Manganese in the upper Severn mid-Wales
Received
9th August 2011
, Accepted 21st November 2011
First published on 30th November 2011
Abstract
The concentrations of manganese (Mn) in the Upper River Severn (the Plynlimon catchments) are examined in relation to rainfall, cloud water, throughfall, stemflow and stream water concentrations where there is over 20 years of monitoring data available. Manganese concentrations are particularly low in rainfall and cloud water, with maximum concentrations occurring under low volumes of catch due to atmospheric “washout” of contaminants and dry deposition. There is strong Mn enrichment in throughfall and stemflow and this is probably linked to cycling through the vegetation. Manganese in the streams and groundwaters are primarily supplied from within-catchment sources. The highest concentrations occur within the tree canopy probably due to element cycling and in groundwaters due to mobilisation from the rock. Manganese concentrations in streams are at their lowest during spring and summer following long dry spells, with rapid increases following subsequent rain. There is no clear long-term trend in Mn concentration in the streams although there are increases in Mn concentrations for years when there is extensive felling of spruce plantation forest and in 1995 following a more extensive dry period. New high resolution monitoring picks up the effects of the rising limb of the hydrograph when concentrations rapidly increase, diurnal patterns during summer low-flow periods and contrasting dynamics between moorland and forested catchments.
Environmental impact
Manganese release from catchments can pose water quality issues for river systems especially within acidic and acid sensitive upland areas or from disturbance from forestry clearance. During a precipitation event, Mn is displaced from the soils into the streams and then subsequent events have a more limited impact on stream concentrations. In contrast in drought periods when the stream is at base flow concentrations decline and diurnal cycling is observed. Long-term and high resolution monitoring shows features influenced by a combination of changes driven by factors such as climatic variation and land use change with complex within-catchment, hyperheic-river and within-river processing that requires further evaluation.
|
1. Introduction
The UK uplands are of strategic environmental importance, with the headwaters of many major river systems providing a major source of potable, industrial and agricultural water supplies and often constitute areas of outstanding natural beauty with high amenity/ecological status. Despite this, they have been and continue to be susceptible to the deposition of acidic oxides from industrial emissions1 and there is also an issue of forestry rotation cycles following the introduction of conifer plantations onto acidic and acid sensitive moorland during the first half of the twentieth century.2,3
Although the main focus on UK upland water quality has been on those components directly linked to acidification and forestry rotation cycles, such as pH, inorganic aluminium and strong acid anions (chloride, sulphate and nitrate), there are other issues of importance. In this paper we deal with manganese (Mn). Concentrations can be relatively high in UK upland rivers and the levels encountered can be of both ecological and water potability significance.4–8 Concentrations exceeding the guideline value for public supplies of 50 μg L−1,9 for example arising from soil disturbance and erosion from forest operations,10 may affect the taste and reduce the water pressure and flow in the supply pipes.11
Manganese is the twelfth most abundant element and, after iron and titanium, the third most abundant transition element in the earth's crust averaging 0.106%.12 Despite this, Mn concentrations are moderately low in surface waters (typically less than 1 mg L−1) with the ratio of dissolved concentration river water and seawater to the crustal average being around two orders of magnitude lower than ions such as sodium and chloride but two orders of magnitude higher than easily hydrolysable trivalent metals such as iron and aluminium.13Manganese has a wide range of oxidation states (−3 to +7) but in nature it primarily occurs in solution and in mineral forms in oxidation states of Mn II and Mn IV, the latter having such a high surface charge density that in this form Mn predominates as low solubility oxides and oxy-hydroxides. Under reducing conditions, Mn II is mobilised but it is lost from the water column in well-oxygenated surface environment where oxidation occurs leading to a much lower solubility.
In this paper, Mn concentrations are described for a key experimental research area in the UK uplands (the Plynlimon catchments of mid-Wales) where there have been extensive water quality monitoring of rainfall, mist, stream and ground waters over 27 years.14,15 The paper also presents the results of a new high resolution monitoring programme that indicates remarkable dynamics for Mn concentrations in streams draining moorland and harvested forest. The aims of the study are to examine the biogeochemical cycling of Mn, to describe the processes that occur during storm events and the diurnal changes in stream concentrations during low flow periods in the summer. Within the study new data on the short-term dynamics are brought to the fore based on a new high resolution study of rainfall and runoff within one of the main tributaries of the upper Severn, the Afon Hafren.16 The hypothesis tested is that long-term and high intensity monitoring of Mn reveals new features that add to or challenges our understanding of hydrogeochemical functioning.17
2. Experimental
Study area
The study area comprises the headwaters of the River Severn (catchment c. 8 km2).18 Here (Fig. 1), the Upper River Severn drains a hill top plateau region dominated by acid moorland and the Hafren Forest in the lower half of the catchment. The Upper Severn comprises two main tributaries, the Afon Hafren and the Afon Hore, together with the Nant Tanllwyth that joins the Afon Hafren near to its confluence with the Afon Hore. The soils in the area are a mixture of upland acid soil types dominated by peaty podzol with some peaty gley and deep peat in the moorland plateau area. The bedrock is fractured Lower Palaeozoic mudstones, shales and grits. The Hafren Forest mainly comprises of Sitka spruce with some Norway spruce, larch and lodgepole pine, planted in various phases from the mid 1940s through to the late 1960s. Both the lower parts of the Hafren and the Hore are within the Hafren forest, while the Tanllwyth catchment is fully within the forest. However, during the study there has been thinning of the forest in places and complete deforestation with replanting in others (details are provided in Table 1). The water quality of the Upper Severn is variable, with baseflow waters of good quality (calcium bicarbonate type) and more acidic and aluminium bearing water of much poorer quality occurring under stormflow conditions. The general water quality functioning of the area is provided by Neal et al.,14,19 the groundwater chemistry15 and broader aspects are covered in a special issue of Hydrology and Earth System Sciences.20 With regards to rainfall and stream flows, summary information is provided elsewhere21 as is information on groundwater levels.15 Details of the high resolution water quality study are covered elsewhere.16
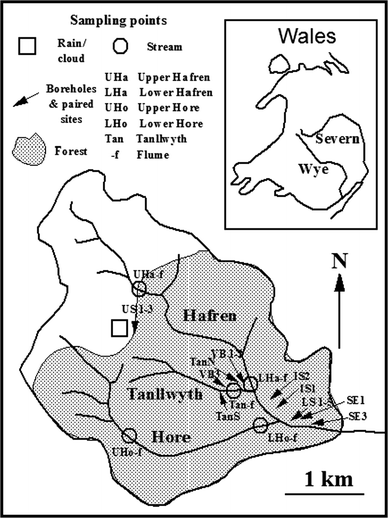 |
| Fig. 1 Map showing the locations of the streams and groundwater sampling points at Plynlimon. | |
Table 1 Catchment summary information. The HA4 and LS4 boreholes represent the same site: LS data correspond to a monthly sampling for one year only and the HA4 data include weekly sampling after the first year of sampling. The paired sites have control and felled catchments, the site names have suffix “c” for the control and suffix “f” for felled sites. SS = Sitka Spruce; M = Acid Grassland. Soil type G = Gley; P = Podzol; Pe = Peat, Gr = Gravel. Under felling activity Y = Yes, 100% fell unless indicated otherwise; N = No fell. The superscript denote felling date: 1, LHa, ongoing thinning; 2, UHo clearfell 2006; 3, LHo, March 1985 to October 1988; 4, Tan, February 1996; 5, S2Ho, August-October 1989; 6, SE1, September-October 1995
Site |
Area (Ha) |
Soil Type |
Veg. Type |
Felling Activity |
Sampling Times/yr |
Start date |
End date |
Atmospheric inputs |
Rain |
— |
— |
— |
— |
52 |
10/05/83 |
Cont |
Rain |
— |
— |
— |
— |
52 |
08/03/07 |
21/01/09 |
Mist |
— |
— |
— |
— |
52 |
25/9/90 |
Cont |
Throughfall |
— |
— |
— |
— |
52 |
01/02/84 |
02/09/92 |
Stemflow |
— |
— |
— |
— |
52 |
01/02/84 |
02/09/92 |
Main streams |
U Hafren |
117 |
Pe
|
M |
N |
52 |
17/07/90 |
Cont |
U Hafren |
117 |
Pe
|
M |
N |
1248 |
08/03/07 |
21/01/09 |
L Hafren |
347 |
P/G |
SS |
Y < 25%1 |
52 |
10/05/83 |
Cont |
L Hafren |
347 |
P/G |
SS |
Y < 25%1 |
1248 |
08/03/07 |
11/03/08 |
U Hore |
178 |
P/G |
SS |
Y50%2 |
|
28/08/84 |
Cont |
L Hore |
335 |
P/G |
SS |
Y50%3 |
52 |
10/05/83 |
Cont |
Intermediate size stream |
Tanllwyth |
51 |
G |
SS |
Y50%4 |
52 |
17/09/91 |
Cont |
Small streams |
South2Hore |
3–6 |
P |
SS |
Y100%5 |
52 |
19/04/88 |
20/02/01 |
SE1f
|
2–4 |
P |
SS |
Y100%6 |
26 |
20/09/94 |
14/02/01 |
SE3c
|
2–4 |
P |
SS |
N |
26 |
11/10/94 |
27/04/99 |
TanN |
<2 |
G |
SS |
N |
26 |
28/04/94 |
27/04/99 |
TanS |
<2 |
G |
SS |
Y100%4 |
26 |
28/04/94 |
14/02/01 |
Groundwater |
HA4
|
|
P |
SS |
Y100%6 |
12/52 |
24/04/94 |
14/02/01 |
SE1
|
|
P |
SS |
Y100%6 |
26 |
10/05/95 |
27/04/99 |
SE3
|
|
P |
SS |
N |
26 |
10/05/95 |
27/04/99 |
TanN |
|
G |
SS |
N |
26 |
05/07/94 |
27/04/99 |
TanS |
|
G |
SS |
Y100%4 |
26 |
09/08/94 |
27/04/99 |
US1
|
|
Pe
|
M |
M |
12 |
24/04/94 |
12/07/95 |
US2
|
|
P |
SS |
N |
12 |
24/04/94 |
12/07/95 |
US3
|
|
P |
SS |
N |
12 |
24/04/94 |
14/06/95 |
US4
|
|
P |
SS |
N |
12 |
24/04/94 |
12/07/95 |
LS1
|
|
P |
SS |
N |
12 |
24/04/94 |
12/07/95 |
LS2
|
|
P |
SS |
N |
12 |
24/04/94 |
12/07/95 |
LS3
|
|
P |
SS |
N |
12 |
24/04/94 |
12/07/95 |
LS4
|
|
P |
SS |
N |
12 |
24/04/94 |
12/07/95 |
IS1
|
|
P |
SS |
N |
12 |
24/04/94 |
12/07/95 |
IS2
|
|
P |
SS |
N |
12 |
24/04/94 |
12/07/95 |
VB1
|
|
P/Gr |
M |
M |
12 |
24/04/94 |
12/07/95 |
Sample collection and chemical analysis
For this study, information is drawn from monitoring programmes of rainfall, cloud water, throughfall, stemflow, stream water and groundwater covering time frames from one to almost thirty years (Table 1). Samples were mainly collected on a weekly basis14 and additionally a 7 hourly monitoring programme in the case of rainfall and the Upper and Lower Hafren for 2007–2008.16 Briefly, the samples of rainfall (bulk deposition) and cloud water (occult deposition) were collected using open and passive lidded “harp type” collectors respectively at the Carreg Wen meteorological site (52° 29′ N, 3° 43′ W) approximately 0.5 km to the south of the Upper Hafren stream flow gauging structure at an altitude of 580 m.22 The throughfall was collected using 4 trough collectors while stemflow was collected from 4 trees using diagonally slanted tubing fixed to the bark that transferred water to a collector at the base of each tree.23 The samples for rainfall, cloud water, throughfall and stemflow were time integrated ones, while for the streams and groundwaters, instantaneous grab samples were collected. At each of the stream sampling sites, an instantaneous sample of stream water was collected every seven hours using Xian 1000 portable automatic samplers, each fitted with a carousel of twenty four 500 ml high density polythene bottles and housed in enclosures on the stream bank.16 The samplers operate on a pressure/vacuum principle drawing water into a silicone coated glass bottle before dispensing it into a 500 ml sample bottle. The waters were filtered within 24 h on return to the laboratories for rainfall, cloud water, throughfall and stemflow, while the stream and groundwater samples were filtered in the field. Filtration was undertaken under vacuum using 0.45 μm cellulose acetate circles apart from the 7 hourly monitoring samples when pressure filtration was used. In all cases, the filtration equipment was thoroughly cleaned before use and the filters/filtration equipment bottles were washed with several aliquots of sample before the sample for analysis was collected. All the sample bottles were pre-washed with 10% HCl (v/v) acid-washed polyethylene bottles.
The samples were stored, at 4 °C in the dark, in acid washed polypropylene bottles, acidified to 1% v/v with high purity concentrated nitric acid within one day of sampling to avoid sample deterioration. Mn concentrations were determined using inductively coupled plasma emission spectrometry (Perkin Elmer DV 3400 ICP-OES)14 up to 1997, and by inductively coupled plasma mass spectrometry (Perkin Elmer DRC II ICP-MS) from 1997 onwards. All analysis from the samples collected from 1997 onwards was conducted according to ISO 17025 and accredited by the United Kingdom Accreditation Service. Calibration standards were cross-checked against certified reference material; for example the National Research Council Canada River Water SLRS-4 Mn bias was 2% in May 2007. To minimise the likelihood of bias between laboratories, both laboratories participated in an external proficiency testing scheme (Aquacheck); for example the average bias of 5 PT samples in 2007 and 2008 was −5% with a range between −2 and −7%. Analysis involved three replicate measurements and approximately 10% of each analytical batch contained internal quality control samples as a means of validating each measurement. For the ICP-OES, check standards were used to correct for instrument drift, and if the standards run as samples differed from the true values by more than 5% then the analysis was repeated. For ICP-MS, an internal standard of gallium was used to correct each sample for instrumental drift. The lowest quotable value for Mn was 0.2 μg L−1 for ICP-OES and 0.02 μg L−1 for ICP-MS (calculated on 4*s n = 10 blank determinations). However, data without the detection limit filter applied is used in the statistical analysis for this paper.
3. Results and discussion
The results from the weekly samplings collected over the last 30 years are summarized in Table 2 together with ancillary information for catchments nearby (Table 3) while Mn concentration time series are shown in Fig. 2 for the main long-term term monitoring sites on the Hafren, Hore and Tanllwyth.
Table 2 Summary statistics for manganese concentrations (μg L−1) in rainfall, cloud water, stream water and groundwater for the Upper Severn catchment. The low and high refer to dry and wet conditions with the average for the bottom and top 10% of flows/volume of catch/groundwater level
|
Average |
Fw Avg |
Median |
std |
min |
max |
N |
Low |
High |
Atmospheric inputs |
Rainfall |
2 |
1 |
1 |
5 |
0 |
99 |
1023 |
8 |
0 |
Cloud |
26 |
32 |
8 |
82 |
0 |
1130 |
831 |
51 |
5 |
Throughfall |
723 |
1250 |
571 |
862 |
61 |
9160 |
141 |
1566 |
652 |
Stemflow |
1414 |
681 |
949 |
1640 |
72 |
10900 |
133 |
1839 |
1005 |
Main streams |
U Hafren |
20 |
20 |
20 |
5 |
0 |
43 |
1000 |
11 |
18 |
L Hafren |
34 |
40 |
34 |
13 |
5 |
128 |
1434 |
22 |
45 |
U Hore |
24 |
22 |
23 |
7 |
6 |
90 |
1324 |
17 |
21 |
L Hore |
31 |
33 |
30 |
9 |
6 |
87 |
1434 |
21 |
35 |
Intermediate stream |
Tanllwyth |
89 |
75 |
87 |
23 |
22 |
167 |
735 |
107 |
60 |
Small streams |
South 2 Hore |
70 |
55 |
68 |
22 |
10 |
153 |
638 |
61 |
44 |
SE1
|
8 |
14 |
5 |
9 |
1 |
67 |
173 |
4 |
19 |
SE3
|
43 |
85 |
25 |
40 |
3 |
195 |
124 |
14 |
121 |
TanN |
16 |
11 |
13 |
12 |
1 |
79 |
106 |
37 |
11 |
TanS |
12 |
9 |
11 |
8 |
2 |
68 |
152 |
11 |
8 |
Groundwater |
US1
|
67 |
na
|
62 |
20 |
20 |
103 |
16 |
na
|
na
|
US2
|
77 |
na
|
48 |
54 |
33 |
233 |
15 |
na
|
na
|
US3
|
47 |
na
|
41 |
16 |
16 |
100 |
16 |
na
|
na
|
LS1
|
53 |
na
|
51 |
9 |
9 |
74 |
16 |
na
|
na
|
LS2
|
139 |
na
|
129 |
66 |
40 |
261 |
15 |
na
|
na
|
LS3
|
76 |
na
|
27 |
146 |
21 |
663 |
16 |
na
|
na
|
LS4
|
61 |
na
|
41 |
53 |
27 |
325 |
206 |
118 |
40 |
IS1
|
45 |
na
|
23 |
79 |
16 |
367 |
16 |
na
|
na
|
IS1
|
87 |
na
|
47 |
91 |
16 |
367 |
32 |
na
|
na
|
SE1
|
153 |
na
|
149 |
58 |
28 |
362 |
102 |
168 |
160 |
SE3
|
131 |
na
|
132 |
46 |
8 |
230 |
102 |
82 |
150 |
TanN |
165 |
na
|
166 |
47 |
47 |
533 |
132 |
410 |
397 |
TanS |
251 |
na
|
217 |
115 |
67 |
460 |
136 |
218 |
157 |
Quarry |
8 |
na
|
2 |
44 |
0 |
505 |
131 |
3 |
10 |
VB1
|
332 |
na
|
201 |
234 |
133 |
771 |
16 |
na
|
na
|
Table 3 Summary statistics for manganese concentrations (μg L−1) in rainfall, stream water and groundwater for the Upper Wye and Vyrnwy areas. The low and high refer to dry and wet conditions with the average for the bottom and top 10% of flows/volume of catch/groundwater level
|
Avg |
Fw Avg |
Median |
std |
min |
max |
N |
Low |
High |
Upper Wye |
Afon Gwy |
17 |
16 |
17 |
6 |
0 |
54 |
83 |
15 |
16 |
Afon Cyff |
24 |
20 |
22 |
12 |
0 |
93 |
83 |
36 |
20 |
Nant Iago |
33 |
na
|
34 |
8 |
8 |
45 |
20 |
na
|
na
|
Vyrnwy |
Rainfall |
7 |
na
|
12 |
12 |
0 |
97 |
126 |
na
|
na
|
Stream 1 |
10 |
na
|
6 |
11 |
0 |
74 |
151 |
na
|
na
|
Stream 2 |
7 |
na
|
3 |
17 |
0 |
180 |
160 |
na
|
na
|
Borehole 1 |
18 |
na
|
5 |
48 |
1 |
281 |
162 |
na
|
na
|
Borehole 2 |
3 |
na
|
2 |
10 |
0 |
90 |
163 |
na
|
na
|
 |
| Fig. 2 Time series plots of Mn for the monitored streams in the Upper Severn based on weekly monitoring. Linear regression lines are included and the regression statistics are provided in Table 7. | |
3.1 Atmospheric inputs and leaching from forests
Manganese concentrations are highly variable with lowest average concentrations occurring in rainfall (average, median and standard deviation 2, 1 and 5 μg L−1, respectively). The variations in Mn concentrations across the Upper Severn fit well with previous studies. For example, Mn concentrations in the rainfall and cloud water are relatively low and the highest concentrations occur at low volumes of catch due to atmospheric scavenging processes and there can be a significant deposition flux related to the cloud water input.22 For the Upper sites streams in the moorland, the contributions to the streams are low and arise from the precipitation and surface soil leaching. Highest average and median concentrations occur in throughfall and stemflow (723/571 and 1414/949 μg L−1, respectively), arising from both the wet and occult deposition, resulting in enhanced Mn deposition in the forested catchments of the Lower Hafren and Lower Hore. Within the throughfall and stemflow there are high concentrations of Mn and this fits well with previous observations where there can be substantial leaching from the foliage.24–26
3.2 Streams (main, intermediate and small)
For the main streams, Mn concentrations are similar in terms of average, median and range (Table 2). Further, unlike rainfall, the average values are similar to the median and for the remainder of this section, only average values are provided to avoid duplication. For example, for the Upper and Lower Hafren and Hore, the average Mn concentrations range between 20 and 35 μg L−1 with Mn concentrations higher at the lower sites attributable to the contribution of the soil water and canopy leachates from the conifer plantations. For the ‘intermediate stream’ of the Tanllwyth, the average Mn concentration (89 μg L−1) is around three times higher than the other streams and similar to arsenic.27 The small streams show a variable range in average (8 to 70 μg L−1) although there is no clear separation between the drainage for the podzol and gley soils. We suspect that there is an influence of soil type but we may not be comparing similar water types (see below) and in general averages/medians are similar while standard deviations are fairly high. For the streams, mean and maximum Mn concentrations are generally lower in headwater streams that rise in the Drosgol and Brynglas Formations (Upper Hafren, Upper Hore) compared to the other headwater streams. As previously noted, Mn mobilisation seems linked to soil horizons with a mixture of organic and inorganic materials.6 However the data for the small streams, which are notionally all on the same mudstone bedrock (Glaslyn Formation), show that these broad relationships are modified and confounded by the influence of soil type and hydrology as well as factors such as drying/wetting cycles.6 Even at the relatively small scale of the Upper Severn catchment (c. 8 km2) it is difficult to explain the relatively large variability in stream and groundwater Mn concentrations without detailed analysis of hydrological flow paths in relation to soil type and land cover.
However, within the main streams, the lowest Mn concentrations occur at the lowest of flows especially after extensive periods of drought. This decline at very low flows (Fig. 3) probably indicates a removal of Mn from the water column under baseflow conditions as opposed to the increased contribution of groundwater. If groundwaters dominated during baseflow conditions the stream Mn concentrations would be expected to be maximal as groundwater concentrations are much higher than stream averages (Table 2). On wetting up, Mn concentrations rapidly increase in concentrations to non-drought levels and for some streams there is a marked decline in concentration with increasing flow from moderate to high flows (Fig. 3) and probably reflects a dilution term following storm-break, when removal of Mn from the water column is small. As such the relationship between Mn and flow shows both linear and inverse relationships that are also indicated by linear regression (Table 4).
 |
| Fig. 3 The relationship between Mn concentration and flow for the Upper Severn streams. Note that there seems to divergent patterns in the plots and linear statistics indicate a mixture of flow and inverse flow relationships with Mn. The statistics are presented in Table 4. | |
Table 4 Multiple linear regression of Mnversus flow and 1/flow for the monitored streams in the Upper Severn based on weekly monitoring (see Fig. 3)
|
Flow |
2σ |
1/Flow |
2σ |
Constant |
2 σ |
r2 |
N |
p |
Upper Hafren |
−7.69 |
1.95 |
−0.1 |
0.01 |
22.91 |
7.79 |
0.274 |
998 |
<0.0001 |
Upper Hore |
−6.81 |
1.78 |
−0.17 |
0.02 |
28.39 |
12.25 |
0.177 |
1218 |
<0.0001 |
Lower Hafren |
0.73 |
1.44 |
−0.67 |
0.06 |
40.79 |
21.41 |
0.299 |
1409 |
<0.0001 |
Lower Hore |
−1.85 |
1.03 |
−0.39 |
0.03 |
36.44 |
15.39 |
0.279 |
1410 |
<0.0001 |
Tanllwyth |
−33.17 |
12.91 |
0.52 |
0.07 |
88.76 |
48.86 |
0.459 |
392 |
<0.0001 |
South2Hore |
−36.55 |
7.87 |
0.15 |
0.09 |
71.28 |
39.95 |
0.188 |
637 |
<0.0001 |
Although we focus on the upper River Severn, there is companion Mn data for the upper River Wye. For this area there are three monitoring points: the Afon Gwy (acid moorland), the Afon Cyff (agriculturally improved, limed, grassland) and the Nant Iago (moorland contaminated by a derelict lead-zinc mine with spoil waste).28Mn concentration averages are 17, 24 and 33 μg L−1 for the Gwy, Cyff and Iago, respectively. In a companion study of conifer forest on brown earth soils average Mn concentrations in runoff averaged 7 and 10 μg L−1 for two sites.29 These values clearly are similar to those for the Upper Severn.
3.3 Groundwaters and the correspondence with the streams
The groundwaters (Table 2) also exhibit a wide range in average and median Mn concentrations (8/2 to 332/217 μg L−1). As with the streams, the average and median concentrations are similar and for brevity only averages are described here. In general the Mn concentrations in groundwater are about three times higher than within the streams (averages 115 and 35 μg L−1, respectively). For Plynlimon, average groundwater concentrations exceed the national drinking water limit of 50 μg L−1. For the 16 boreholes, the majority (81%) show elevated average concentrations above the drinking water limit with maximum measured values up to thirteen times this threshold limit. Only in one of these sampling points (Quarry) was the average value (2 μg L−1) well below this limit. The concentrations in this catchment are well above the median of 13 μg L−1 for Scottish groundwaters.9 With respect to the Plynlimon streams, average concentrations for the Upper and Lower Hafren and Hore as well as the remaining small streams are below the 50 μg L−1 threshold, with only occasional exceedance.
Manganese in the streams and groundwaters are mainly derived from the catchment (typically around 90 to 95%) as shown for the Plynlimon catchments with previous mass-balance studies14,30 and the highest concentrations occur within the groundwaters where residence times will be high. Some of the broad variations in Mn concentrations amongst the streams and groundwater can probably be accounted for by variations in bedrock Mn. Concentrations of Mn in four of the bedrock units present at Plynlimon vary by an order of magnitude (Table 5) from 3200 mg kg−1 in the Silurian Devils Bridge Formation Series to 218 mg kg−1 in the Drosgol Formation of Ordovician age.31,32 Thus, groundwater in boreholes intercepting the Drosgol Formation (US1–US3) generally has lower Mn concentrations relative to those intersecting other formations. However this broad simplification is confounded by the modifying influence of catchment hydrological pathways on the chemistry of the water measured in the boreholes. The boreholes were not screened to sample water from a specific depth in the soil, drift or bedrock. Thus some of the shallower holes intercept soil through-flow whilst deeper holes sample “true” groundwater: cf. an earlier presentation on groundwaters at Plynlimon.15 There is a strong vertical gradient in soil total Mn concentrations as shown by the example soil profiles under acid grassland in the Wye catchment (Table 6).33 Thus for a given geology, lower Mn concentrations might be anticipated in those boreholes which sample soil water compared to those intercepting groundwater in the bedrock. The groundwaters have variable depth with the shallowest being for VB1, LS1, LS2, LS3 and US1 (depths averaging less than 2 m), while the deepest being for US2 and US3 (averaging around 11 m): the other sites have average depths typically around 4 to 8 m. Mn concentrations do not exhibit clear relationships with depth in terms of individual sites or averages across sites and clearly the Mn inputs to the groundwater across the area are variable and the underlying processes not easy to pin down. Nonetheless, the highest concentrations in the groundwater are associated with the gley catchments, but there also seems to be high concentrations associated with one site in gravels near to the river (VB1). The Mn mobilisation may well be linked to reducing conditions but other factors also come into play.9 Further, with reducing conditions Fe is also mobilised, but Mn is only weakly correlated with Fe. However, we have noted that in the groundwaters, green Fe(OH)2 flocks form within the groundwaters sampled within the gley and similar precipitates form within the streams at seepage points and the flocks turn brown on oxidation in the stream. As such, the linkage between Mn and Fe may be weakened by differences in oxidation and precipitation kinetics. In fact, the only strong relationship with Mn is cobalt (both in the groundwater and stream) and this may well reflect similar hydrochemical characteristics.
Table 5 Total Mn concentrations measured in bedrock at Plynlimon31,32
Rock type |
Age |
Mean (mg kg−1) |
Std dev (mg kg−1) |
N |
Devils Bridge Formation |
Silurian |
3200 |
906 |
4 |
Cwmere Group |
Silurian |
383 |
126 |
8 |
Brynglas Group |
Ordovician |
525 |
115 |
8 |
Drosgol Group |
Ordovician |
218 |
27.4 |
7 |
Table 6 Total Mn concentrations measured by DC-arc direct reading emission spectrometry in a ferric stagnopodzol soil located on the Devils Bridge Formation in the Wye catchment at Plynlimon (CEH/BGS unpublished data)33
Horizon |
Thickness (cm) |
Mn (mg kg−1) |
Oh |
10 |
60 |
Eag |
7 |
40 |
Bs1
|
30 |
270 |
Bs2
|
14 |
840 |
Bs/C |
9 |
1070 |
C |
30+ |
1500 |
3.4 The issues of Mn mobility in relation to conifer harvesting/replanting and climatic effects
Clear felling of the forest results in disturbance of the forest soils and this seems to have released Mn within the soils to be flushed to the river during periods of high flow when the catchment wets up. At Plynlimon, stem-only harvesting was practised and residual material (including stumps) was left on the catchment. The majority of the felled areas were replanted with Sitka spruce within two years of harvesting, although some high altitude ground above the source of the Hore has been left unplanted and will be allowed to revert to open moorland. The data shows an increase in Mn concentration followed by a decline within a few years. For example, for the Lower Hore where about a half of the catchment was felled over a four year period from 1985 to 1988, Mn concentrations increased from around 30 μg L−1 to around 40 μg L−1 (Fig. 2), while for small South2Hore stream, felling over a few months in 1989 led to an increase from around 100 μg L−1 to around 150 μg L−1 with decline over the next few years to levels about a 60% that of pre-fell (Fig. 2, Table 7). Clearly, the disruption of the soils at times of fell and/or the release of Mn from forest debris5 led to Mn release to the streams. Regarding long term changes in Mn concentration, linear regression indicates that there are no significant trends apart from the situation where initial felling enhanced Mn concentrations as followed by subsequent decline. Other than a felling effect, perhaps the major changes observed link to climate impacts as stream concentrations are strongly related to monthly rainfall amounts (r = 0.888, p < 0.0001) with the greatest stream concentration associated with the higher rainfall between from October to February. However, on an annual basis, the average stream concentrations are not correlated with annual rainfall amount. For the Lower Hafren average Mn concentrations appear to have declined in the last decade although the change is not uniform (Fig. 2). Indeed, care has to be taken in examining trends as there are also the impacts of felling practice to consider. For the Upper Hafren, where there has been no land disturbance as the system is simply moorland, there are no clear trends at all and it may well be that any trends must be reviewed in relation to land management and climatic factors.
Table 7 Linear regression of Mnversus time for the monitored streams in the Upper Severn based on weekly monitoring (see Fig. 2)
|
Gradient |
2σ |
Constant |
2σ |
r2 |
N |
p |
Upper Hafren |
0.23 |
0.05 |
−441.2 |
12.9 |
0.069 |
1324 |
<0.0001 |
Upper Hore |
0.01 |
0.05 |
−1.2 |
9.1 |
0.000 |
1000 |
|
Lower Hafren |
−0.49 |
0.08 |
1011.7 |
24.3 |
0.093 |
1420 |
<0.0001 |
Lower Hore |
−0.41 |
0.06 |
842.9 |
16.9 |
0.128 |
1423 |
<0.0001 |
Tanllwyth |
−1.81 |
0.31 |
3712.3 |
53.3 |
0.110 |
1127 |
<0.0001 |
South2Hore |
−3.10 |
0.40 |
6248.5 |
37.7 |
0.275 |
638 |
<0.0001 |
3.5
Mn cycling during storm events and drought periods
The 7 h data for the Upper Hafren (where there is the longest data run) shows the highly dynamic nature of Mn concentrations within the stream (Fig. 4). Indeed, following Neal et al.,16 the influence of reducing sampling frequency can be clearly observed (Fig. 4). The degree of variability is as dynamic as that for flow but over a narrower range of values. Looking over a smaller time period (Fig. 5), the 7 hourly monitoring indicates that the initial rise in Mn concentration, following drought break on day 262, occurs rapidly (within a day) and is linked to the rising limb of the hydrograph. Subsequently Mn concentrations gradually decline in line with the decrease in stream flow. It is likely that the rapid increase in Mn stream water concentrations during storm events arise from flushing the soil water from the upper soil horizons.8 Following this initial peak, the Mn concentration response to subsequent hydrograph peaks is muted until after the next dry period, indicating the importance the antecedent condition exerts on the leaching of the soil water to the stream. In general, the 7-hourly sampling interval does provide a peak associated with the storm event, although we miss the detail associated with the rising limb of the hydrograph. In terms of stream dynamics linked to flow, the greatest changes occur for the Lower Hafren and this may well link to the more rapid transit from the forest ditch systems that rapidly convey water during storm periods, which in turn may link to flushing of throughfall and stemflow. Set against this, the high dampening of rainfall signals even for relatively inert elements implies long and variable residence times.34–37 Rather, we would consider that localised inputs may be significant as for example high Mn concentrations have been observed at one groundwater site within the gravels near to the river (VB1). In contrast, the diurnal patterns are most clearly observed for the Upper Hafren (Fig. 6) and this may be linked to higher light levels associated with the moorland as opposed to the forest areas.
 |
| Fig. 4 Simulated samplings at different frequencies derived from the 7-hourly samplings. | |
 |
| Fig. 5 Time series plots of Mn concentration and flow for the Upper and Lower Hafren based on 7 hourly monitoring from 18th September 2007 to 27th October 2007. | |
 |
| Fig. 6 Diurnal cycling of manganese for the Upper and Lower Hafren for 3rd–6th May 2007 at a time of low and declining flow. | |
Further, during very low-flow periods (0.018 m3 s−1 for the Upper Hafren; 0.032 m3 s−1 for the Lower Hafren) that occur during the lower rainfall periods (March to September), diurnal oscillations can be observed for both the Upper Hafren and Lower Hafren when Mn concentrations are minimal in mid-afternoon and peak around midnight (Fig. 6). A case study is presented of a storm period and the subsequent return to baseflow conditions for the Upper Hafren stream draining from the open moorland. Following a 20-day dry period up to day 112, there was 55 mm precipitation for 2 days with a rise in the stream Mn concentration from 12 to 24 μg L−1 (Fig. 7). Without further rainfall events between days 115 and 125, the stream flow declined with diurnal cycling of Mn resuming on day 117. The initial cycle amplitude of 1 μg L−1 gradually increased to 2.7 μg L−1 on day 125 (30% of the mean Mn concentration of 9.4 μg L−1). For samples collected from the Lower Hafren site (Fig. 6) in the forest the same pattern is observed as at the moorland Upper Hafren site, with a slightly smaller amplitude of 2 μg L−1 which represents 16% of the mean Mn concentration of 12 μg L−1; i.e., it appears that the shading from the forest has reduced the amplitude of the diurnal cycle. The percentage amplitude of the cycle in the stream at Plynlimon is much smaller than at neutral-alkaline former mining area sites in Montana and Idaho which ranged from 22% up to 294% of the mean Mn concentration.38 There is no clear evidence that the cycling is related to evapo-transpiration as the stream flow declines steadily throughout the whole period when diurnal cycling is observed. However there is synchronicity of the diurnal Mn cycling with pH, calcium and nitrate; for example pH rises from a minimum in mid-afternoon of 6.39 to a maximum of 6.51 in the early hours of the morning. We note that with sample storage there may well have been some CO2 degassing and hence there may be some error in the pH measurement. However, a previous study indicated that the waters are relatively close to saturation with respect to CO2 and hence this error is probably of second order importance.39
 |
| Fig. 7 Stream Mn concentrations rise during a precipitation event on day 114 (25th April 2007) and then declines and diurnal cycling resumes. | |
These diurnal changes may well link to 1) biological processing within the stream during the summer months when biological activity will be high and flows very low; 2) light induced oxidation and precipitation/removal of MnOx from the water column, and the rate of oxidative loss of Mn is enhanced due to photosynthesis of the algae40 and increases as a function of [OH−], however our measurements indicate the loss of Mn in the daytime is associated with an increase in [H+]; 3) precipitation/adsorption onto the stream bedrock; 4) absorption by biofilms, and the increase during the dark hours is driven by Mn reduction and dissolution; 5) diurnal fluctuations in groundwaters with more groundwater input at night. At present we are unable to distinguish which is the most important driver or whether it is a combination of two or more of the above mechanisms contributing to the clear diurnal patterns in the Mn stream concentrations at observed during periods of low flow. In contrast to Mn, we observe that arsenic and molybdenum, present in the anionic forms, exhibit the highest concentrations in the diurnal cycle in the early afternoon.27
3.6 How often do you need to sample to understand the dynamics of Mn cycling in the stream?
Sampling at the high frequency of 7-hourly intervals is attractive as it has offered us the opportunity to gain a better understanding of the processes occurring during storms, and drought, and we can use this data to simulate sampling of lower frequencies (Fig. 4). While lower resolution sampling that is typical of standard monitoring programmes (fortnightly to monthly) picks up dips in Mn at extreme low flow, it is only at the weekly scale that greater dynamics start to emerge, but at 7 hourly monitoring a much greater dynamic is revealed. Note that daily sampling shows a similar feature, but of course diurnal patterns cannot be picked up.
4. Conclusions
Monitoring of Mn in the headwater streams of the Severn over the last 30 years reveals relatively low levels in relation to the threshold limits established for drinking water. In contrast, the levels in the groundwaters are much higher and would be unsuitable for drinking water use. Generally Mn is flushed into streams at the onset of significant precipitation events and concentrations increase in response to felling activities, with decay during subsequent years. High resolution monitoring reveals a wealth of dynamic features not picked up with standard monitoring programmes. The mechanisms driving these changes cannot be pinned down here. During the summer and in drought periods there is a small diurnal pattern in Mn concentrations with the highest concentrations occurring at night. During events, there may well be a complex array of inputs including groundwater and near surface inputs of variable chemistry, with the rising limb showing distinctive features as localised inputs near to the stream are flushed to the river at times when the flows have not risen sufficiently to dilute their inputs out. These features need further investigation in the context of hyperheic/groundwater transfers and within-river Mn processing.
Thus, in terms of the initial hypothesis is justified in that undertaking long-term and high intensity monitoring of Mn reveals features not observed within shorter term research programmes. This feature is now being shown to be general for a very wide range of elements.16,27 Nonetheless, as long-term and high resolution monitoring information becomes more available, new questions arise linking to issues of within-catchment complexity, long-term climatic and pollution change and the nature of within-river and hyperheic influences river water quality. The key to further understanding probably comes with combining detailed within catchment studies with long-term monitoring programmes within the context of earth observatories and universally available long-term datasets.16,41–43
Acknowledgements
The paper represents a lifetimes study for Colin Neal and Brian Reynolds. During this time so many scientist have contributed with the field work and the chemical analysis. We thank them all. We also acknowledge the contribution from the Journal's reviewers, who contributed significantly to the final version of the paper.
References
- M. Hornung, K. R. Bull, M. Cresser, J. Ullyett, J. R. Hall, S. Langan, P. J. Loveland and M. J. Wilson, Environ. Pollut., 1995, 87, 207–214 CrossRef CAS.
- J. A. Hudson, K. Gilham and I. Calder, Hydrol. Earth Syst. Sci., 1997, 1, 207–214 Search PubMed.
- C. Neal, S. J. Ormerod, S. J. Langan, T. R. Nisbet and J. Roberts, Hydrol. Earth Syst. Sci., 2004, 8, 589–595 CrossRef CAS.
-
C. B. Buckley and L. Keil, in Acid Waters in Wales, ed. R. Edwards, A. Gee and J. Stoner, Kluwer Dordrecht, 1990, pp. 145–158 Search PubMed.
- K. V. Heal, Sci. Total Environ., 2001, 265, 169–179 CrossRef CAS.
- A. M. Hardie, K. V. Heal and A. Lilly, Water, Air, Soil Pollut., 2007, 182, 369–382 CrossRef CAS.
- K. V. Heal, P. E. Kneale and A. T. Macdonald, Hydrological Sciences Journal, 2002, 47, 769–780 CrossRef CAS.
- C. Abesser, R. Robinson and C. Soulsby, J. Hydrol., 2006, 326, 59–78 CrossRef.
- S. C. Homoncik, A. M. MacDonald, K. V. Heal, B. E. O. Dochartaigh and B. T. Ngwenya, Sci. Total Environ., 2010, 408, 2467–2473 CrossRef CAS.
-
Forestry & Water Guidelines, Forestry Commission, Edinburgh, 2003 Search PubMed.
- K. J. Sly, M. C. Hodgkinson and V. Arunpairojana, Applied Environmental Microbiology, 1990, 56, 628–639 Search PubMed.
-
N. N. Greenwood and A. Earnshaw, Chemistry of the Elements, Elsevier, Amsterdam, 2005 Search PubMed.
- C. Neal, Hydrol. Earth Syst. Sci., 2000, 4, 499–509 CrossRef.
- C. Neal, R. J. Wilkinson, M. Neal, M. L. Harrow, H. Wickham, L. Hill and C. Morfitt, Hydrol. Earth Syst. Sci., 1997, 1, 583–618 CrossRef.
- C. Neal, A. J. Robson, P. Shand, W. M. Edmunds, A. J. Dixon, D. K. Buckley, S. Hill, M. L. Harrow, M. Neal and B. Reynolds, Hydrol. Earth Syst. Sci., 1997, 1, 3–18 CrossRef.
- C. Neal, B. Reynolds, P. Rowland, D. Norris, J. W. Kirchner, M. Neal, D. Sleep, A. Lawlor, C. Woods, S. Thacker, H. Guyatt, C. Vincent, K. Hockenhull, H. Wickham, S. Harman and L. Armstrong, Sci. Total Environ., 2011 Search PubMed , in press.
- J. W. Kirchner, X. Feng, C. Neal and A. J. Robson, Hydrol. Processes, 2004, 18, 1353–1359 CrossRef.
- C. Brandt, M. Robinson and J. W. Finch, Hydrol. Earth Syst. Sci., 2004, 8, 345–354 CrossRef CAS.
- C. Neal, B. Reynolds, M. Neal, B. Pugh and H. Wickham, Hydrol. Earth Syst. Sci., 2001, 5, 459–476 CrossRef.
- C. Neal, Hydrol. Earth Syst. Sci., 1997, 1, 381–764 Search PubMed.
- J. A. Hudson, S. B. Crane and J. R. Blackie, Hydrol. Earth Syst. Sci., 1997, 1, 409–427 CrossRef.
- J. Wilkinson, B. Reynolds, C. Neal, S. Hill, M. Neal and M. Harrow, Hydrol. Earth Syst. Sci., 1997, 1, 557–569 CrossRef.
- B. Reynolds and C. Neal, J. Environ. Qual., 1991, 20, 518–521 CrossRef.
- C. Neal, G. P. Ryland, T. Conway, H. A. Jeffery, M. Neal, A. J. Robson, C. J. Smith, J. Walls and C. L. Bhardwaj, Sci. Total Environ., 1994, 142, 127–141 CrossRef CAS.
- M. Niklinska, M. Maryanski, G. Szarek and R. Laskowski, Water, Air, Soil Pollut., 1995, 85, 1771–1776 CrossRef CAS.
- R. J. Skrivan, J. Rusek, D. Fottova, M. Burian and L. Minarik, Water, Air, Soil Pollut., 1995, 85, 841–846 CrossRef.
- A. P. Rowland, C. Neal, B. Reynolds, H. P. Jarvie, D. Sleep, A. J. Lawlor and M. Neal, J. Environ. Monit., 2011, 13, 1255–1263 RSC.
- B. Reynolds, C. Neal., M. Hornung and P. A. Stevens, J. Hydrol., 1986, 87, 167–185 CrossRef CAS.
- C. Neal, B. Reynolds, M. Neal and B. Williams, Hydrol. Earth Syst. Sci., 2004, 8, 460–484 CrossRef CAS.
- P. Durand, C. Neal, H. A. Jeffery, G. P. Ryland and M. Neal, J. Hydrol., 1994, 157, 139–156 CrossRef CAS.
-
N. Breward, University of Leeds Unpublished, 1990.
- N. Breward, Geochemical cycling in the Wye catchment. Part 1. Geochemical relationships in the bedrock and stream sediments of the Cyff system, 1985 Search PubMed.
- D. Peachey and N. Breward, A comparative study of the results obtained from the analysis of geological samples by various methods, 1980 Search PubMed.
- J. W. Kirchner, X. Feng and C. Neal, Nature, 2000, 403, 524–527 CrossRef CAS.
- J. W. Kirchner, X. Feng and C. Neal, J. Hydrol., 2001, 254, 81–100 CrossRef.
- C. Neal and J. W. Kirchner, Hydrol. Earth Syst. Sci., 2000, 4, 295–310 CrossRef.
- S. E. Godsey, W. Aas, T. A. Clair, H. A. de Wit, I. J. Fernandez, J. S. Kahl, I. A. Malcolm, C. Neal, M. Neal, S. J. Nelson, S. A. Norton, M. C. Palucis, B. L. Skjelkvåle, S. Soulsby, D. Tetzlaff and J. W. Kirchner, Hydrol. Processes, 2010, 24, 1660–1671 CrossRef CAS.
- D. A. Nimick, C. H. Gammons, T. E. Cleasby, J. P. Madison, D. Skaar and C. M. Brick, Water Resour. Res., 2003, 39, 1247 CrossRef.
- C. Neal, Sci. Total Environ., 1988, 76, 279–283 CrossRef CAS.
- D. T. Scott, D. M. McKnight, B. M. Voelker and D. C. Hrncir, Environ. Sci. Technol., 2002, 36, 453–459 CrossRef CAS.
- C. Neal, P. Rowland, P. Scholefield, C. Vincent and D. Woods, Sci. Total Environ., 2011, 409, 1516–1529 CrossRef CAS.
- C. Neal, B. Reynolds, D. Norris, J. W. Kirchner, M. Neal, P. Rowland, H. Wickham, S. Harman, L. Armstrong, D. Sleep, A. Lawlor, C. Woods, B. Williams, M. Fry, G. Newton and D. Wright, Hydrol. Processes, 2011 DOI:10.1002/hyp.8191.
- C. Neal, M. Bowes, H. P. Jarvie, P. Scholefield, G. J. L. Leeks, M. Neal, P. Rowland, H. Wickham, S. Harman, L. Armstrong, D. Sleep, A. Lawlor and C. E. Davies, Hydrol. Process. (HP Today), 2011 Search PubMed , in press.
|
This journal is © The Royal Society of Chemistry 2012 |
Click here to see how this site uses Cookies. View our privacy policy here.