerevisiae, beta-cyclodextrin and modified starch, Food Chem., 2011, 125(3), 913–922 CrossRef CAS.
- H. L. Yu and Q. R. Huang, Enhanced in vitro anti-cancer activity of curcumin encapsulated in hydrophobically modified starch, Food Chem., 2010, 119(2), 669–674 CrossRef CAS.
- E. Dickinson, Interfacial structure and stability of food emulsions as affected by protein-polysaccharide interactions, Soft Matter, 2008, 4(5), 932–942 RSC.
- I. Peinado, U. Lesmes, A. Andres and J. D. McClements, Fabrication and Morphological Characterization of Biopolymer Particles Formed by Electrostatic Complexation of Heat Treated Lactoferrin and Anionic Polysaccharides, Langmuir, 2010, 26(12), 9827–9834 CrossRef CAS.
- C. M. Oliver, M. A. Augustin and L. Sanguansri, Maillard-based casein-carbohydrate microcapsules for the delivery of fish oil: emulsion stability during in vitro digestion, Aust. J. Dairy Technol., 2009, 64(1), 80–83 CAS.
- M. A. Augustin and Y. Hemar, Nano- and micro-structured assemblies for encapsulation of food ingredients, Chem. Soc. Rev., 2009, 38, 902 RSC.
- V. Gudipati, S. Sandra, D. J. McClements and E. A. Decker, Oxidative Stability and in Vitro Digestibility of Fish Oil-in-Water Emulsions Containing Multilayered Membranes, J. Agric. Food Chem., 2010, 58(13), 8093–8099 CrossRef CAS.
- D. Guzey and D. J. McClements, Formation, stability and properties of multilayer emulsions for application in the food industry, Adv. Colloid Interface Sci., 2006, 128, 227–248 CrossRef.
- M. S. Katsuda, D. J. McClements, L. H. S. Miglioranza and E. A. Decker, Physical and oxidative stability of fish oil-in-water emulsions stabilized with beta-lactoglobulin and pectin, J. Agric. Food Chem., 2008, 56(14), 5926–5931 CrossRef CAS.
- T. Tokle, U. Lesmes and D. J. McClements, Impact of Electrostatic Deposition of Anionic Polysaccharides on the Stability of Oil Droplets Coated by Lactoferrin, J. Agric. Food Chem., 2010, 58(17), 9825–9832 CrossRef CAS.
- J. M. Ames, Evidence against dietary advanced glycation endproducts being a risk to human health, Mol. Nutr. Food Res., 2007, 51(9), 1085–1090 CAS.
- C. M. Oliver, L. D. Melton and R. A. Stanley, Creating proteins with novel functionality via the Maillard reaction: A review, Crit. Rev. Food Sci. Nutr., 2006, 46(4), 337–350 CrossRef CAS.
- M. A. Augustin, L. Sanguansri and O. Bode, Maillard reaction products as encapsulants for fish oil powders, J. Food Sci., 2006, 71(2), E25–E32 CrossRef CAS.
- S. Drusch, S. Berg, M. Scampicchio, Y. Serfert, V. Somoza, S. Mannino and K. Schwarz, Role of glycated caseinate in stabilisation of microencapsulated
lipophilic functional ingredients, Food Hydrocolloids, 2009, 23(3), 942–948 CrossRef CAS.
- U. Lesmes and D. J. McClements, Controlling lipid digestibility: Response of lipid droplets coated by [beta]-lactoglobulin-dextran Maillard conjugates to simulated gastrointestinal conditions, Food Hydrocolloids, 2012, 26(1), 221–230 CrossRef CAS.
- J. O'Regan and D. M. Mulvihill, Preparation, characterisation and selected functional properties of sodium caseinate-maltodextrin conjugates, Food Chem., 2009, 115(4), 1257–1267 CrossRef CAS.
- U. R. Pothakamury and G. V. BarbosaCanovas, Fundamental aspects of controlled release in foods, Trends Food Sci. Technol., 1995, 6(12), 397–406 CrossRef CAS.
- V. Crupi, R. Ficarra, M. Guardo, D. Majolino, R. Stancanelli and V. Venuti, UV-vis and FTIR-ATR spectroscopic techniques to study the inclusion complexes of genistein with beta-cyclodextrins, J. Pharm. Biomed. Anal., 2007, 44(1), 110–117 CrossRef CAS.
- V. Crupi, D. Majolino, A. Paciaroni, B. Rossi, R. Stancanelli, V. Venuti and G. Viliani, The effect of hydrogen bond on the vibrational dynamics of genistein free and complexed with beta-cyclodextrins, J. Raman Spectrosc., 2010, 41(7), 764–770 CAS.
- C. M. Oliver, A. Kher, D. McNaughton and M. A. Augustin, Use of FTIR and mass spectrometry for characterization of glycated caseins, J. Dairy Res., 2009, 76(1), 105–110 CrossRef CAS.
- E. Shimoni, Using AFM to explore food nanostructure, Curr. Opin. Colloid Interface Sci., 2008, 13(5), 368–374 CrossRef CAS.
- D. G. Dalgleish and F. R. Hallett, Dynamic Light-Scattering-Applications to Food Systems, Food Res. Int., 1995, 28(3), 181–193 CrossRef.
- A. Matalanis, U. Lesmes, E. A. Decker and D. J. McClements, Fabrication and characterization of filled hydrogel particles based on sequential segregative and aggregative biopolymer phase separation, Food Hydrocolloids, 2010, 24(8), 689–701 CrossRef CAS.
- G. A. van Aken, M. H. Vingerhoeds and R. A. de Wijk, Textural perception of liquid emulsions: Role of oil content, oil viscosity and emulsion viscosity, Food Hydrocolloids, 2011, 25(4), 789–796 CrossRef CAS.
- Y. H. Cho and D. J. McClements, Theoretical Stability Maps for Guiding Preparation of Emulsions Stabilized by Protein-Polysaccharide Interfacial Complexes, Langmuir, 2009, 25(12), 6649–6657 CrossRef CAS.
- M. D. Normand, U. Lesmes, M. G. Corradini and M. Peleg, Wolfram Demonstrations: Free Interactive Software for Food Engineering Education and Practice, Food Eng. Rev., 2(3), 157–167 CrossRef.
- J. M. Cevallos-Cevallos, J. I. Reyes-De-Corcuera, E. Etxeberria, M. D. Danyluk and G. E. Rodrick, Metabolomic analysis in food science: a review, Trends Food Sci. Technol., 2009, 20(11–12), 557–566 CrossRef CAS.
- D. S. Wishart, Metabolomics: applications to food science and nutrition research, Trends Food Sci. Technol., 2008, 19(9), 482–493 CrossRef CAS.
- F. Buyukozturk, J. C. Benneyan and R. L. Carrier, Impact of emulsion-based drug delivery systems on intestinal permeability and drug release kinetics, J. Controlled Release, 2010, 142(1), 22–30 CrossRef CAS.
- H. L. Mu, Bioavailability of omega-3 long-chain polyunsaturated fatty acids from foods, Agro Food Ind. Hi-Tech, 2008, 19(4), 24–26 CAS.
- J. Parada and J. M. Aguilera, Food microstructure affects the bioavailability of several nutrients, J. Food Sci., 2007, 72(2), R21–R32 CrossRef CAS.
- K. J. H. Wienk, J. J. M. Marx and A. C. Beynen, The concept of iron bioavailability and its assessment, Eur. J. Nutr., 1999, 38(2), 51–75 CrossRef CAS.
- B. Kuo, R. W. McCallum, K. L. Koch, M. D. Sitrin, J. M. Wo, W. D. Chey, W. L. Hasler, J. M. Lackner, L. A. Katzt, J. R. Semler, G. E. Wilding and H. P. Parkman, Comparison of gastric emptying of a nondigestible capsule to a radio-labelled meal in healthy and gastroparetic subjects, Aliment. Pharmacol. Ther., 2008, 27(2), 186–196 CrossRef CAS.
- I. Sarosiek, K. H. Selover, L. A. Katz, J. R. Semler, G. E. Wilding, J. M. Lackner, M. D. Sitrin, B. Kuo, W. D. Chey, W. L. Hasler, K. L. Koch, H. P. Parkman, J. Sarosiek and R. W. McCallum, The assessment of regional gut transit times in healthy controls and patients with gastroparesis using wireless motility technology, Aliment. Pharmacol. Ther., 2010, 31(2), 313–322 CAS.
- S. S. C. Rao, B. Kuo, R. W. McCallum, W. D. Chey, J. K. Dibaise, W. L. Hasler, K. L. Koch, J. M. Lackner, C. Miller, R. Saad, J. R. Semler, M. D. Sitrin, G. E. Wilding and H. P. Parkman, Investigation of Colonic and Whole-Gut Transit With Wireless Motility Capsule and Radiopaque Markers in Constipation, Clin. Gastroenterol. Hepatol., 2009, 7(5), 537–544 CrossRef.
- P. J. Lu, P. I. Hsu, C. H. Chen, M. Hsiao, W. C. Chang, H. H. Tseng, K. H. Lin, S. K. Chuah and H. C. Chen, Gastric juice acidity in upper gastrointestinal diseases, World J. Gastroenterol., 16(43), 5496–5501 CrossRef.
-
A. J. Vender, J. Sherman and D. S. Lucaino, Human Physiology, WCB MacGraw-Hill, 7th edn, 1998 Search PubMed.
- A. M. O'Hara and F. Shanahan, The gut flora as a forgotten organ, EMBO Rep., 2006, 7(7), 688–693 CrossRef CAS.
- F. Guarner and J. R. Malagelada, Gut flora in health and disease, Lancet, 2003, 361(9356), 512–519 CrossRef.
- G. Boudry, E. S. David, V. Douard, I. M. Monteiro, I. Le Huerou-Luron and R. P. Ferraris, Role of Intestinal Transporters in Neonatal Nutrition: Carbohydrates, Proteins, Lipids, Minerals, and Vitamins, J. Pediatr. Gastroenterol. Nutr., 51(4), 380–401 CrossRef CAS.
- K. E. Scholz-Ahrens, P. Ade, B. Marten, P. Weber, W. Timm, Y. Asil, C. C. Gluer and J. Schrezenmeir, Prebiotics, probiotics, and synbiotics affect mineral absorption, bone mineral content, and bone structure, J. Nutr., 2007, 137(3), 838S–846S CAS.
- H. M. Said, Intestinal absorption of water-soluble vitamins in health and disease, Biochem. J., 437(3), 357–72 CrossRef CAS.
- J. S. Cohn, A. Kamili, E. Wat, R. W. S. Chung and S. Tandy, Reduction in intestinal cholesterol absorption by various food components: Mechanisms and implications, Atheroscler. Suppl., 11(1), 45–48 CrossRef CAS.
-
L. R. Johnson, Gastrointestinal Physiology, Mosby Elsevier, Philadelphia, PA, 7th edn, 2007 Search PubMed.
-
G. J. Tortora, B. Derrickson, Principles of Anatomy and Physiology, Wiley and Sons Inc., Hoboken, 13th edn, 2011, Vol. 2 Search PubMed.
- K. K. Reed and R. Wickham, Review of the Gastrointestinal Tract: From Macro to Micro, Semin. Oncol. Nurs., 2009, 25(1), 3–14 CrossRef.
- L. Schenkels, E. C. I. Veerman and A. V. N. Amerongen, Biochemical-Composition of Human Saliva in Relation to Other Mucosal Fluids, Crit. Rev. Oral Biol. Med., 1995, 6(2), 161–175 CAS.
-
J. Dipalma; C. L. Kirk; M. Hamosh; A. R. Colon; S. B. Benjamin; P. Hamosh, Lipase and Pepsin Activity in the Gastric-Mucosa of Infants, Children, and Adults. Gastroenterology 1991, 101, (1), pp. 116–121 Search PubMed.
- A. Allen and G. Flemstrom, Gastroduodenal mucus bicarbonate barrier: protection against acid and pepsin, Am. J. Physiol., 2005, 288(1), C1–C19 CAS.
- J.
D. Kaunitz, Barrier function of gastric mucus, Keio J. Med., 1999, 48(2), 63–68 CrossRef CAS.
- J. M. DeSesso and C. F. Jacobson, Anatomical and physiological parameters affecting gastrointestinal absorption in humans and rats, Food Chem. Toxicol., 2001, 39(3), 209–228 CrossRef CAS.
- T. T. Kararli, Comparison of the Gastrointestinal Anatomy, Physiology, and Biochemistry of Humans and Commonly Used Laboratory-Animals, Biopharm. Drug Dispos., 1995, 16(5), 351–380 CrossRef CAS.
- M. Martinez, G. Amidon, L. Clarke, W. W. Jones, A. Mitra and J. Riviere, Applying the biopharmaceutics classification system to veterinary pharmaceutical products Part II. Physiological considerations, Adv. Drug Delivery Rev., 2002, 54(6), 825–850 CrossRef CAS.
-
E. Acosta, Delivery and controlled release of bioactives in foods and nutraceuticals, Woodhead Publishing Limited: CRC Press, 2008 Search PubMed.
- S. J. Hur, B. O. Lim, E. A. Decker and D. J. McClements,
In vitro human digestion models for food applications, Food Chem., 2011, 125(1), 1–12 CrossRef CAS.
- F. B. Kong and R. P. Singh, A Human Gastric Simulator (HGS) to Study Food Digestion in Human Stomach, J. Food Sci., 2010, 75(9), E627–E635 CrossRef CAS.
- A. Mercuri, A. Lo Curto, M. S. J. Wickham, D. Q. M. Craig and S. A. Barker, Dynamic gastric model (DGM): a novel in vitro apparatus to assess the impact of gastric digestion on the droplet size of self-emulsifying drug-delivery systems, J. Pharm. Pharmacol., 2008, 60, 4 CrossRef.
- J. Y. Yoo and X. D. Chen, GIT Physicochemical Modeling-A Critical Review, Int. J. Food Eng., 2006, 2(4), 4 Search PubMed.
- K. Molly, M. V. Woestyne and W. Verstraete, Development of A 5-Step Multichamber Reactor as a Simulation of the Human Intestinal Microbial Ecosystem, Appl. Microbiol. Biotechnol., 1993, 39(2), 254–258 CrossRef CAS.
- M. Minekus, P. Marteau, R. Havenaar and J. H. J. Huisintveld, A Multicompartmental Dynamic Computer-Controlled Model Simulating the Stomach and Small-Intestine, ATLA, Altern. Lab. Anim., 1995, 23(2), 197–209 Search PubMed.
- G. T. Macfarlane, S. Macfarlane and G. R. Gibson, Validation of a Three-Stage Compound Continuous Culture System for Investigating the Effect of Retention Time on the Ecology and Metabolism of Bacteria in the Human Colon, Microb. Ecol., 1998, 35(2), 180–187 CrossRef CAS.
- T. Mattila-Sandholm, J. Matta and M. Saarela, Lactic acid bacteria with health claims-interactions and interference with gastrointestinal flora, Int. Dairy J., 1999, 9(1), 25–35 CrossRef.
- Y. Zhang, K. Gilbertson and W. H. Finlay,
In vivo-in vitro comparison of deposition in three mouth-throat models with Qvar (R) and Turbuhaler (R) inhalers, J. Aerosol Med., 2007, 20(3), 227–235 CrossRef CAS.
- Y. Zhou, J. Sun and Y.-S. Cheng, Comparison of Deposition in the USP and Physical Mouth-Throat Models with Solid and Liquid Particles, J. Aerosol Med. Pulm. Drug Delivery, 2011, 24, 1 CrossRef.
- T. Mills, F. Spyropoulos, I. T. Norton and S. Bakalis, Development of an in vitro mouth model to quantify salt release from gels, Food Hydrocolloids, 2011, 25(1), 107–113 CrossRef CAS.
- A. Sarkar, K. K. T. Goh, R. P. Singh and H. Singh, Behaviour of an oil-in-water emulsion stabilized by beta-lactoglobulin in an in vitro gastric model, Food Hydrocolloids, 2009, 23(6), 1563–1569 CrossRef CAS.
- F. B. Kong and R. P. Singh, Solid Loss of Carrots During Simulated Gastric Digestion, Food Biophys., 2010, 6(1), 84–93 CrossRef.
- M. J. Ferrua and R. P. Singh, Modeling the Fluid Dynamics in a Human Stomach to Gain Insight of Food Digestion, J. Food Sci., 2010, 75(7), R151–R162 CrossRef CAS.
- H. Kozu, I. Kobayashi, M. Nakajima, K. Uemura, S. Sato and S. Ichikawa, Analysis of Flow Phenomena in Gastric Contents Induced by Human Gastric Peristalsis Using CFD, Food Biophys., 2010, 5(4), 330–336 CrossRef.
- A. Tharakan, I. T. Norton, P. J. Fryer and S. Bakalis, Mass Transfer and Nutrient Absorption in a Simulated Model of Small Intestine, J. Food Sci., 2010, 75(6), E339–E346 CrossRef CAS.
- D. Dupont, G. Mandalari, D. Molle, J. Jardin, J. Leonil, R. M. Faulks, M. S. J. Wickham, E. N. C. Mills and A. R. Mackie, Comparative resistance of food proteins to adult and infant in vitro digestion models, Mol. Nutr. Food Res., 2010, 54(6), 767–780 CAS.
- A. Macierzanka, A. I. Sancho, E. N. C. Mills, N. M. Rigby and A. R. Mackie, Emulsification alters simulated gastrointestinal proteolysis of beta-casein and beta-lactoglobulin, Soft Matter, 2009, 5(3), 538–550 RSC.
- A. Sarkar, K. K. T. Goh and H. Singh, Colloidal stability and interactions of milk-protein-stabilized emulsions in an artificial saliva, Food Hydrocolloids, 2009, 23(5), 1270–1278 CrossRef CAS.
- A. Sarkar, K. K. T. Goh and H. Singh, Properties of oil-in-water emulsions stabilized by beta-lactoglobulin in simulated gastric fluid as influenced by ionic strength and presence of mucin, Food Hydrocolloids, 2010, 24(5), 534–541 CrossRef CAS.
Footnote |
† Equal contribution |
|
This journal is © The Royal Society of Chemistry 2012 |