DOI:
10.1039/C0SM00797H
(Paper)
Soft Matter, 2011,
7, 220-224
Photochemical control of membrane raft organization
Received
10th August 2010
, Accepted 14th September 2010
First published on 9th October 2010
Abstract
Controllable membrane phase separation through the action of a synthetic photoresponsive amphiphile is reported. We studied multi-component giant vesicles formed from a ternary lipid mixture of saturated and unsaturated phospholipid and cholesterol together with the photoresponsive amphiphile. A change in the conformation of the photoresponsive amphiphile can switch membrane lateral segregation in a reversible manner. Cis-isomerization induces lateral phase separation in one-phase membranes or produces additional lateral domains in two-phase membranes. Membranes that are close to miscibility boundary show high photo-responsiveness. This is the first report on the reversible control of membrane lateral segregation triggered by a conformational change in a membrane-constituting molecule. These findings may lead to new methods for controlling membrane self-organization such as raft engineering.
Introduction
The regulation of lateral organization in plasma membranes is essential for physiological functions.1 Within fluid membranes, microdomains called lipid rafts, which are composed largely of cholesterol and saturated lipids, are laterally segregated with high lipid order and slow dynamics.2 During molecular-associating events at a membrane interface, such as signaling and trafficking, stabilized microdomains are temporally produced to effectively concentrate specific molecules.3 It is hypothesized that raft-stabilizing proteins such as glycosylphosphatidylinositol-anchored proteins (GPI-APs) promote the formation of microdomains.4 However, the mechanism of the molecular triggering of microdomain formation is still unclear.
Membrane lateral segregations are considered to be a form of liquid-order (Lo)–liquid-disorder (Ld) phase separation that develops due to the interaction between lipid molecules; Lo and Ld phases correspond to rafts and the surrounding fluid membrane, respectively.1 To reveal the physicochemical properties of membrane microdomains, studies on model membrane systems using artificial lipid vesicles have been performed.5 Recently, with regard to the molecular mechanism for stabilizing microdomains, it has been suggested that subtle changes in membrane components at the molecular level, induced by protein binding,6actin polymerization,7 photoresponsive cholesterol,8 and lipid peroxidation,9 can affect membrane lateral segregation. Hammond et al., reported that clustering GM1 with cholera toxin B (CTB) increased miscibility transition temperature to cause a homogeneous membrane to phase separate into domains.6 Liu and Fletcher also reported that actin cytoskeleton through a phosphatidylinositol 4,5 bisphosphate (PIP2) - neural Wiskott-Aldrich syndrome protein (N-WASP) link elevated miscibility temperature to stabilize phase-separated domains.7 Yasuhara et al., observed changes in domain structures under cis-isomerization of an azobenzene-modified cholesterol.8 Ayuyan and Cohen reported that photooxidation promoted membrane phase separation.9
Previously, we reported that a synthetic photoresponsive amphiphile (KAON12), the molecular conformation of which can be switched by light irradiation (Scheme 1), can alter the mechanical properties of lipid bilayers to induce shape transitions of homogeneous vesicles, such as budding and hole opening.10 In the present study, we used KAON12 to achieve the controllable formation of lateral domains within heterogeneous membranes. We studied multi-component giant vesicles formed from a ternary lipid mixture of saturated and unsaturated phospholipid and cholesterol together with this photoresponsive amphiphile. To the best of our knowledge, this is the first report on the reversible control of membrane lateral segregation triggered by a conformational change in a membrane-constituting molecule.
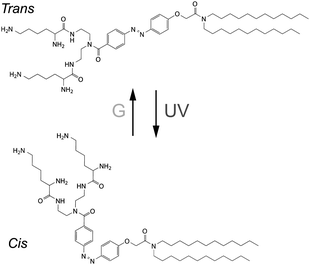 |
| Scheme 1 Chemical formula of KAON12. | |
Results
Lateral membrane phase separation
Vesicles were prepared from a lipid mixture of the photoresponsive amphiphile KAON12, dioleoyl phosphatidylcholine (DOPC), dipalmitoyl phosphatidylcholine (DPPC) and cholesterol (Chol). The ternary system of DOPC/DPPC/Chol shows phase separation between a Lo phase composed largely of DPPC and Chol, and a Ld phase which is rich in DOPC.5 The membranes contain 1% of the fluorescent phospholipid rhodamine red-X dihexadecanoyl phosphoethanolamine (rho-PE), which preferentially partitions into the Ld phase.5Fig. 1a and b show typical microscopic images of one-phase and two-phase vesicles, respectively, with a composition of 60 mol % lipids (DOPC:DPPC:Chol = 24
:
12
:
24) and 40 mol % KAON12. The isomer of KAON12 is the trans form. Fig. 1c shows the probability of the one-phase and two-phase vesicles as a function of the DOPC:DPPC ratio under a constant fraction of Chol:KAON12 = 24
:
40. Membranes with a higher DPPC ratio tend to exhibit phase separation into domains. The coexistence of one-phase and two-phase vesicles at DOPC:DPPC = 21
:
15 is probably due to a wide variation in the mixed fraction of lipids during the vesicle preparation. We consider that KAON12 is rich in the Ld region similar to DOPC, since it has been previously reported that binary DOPC/KAON12 membranes do not show phase separation.10
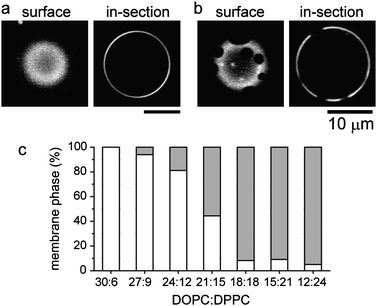 |
| Fig. 1 Typical microscopic images of one-phase (a) and two-phase (b) vesicles of DOPC/DPPC/Chol/KAON12. (c) Probability of one-phase and two-phase vesicles as a function of the DOPC:DPPC ratio, with 24% Chol and 40% trans-KAON12. The number of vesicles observed for each DOPC:DPPC ratio are N = 36 (30 : 6), 50 (27 : 9), 90 (24 : 12), 81 (21 : 15), 85 (18 : 18), 43 (15 : 21), and 39 (12 : 24). White and gray boxes indicate one-phase and two-phase vesicles, respectively. | |
Photocontrolled membrane phase organization
First, we investigated the effect of photoirradiation on one-phase membranes with trans-KAON12, and found a photo-induced reversible change in membrane phase separation. Fig. 2a shows a typical micrograph of the reversible molecular switching of membrane phase organization (also see a movie and pictures of other giant vesicles in the Supplementary Information†), where the vesicle is composed of DOPC:DPPC:Chol:KAON12 = 24
:
12
:
24
:
40 (at this lipid composition, the percentage of the one-phase vesicles with trans-KAON12 is 81% as shown in Fig. 1c). Before UV irradiation, the trans-membrane surface is homogeneous without domains. Immediately after the azobenzene isomer is switched from the trans to cis form by irradiation with UV light, many small domains appear over the entire membrane (Fig. 2a upper). The generated domains exhibit random thermal motion, and the domains become larger through collision and fusion during such motion. Thus, the photo-isomerization of KAON12 from the trans- to cis-form induced membrane phase separation. The fluorescence intensities of rho-PE on the membrane surface clearly indicate a difference in lateral organization between the one-phase trans-membrane and two-phase cis-membrane (Fig. 2b). Interestingly, the two-phase membrane reverted to one-phase by trans-isomerization (i.e., upon the discontinuation of UV irradiation during treatment with green light) (Fig. 2a lower). Thus, cis-isomerization induced stabilized lateral domains, and these domains were again destabilized and disappeared under trans-isomerization (Fig. 2c).
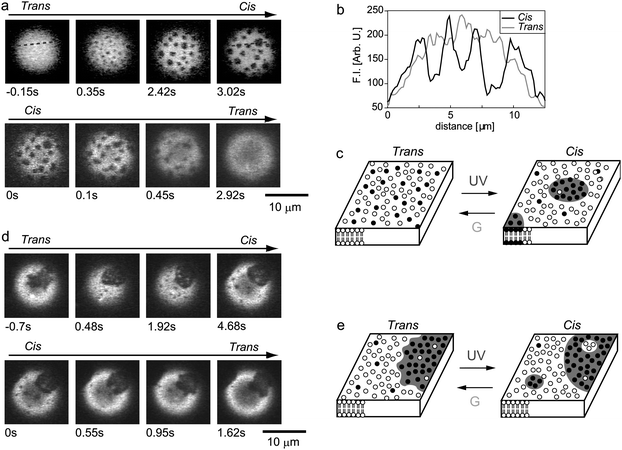 |
| Fig. 2 Photo-induced reversible organization of lateral domains in one-phase (a–c) and two-phase (d, e) bilayer membranes. (a, d) Fluorescence photomicrographs. The time elapsed is shown on each micrograph. (b) Fluorescence intensities (F.I.) for rho-PE of trans- and cis-membranes along the dashed line in (a). (c, e) Schematic representation of the molecular switching of the membrane organization. Open circles represent DOPC and KAON12, and filled circles show DPPC and Chol. | |
Next, we focused on two-phase membranes, i.e., phase-separated vesicles with trans-KAON12. Fig. 2d shows typical micrograph of the reversible photo-switching of membrane phase organization (also see a movie and pictures of other giant vesicles in the Supplementary Information†), where the vesicle is composed of DOPC:DPPC:Chol:KAON12 = 24
:
12
:
24
:
40 (at this lipid composition, the percentage of the two-phase vesicles with trans-KAON12 is 19% as shown in Fig. 1c). Before UV irradiation, the trans-membrane surface is covered by domains. Notably, thermal fluctuation of the domain boundary was occasionally observed with this membrane composition (35% of the two-phase vesicles exhibited such fluctuating domains). When we irradiated the membrane with UV light to induce cis-isomerization of KAON12, additional small domains appeared within the membrane surface (Fig. 2d upper): Lo domains emerged in the Ld phase region, and Ld domains appeared in the Lo phase regions. Furthermore, fluctuation of the domain boundary was suppressed under cis-isomerization, which indicates that cis-membranes have greater line tension than trans-membranes. Upon trans-isomerization, the produced domains again disappeared, and phase fluctuation recovered (Fig. 2d lower). Schematic illustrations are shown in Fig. 2e. These reversible changes in membrane phase organization in Fig. 2 were observed more than 10 times. Thus, under UV irradiation, initially uniform one-phase membranes show phase separation into two microscopic coexisting phases, whereas initially two-phase membranes produced additional domains within the membranes. This photo-switching in membrane phases can be observed with a molar fraction of KAON12 of above ∼30%.
Photo-responsiveness of membrane phase organization
The responsiveness of membrane phase organization upon photoisomerization, as shown in Fig. 2, largely depended on the composition of ternary lipids (DOPC/DPPC/Chol). We characterized the photo-responsiveness of the membrane system as a function of the DOPC:DPPC ratio with different Chol fractions (Fig. 3). Photo-induced changes in membrane phase separation were most frequently observed, provided that membrane ternary compositions were close to the miscibility boundary that separates one- and two-phase states. In the situation close to miscibility, thermal fluctuation of the domain boundary was often observed in the two-phase membrane surfaces, and all of the fluctuating domains responded as shown in Fig. 2d. In contrast, membranes with compositions far from the miscibility boundary do not respond to photo-isomerization. Photo-isomerization of KAON12 has little effect on membranes with a low Chol concentration of 12%.
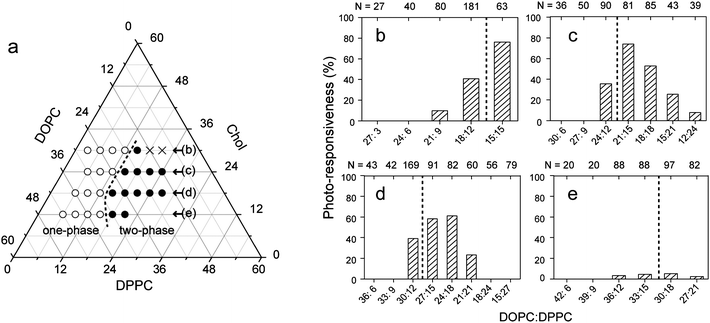 |
| Fig. 3 Responsiveness of membrane phase organization upon photo-isomerization. (a) Phase diagram of ternary DOPC/DPPC/Chol membranes with 40% trans-KAON12. The open and filled circles correspond to one-phase (homogeneous) membranes without domains and two-phase membranes with domains, respectively. The cross indicates no vesicles. (b–e) Percentage of photo-responsiveness as a function of the DOPC:DPPC ratio with each Chol concentration as shown in part (a): 30% (b), 24% (c), 18% (d), and 12% (e). Responsiveness is defined as the number of vesicles that show photo-induced phase dynamics, which include both initially one-phase and two-phase membranes as shown in Fig. 2 (see Supplementary Information†), divided by the total number of observed vesicles. The broken lines show the miscibility boundary. | |
Discussion
With regard to thermodynamic phase separation, the stability of bilayer miscibility, such as the emergence of raft domains, is determined by the interfacial energy at the domain edge, i.e., line tension. When the interfacial energy is as low as the thermal energy, domain edge fluctuation is visible optically, which was previously observed in lipid membranes near miscibility critical points.11 As the temperature is increased toward the miscibility temperature, edge fluctuation increases, and two-phase membranes transition into a one-phase state without domains. Line tension σ changes depending on the difference between the system temperature T and the miscibility critical temperature Tc as σ ∼ (Tc − T)ν, where ν is the critical exponent.11 In this study, we observed such change in the edge fluctuation associated with photo-induced phase reorganization, which suggests that the molecular switching of line tension may be the mechanism for photocontrolled domain formation. The change in the molecular conformation of KAON12 into its bulky cis-isomer probably increases the miscibility temperature, consequently leading to the increase in line tension. In other words, miscibility boundary shifts in the isothermal phase diagram under photo-isomerization. It has been reported that the hydrophobic mismatch between the two phases elevates the miscibility temperature.12Cis-isomer has a looser packing configuration than trans-isomer at the interface, which possibly enhance such hydrophobic mismatch. Further experiments on the quantitative analysis of the change in line tension are underway.
Yasuhara et al., reported that cis-isomerization of an azobenzene-modified cholesterol destabilize lateral domains,8 which is opposite to our results. This may be attributed to the difference in partitioning membrane phase between these two molecules: our KAON12 is rich in the Ld region, while their azobenzene-modified cholesterol is preferentially distributed into the Lo region. When molecular packing in the Ld region becomes much looser under cis-isomerization, hydrophobic mismatch between the two phases is enhanced. Conversely, looser molecular packing in the Lo region suppresses the hydrophobic mismatch. Thus, cis-isomerization in the Ld phase tends to increase the miscibility temperature to induce phase-separation into domains, while cis-isomerization in the Lo phase decreases the miscibility temperature to destabilize domains. Cell membranes are crowded with transmembrane (TM) proteins that are laterally sorted in Lo (raft) or Ld (non-raft) phase regions according to their preferences for membrane order.4Cells may take advantage of the activation of raft or non-raft TM proteins to effectively control membrane domains. Differences in mechanical properties, such as membrane tension, of the model membranes from those of the plasma membranes should be taken into consideration and investigated. Further studies of model and actual plasma membranes are awaited in order to fully understand the molecular mechanism to regulate membrane organization.
Recently, using giant vesicle systems, Ayuyan and Cohen reported that photo-induced lipid peroxidation induced uniform vesicles to phase separate.9 The mechanism is proposed to be the formation of various amounts of oxidation products within the bilayer. Oxidative conformational changes, such as cleavage of the alkyl chains at the site of a double bond, lead to molecular packing arrangement into membrane domains. However, lipid oxidation is a complex process, and it is hard to extract and quantify the products. There remains unknown parameter concerning the change in molecular conformation. Conversely, our membrane system can provide an efficient tool to examine the effect of the changes in membrane materials on self-assembled mesoscopic structures because the photoresponsive molecule can be switched just between trans- and cis-form reversibly.
We found that membranes that were close to the miscibility boundary showed higher photo-responsiveness, such as 76% (DOPC:DPPC:Chol = 15
:
15
:
30) and 74% (DOPC:DPPC:Chol = 21
:
15
:
24). The existence of other vesicles that do not response even in the situation close to miscibility is probably due to a wide variation in the mixed fraction of KAON12 during the vesicle preparation, as previously reported.10
Here, we examined lateral phase separation of multi-component membranes with 40% KAON12 under photo-isomerization. We confirmed that this photo-switching in membrane phases can be observed with a molar fraction of KAON12 of above ∼30%. No apparent difference in the photo-induced phase behavior, as shown in Fig. 2, was noted between these different fractions of KAON12. Notably, at a KAON12 concentration above 50%, we could not obtain any giant vesicles. The necessary amount of KAON12 (∼30%) for the photo-induced microscopic phase organization is nearly equal to the amount for the previously reported shape transitions of homogeneous giant vesicles: ∼20% and ∼30% of KAON12 were required to induce the vesicular transformations of closed vesicles and the formation of membrane pores, respectively.10 Trials on the molecular design of photoresponsive amphiphiles toward the elegant optical control of self-assembled membranes with their smaller amount are awaited.
Conclusions
We succeeded in controlling membrane phase separation of giant vesicles through the action of a photoresponsive amphiphilic molecule (KAON12). Photo-isomerization induces lateral phase separation in one-phase membranes or produces additional lateral domains in two-phase membranes, in a reversible manner. Our results indicate that, provided that membranes are close to miscibility, molecular perturbations, such as isomerization, can lead to a profound change in laterally organized structures to trigger the formation of raft domains. We discussed the mechanism of this photocontrolled membrane phase separation in terms of the change in the miscibility temperature due to the switching of molecular packing configuration. These findings may contribute to a better understanding of the molecular mechanism for regulating plasma membrane rafts, and may lead to new methods for controlling mesoscopic membrane structures. As the next research target, it may of value to try to apply the methodology on the investigation of the interleaflet interactions of heterogeneous bilayer membranes, by preparing asymmetric bilayers with the photoresponsive amphiphile just on one side.13
Experimental
Materials
We designed and synthesized a photosensitive amphiphilic molecule containing azobenzene (KAON12); the details of synthesis have been described previously.8 The conformation (trans or cis) of this molecule can be switched by irradiation with light (Scheme 1). 1,2-Dioleoyl-sn-glycero-3-phosphocholine (DOPC), 1,2-dipalmitoyl-sn-glycero-3-phosphocholine (DPPC) and cholesterol (Chol) were purchased from Avanti Polar Lipids (Alabaster, USA). The fluorescent lipid N-(rhodamine red-X)-1,2-dihexadecanoyl-sn-glycero-3-phosphoethanolamine triethylammonium salt (rho-PE, λex = 560 nm, λem = 580 nm) was obtained from Invitrogen. Deionized water, obtained from a Millipore Milli Q purification system, was used to prepare reagents.
Preparation of giant vesicles
Giant vesicles were prepared by the natural swelling method from dry lipid films;8lipid mixtures of KAON12, DOPC, DPPC and Chol dissolved in methanol–chloroform (1
:
3, v/v) along with rho-PE in a glass test tube were dried under vacuum for 3 h to form thin lipid films. The films were then hydrated overnight with deionized water at 37 °C. The final concentration was 0.2 mM of lipids (DOPC/DPPC/Chol/KAON12) and 1 mol% rho-PE.
Microscopic observation under photoirradiation conditions
The sample solution (3 μL) was placed on a glass coverslip, which was covered with another smaller coverslip at a spacing of ca. 0.1 mm. We observed changes in membrane morphology with a fluorescence microscope (IX71, Olympus, Japan) equipped with a confocal scanner (CUS-10, Yokogawa, Japan), at room temperature. A 532 nm laser was used to excite rho-PE, and for trans-isomerization. Cis-isomerization was achieved by UV irradiation through a standard filter set (WU, Olympus; λex = 330–385 nm, dichroic mirror 400 nm, λem = 420 nm), with the use of an extra-high-pressure mercury lamp (100 W). The molecular conformation (trans or cis) of KAON12 was switched by photo-irradiation with green light either with or without UV light (see Supplementary Information†). The power of the irradiated light on the microscopy stage was 72 μW (green) and 552 μW (UV), which was measured using an optical power measurement system (X-Cite XP750 & XR2100, EXFO, Canada). The images were recorded on a hard-disc drive at 30 frames s−1.
Acknowledgements
This work was supported by a Grant-in-Aid for Scientific Research on Priority Areas “Soft Matter Physics” and “Bio Manipulation” from the MEXT of Japan, and by the Sunbor Grant from the Suntory Institute for Bioorganic Research.
Notes and references
- D. Lingwood and K. Simons, Science, 2010, 327, 46 CrossRef CAS
.
-
(a) K. Simons and E. Ikonen, Nature, 1997, 387, 569 CrossRef CAS
;
(b) D. A. Brown and E. London, J. Biol. Chem., 2000, 275, 17221 CrossRef CAS
.
- A. Kusumi, I. Koyama-Honda and K. Suzuki, Traffic, 2004, 5, 213 CrossRef CAS
.
- J. F. Hancock, Nat. Rev. Mol. Cell Biol., 2006, 7, 456 CrossRef CAS
.
-
(a) T. Baumgart, S. T. Hess and W. W. Webb, Nature, 2003, 425, 821 CrossRef CAS
;
(b) M. Staykova, R. Lipowsky and R. Dimova, Soft Matter, 2008, 4, 2168 RSC
;
(c) T. Hamada, Y. Miura, K. Ishii, S. Araki, K. Yoshikawa, M. Vestergaard and M. Takagi, J. Phys. Chem. B, 2007, 111, 10853 CrossRef CAS
;
(d) L. Bagatolli and P. B. S. Kumar, Soft Matter, 2009, 5, 3234 RSC
.
- A. T. Hammond, F. A. Heberle, T. Baumgart, D. Holowka, B. Baird and G. W. Feigenson, Proc. Natl. Acad. Sci. U. S. A., 2005, 102, 6320 CrossRef CAS
.
- A. P. Liu and D. A. Fletcher, Biophys. J., 2006, 91, 4064 CrossRef CAS
.
- K. Yasuhara, Y. Sasaki and J. Kikuchi, Colloid Polym. Sci., 2008, 286, 1675 CrossRef CAS
.
-
(a) A. G. Ayuyan and F. S. Cohen, Biophys. J., 2006, 91, 2172 CrossRef CAS
;
(b) L. S. Hirst and J. Yuan, Liq. Cryst., 2009, 36, 739 CrossRef CAS
;
(c) N. F. Morales-Penningston, J. Wu, E. R. Farkas, S. L. Goh, T. M. Konyakhina, J. Y. Zheng, W. W. Webb and G. W. Feigenson, Biochim. Biophys. Acta, Biomembr., 2010, 1798, 1324 CrossRef CAS
.
-
(a) T. Hamada, Y. T. Sato, T. Nagasaki and K. Yoshikawa, Langmuir, 2005, 21, 7626 CrossRef CAS
;
(b) K. Ishii, T. Hamada, M. Hatakeyama, R. Sugimoto, T. Nagasaki and M. Takagi, ChemBioChem, 2009, 10, 251 CrossRef CAS
;
(c) T. Hamada, R. Sugimoto, M. C. Vestergaard, T. Nagasaki and M. Takagi, J. Am. Chem. Soc., 2010, 132, 10528 CrossRef CAS
.
-
(a) A. R. Honerkamp-Smith, P. Cicuta, M. D. Collins, S. L. Veatch, M. den Nijs, M. Schick and S. L. Keller, Biophys. J., 2008, 95, 236 CrossRef CAS
;
(b) S. L. Veatch, P. Cicuta, P. Sengupta, A. Honerkamp-Smith, D. Holowka and B. Baird, ACS Chem. Biol., 2008, 3, 287 CrossRef CAS
;
(c) B. L. Stottrup, A. M. Heussler and T. A. Bibelnieks, J. Phys. Chem. B, 2007, 111, 11091 CrossRef CAS
;
(d) C. Esposito, A. Tian, S. Melamed, C. Johnson, S.-Y. Tee and T. Baumgart, Biophys. J., 2007, 93, 3169 CrossRef CAS
.
- A. J. Garcia-Sáez, S. Chiantia and P. Schwille, J. Biol. Chem., 2007, 282, 33537 CrossRef CAS
.
-
(a) M. D. Collins and S. L. Keller, Proc. Natl. Acad. Sci. U. S. A., 2008, 105, 124 CrossRef CAS
;
(b) T. Hamada, Y. Miura, Y. Komatsu, Y. Kishimoto, M. Vestergaard and M. Takagi, J. Phys. Chem. B, 2008, 112, 14678 CrossRef CAS
;
(c) S. May, Soft Matter, 2009, 5, 3148 RSC
.
Footnote |
† Electronic supplementary information (ESI) available: Pictures of other giant vesicles, percentage of membrane phase and photoresponse for each membrane composition, and movies of photo-induced membrane phase changes corresponding to Fig. 2a and d. See DOI: 10.1039/c0sm00797h |
|
This journal is © The Royal Society of Chemistry 2011 |
Click here to see how this site uses Cookies. View our privacy policy here.