DOI:
10.1039/C1RA00209K
(Paper)
RSC Adv., 2011,
1, 408-414
Effect of different additives on the hydrogen storage properties of the MgH2-LiAlH4 destabilized system
Received
25th May 2011
, Accepted 17th June 2011
First published on 10th August 2011
Abstract
The hydrogen storage properties of the MgH2-LiAlH4 (4
:
1) composite system with and without additives were studied. 5 wt.% of TiF3, NbF5, NiF2, CrF2, YF3, TiCl3·1/3AlCl3, HfCl4, LaCl3, CeCl3, and NdCl3, respectively, was added to the MgH2-LiAlH4 (4
:
1) mixture, and their catalytic effect was investigated. Temperature programmed desorption results show that addition of metal halides to the MgH2-LiAlH4 (4
:
1) composite system improves the onset desorption temperature. The hydrogen desorption properties of metal halide-doped MgH2-LiAlH4 (4
:
1) composites were also improved as compared to the undoped MgH2-LiAlH4 (4
:
1) composite. Furthermore, the activation energy and the change in enthalpy in the doped and undoped composite were measured by differential scanning calorimetry. In addition, the reaction pathway of the MgH2-LiAlH4 (4
:
1) composite system and the mechanisms that work in this composite during the de/re-hydrogenation process were determined by X-ray diffraction.
1. Introduction
As one of the ideal candidates as an energy carrier for both mobile and stationary applications, hydrogen is also considered as a material that can avert adverse effects on the environment and reduce dependence on imported oil for countries without natural resources.1 There are many approaches to store hydrogen, including high pressure, cryogenics, and chemical compounds that reversibly release H2 upon heating (solid state storage). Storing hydrogen in solid state form offers several benefits over a pressurized gas or a cryogenic liquid form. Among the solid-state hydrogen storage materials, much work has been focused on MgH2, due to its high hydrogen capacity (7.6 wt%), with the added advantages of low cost,2,3 and superior reversibility.4 However, MgH2 only starts to desorb hydrogen above 300 °C,5 and has slow desorption kinetics.6 These disadvantages have been overcome by reducing the particle size,7 doping with catalysts,8–10 and reacting with other metal hydrides (destabilization concept).11–16 The destabilization concept has been extensively investigated as an approach aimed at modifying the thermodynamics and kinetics of the hydrogen sorption reaction. Vajo et al.17 destabilised MgH2 by adding Si. The results indicated that the MgH2/Si system could be practical for hydrogen storage at reduced temperature. However, the formation of Mg2Si would reduce the gravimetric hydrogen density because Si cannot be hydrogenated, so this intermediate phase seems hard to make reversible. Zhang et al.11 showed that MgH2 can be destabilized effectively by LiAlH4. They found that the reaction enthalpy of the MgH2-relevant decomposition in MgH2-LiAlH4 composites (1
:
1, 1
:
2, and 4
:
1 in mole ratio) was reduced by 31, 27.4, and 15 kJ mol−1 H2 compared to as-milled pristine MgH2 (76 kJ mol−1 H2). According to Zhang et al., the hydrogen desorption is observed to take place in two stages: the first stage is the two-step decomposition of LiAlH4 as shown in eqn (1) and (2). | 3LiAlH4 → Li3AlH6 + 2Al + 3H2 | (1) |
| Li3AlH6 → 3LiH + Al + 3/2H2 | (2) |
During the second stage, the yielded LiH and Al phases decompose the MgH2 to form Li0.92Mg4.08 and Mg17Al12 phases, as shown in eqn (3) and (4). | 4.08MgH2 + 0.92LiH → Li0.92Mg4.08 + 4.54H2 | (3) |
| 17MgH2 + 12Al → Al12Mg17 + 17H2 | (4) |
The hydrogen absorption at 400 °C under 4.0 MPa hydrogen involves two reactions, as shown in eqn (5) and (6). | Li0.92Mg4.08 + 4.5H2 →? 4.08MgH2 + 0.92LiH | (5) |
| Al12Mg17 + (17 − 2y)H2 → yMg2Al3 + (17 − 2y)MgH2 + (12 − 3y)Al | (6) |
Recently, Chen et al.13 confirmed the two-stage nature of the hydrogen desorption in LiAlH4 + xMgH2 (x = 1, 2.5, and 4). They found that the two-stage decomposition was similar to what is described by eqn (1), (2), (3), and (4), but from the X-ray diffraction (XRD) pattern, the rehydrogenated composites showed the absence of an Al3Mg2 phase, which indicates that Mg17Al12 is fully transformed into MgH2 and Al as shown in reaction (7):
| Al12Mg17 + 17H2 → 17MgH2 + 12Al | (7) |
Chen
et al. found that
eqn (7) could be fully reversible without formation of Mg
2Al
3 if a high pressure of hydrogen (>10 MPa) was applied during the rehydrogenation process. Both articles claimed that the destabilization of MgH
2 by the LiAlH
4 resulted from the formation of Al
12Mg
17 and Li
0.92Mg
4.08. Although the hydrogen storage properties of MgH
2 were improved after combination with LiAlH
4, it still doesn't fulfil the requirements for practical application as a suitable hydrogen storage material. Therefore, it is of importance to find a
catalyst or additive that can improve the hydrogen storage properties of the MgH
2-LiAlH
4 composite system.
In this paper we report the hydrogen storage properties of MgH2-LiAlH4 (4
:
1) composite system with additives including 5 wt.% of TiF3, NbF5, NiF2, CrF2, YF3, TiCl3·1/3(AlCl3), HfCl4, LaCl3, CeCl3, and NdCl3, respectively, and investigated the additives' catalytic effects.
2. Experimental details
MgH2 (hydrogen storage grade), LiAlH4 (≥95%), TiF3, NbF5 (98%), NiF2, CrF2 (98%), YF3 (99.9%), TiCl3 1/3(AlCl3), and HfCl4 (98%) were purchased from Sigma Aldrich. LaCl3 (99.9%), CeCl3 (99.5%), and NdCl3 (99.9%) were obtained from Alfa Aesar. Ball milling (BM) of MgH2 and LiAlH4 powders in the mole ratio of 4
:
1 was performed in a planetary ball mill (QM-3SP2) for 18 min at the rate of 400 rpm. For simplicity, the mixture of MgH2 and LiAlH4 with molar ratio of 4
:
1 will be referred to as MgH2-LiAlH4 composite. Handling of the samples was conducted in an MBraun Unilab glove box filled with high-purity Ar atmosphere. Samples were put into a sealed stainless steel vial together with hardened stainless steel balls. The ratio of the weight of balls to the weight of powder was 30
:
1. 5 wt.% of TiF3, NbF5, NiF2, CrF2, YF3, TiCl3·1/3AlCl3, HfCl4, LaCl3, CeCl3, and NdCl3 was respectively mixed with MgH2-LiAlH4 under the same conditions to investigate their catalytic effects. Pure MgH2 and LiAlH4 were also prepared under the same conditions for comparison purposes.
The temperature programmed desorption (TPD) and re/de-hydrogenation kinetics experiments were performed in a Sieverts-type pressure-composition-temperature (PCT) apparatus (Advanced Materials Corporation). The sample was loaded into a sample vessel in the glove box. For the TPD experiment, all the samples were heated in a vacuum chamber, and the amount of desorbed hydrogen was measured to determine the lowest decomposition temperature. The heating rate for the TPD experiment was 5 °C/min, and samples were heated from room temperature to 450 °C. The re/de-hydrogenation kinetics measurements were performed at the desired temperature with initial hydrogen pressures of 3.0 MPa and 0.001 MPa, respectively.
The phase structures of the samples before and after desorption, as well as after rehydrogenation, were determined by X-ray diffraction (XRD, GBC MMA X-ray diffractometer with Cu Kα radiation). Before measurement, a small amount of sample was spread uniformly on the sample holder, which was wrapped with a plastic wrap to prevent oxidation. θ-2θ scans were carried out over diffraction angles from 25° to 80° with a speed of 2.00°/min.
Differential scanning calorimetry (DSC) analysis of the dehydrogenation process was carried out on a Mettler Toledo TGA/DSC 1. About 2–6 mg of sample was loaded into an alumina crucible in the glove box. The crucible was then placed in a sealed glass bottle in order to prevent oxidation during transportation from the glove box to the DSC apparatus. An empty alumina crucible was used as the reference material. The samples were heated from room temperature to 500 °C under an argon flow of 30 ml/min, and different heating rates were used.
3. Results and discussion
Fig. 1 displays the TPD performance of the MgH2-LiAlH4 composite system. The as-milled LiAlH4 and as-milled MgH2 were also included for comparison purposes. The as-milled MgH2 starts to release hydrogen at about 330 °C and desorbs about 7.1 wt.% H2 after 420 °C. Meanwhile, the as-milled LiAlH4 starts to desorb hydrogen at about 142 °C and about 173 °C for the first and second stage, respectively. For the MgH2-LiAlH4 composite, there are two significant stages of dehydrogenation that occur during the heating process. The first stage, which takes place within the temperature range from 130 to 250 °C, is attributed to the two-step decomposition of LiAlH4, as indicated in eqn (1) and (2). The second dehydrogenation stage, starting at about 270 °C and completed at about 360 °C, can be attributed to the MgH2-relevant decomposition. These two stages of dehydrogenation are of the same order as reported by Zhang et al.11 and Chen et al.13
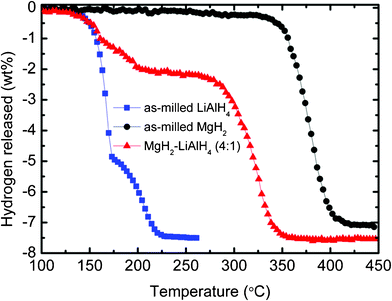 |
| Fig. 1
TPD curves of the as-milled MgH2, the as-milled LiAlH4, and the MgH2-LiAlH4 composite. | |
In order to investigate the reaction progress and mechanism, XRD measurements were performed on the MgH2-LiAlH4 composite after dehydrogenation at 250 °C and 400 °C, as shown in Fig. 2. The as-milled MgH2-LiAlH4 is also included in this figure. From Fig. 2(a), it can be seen that MgH2 and LiAlH4 phases are present in the as-milled MgH2-LiAlH4 composite. Fig. 2(b) indicates the presence of LiH and Al phases beside the MgH2 after dehydrogenation at 250 °C. The fact that no LiAlH4 phase was found indicates that the reactions in eqn (1) and (2) had been completed at this stage. After dehydrogenation at 400 °C, the XRD pattern of Fig. 2(c) reveals that the intermediate phases Li0.92Mg4.08 and Al12Mg17 were eventually formed in the composite system besides Mg. These results confirmed that the hydrogen released in the second stage is from the MgH2-relevant decomposition through the reactions in eqn (3) and (4), and the decomposition of the excess MgH2(Eqn 8), which occurs at a temperature 60 °C lower than the decomposition temperature of the pure as-milled MgH2. In this study, it was found that the decomposition temperatures of the first and second stage dehydrogenation are quite similar to what was reported by Zhang et al.,11 but slightly higher compared to those reported by Chen et al.13 This difference may due to the different duration of the milling process, as Chen et al. reported that the sample was ball milled for 10 h, as compared to 18 min in this study.
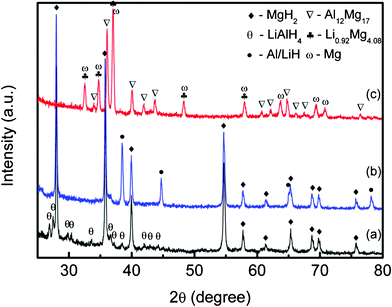 |
| Fig. 2
XRD patterns of the MgH2-LiAlH4 composite after 18 min ball milling (a), and after dehydrogenation at (b) 250 °C and (c) 400 °C. | |
Fig. 3(a) and (b) shows the influence of catalytic additives on the MgH2-LiAlH4 composite decomposition temperature as measured by temperature-programmed-desorption (TPD). The undoped MgH2-LiAlH4 is also included for comparison. Among the metal fluorides used in this study (Fig. 3(a)), TiF3 exhibits a strong catalytic influence on MgH2-LiAlH4 decomposition, followed by NiF2, CrF2, NbF5, and YF3. The TiF3-doped MgH2-LiAlH4 composite sample starts to release hydrogen at about 70 °C and about 180 °C for the first and second stage, respectively, which represents respective reductions of about 60 °C and about 90 °C compared with undoped MgH2-LiAlH4. Meanwhile, among the metal chloride additives (Fig. 3(b)), TiCl3·1/3AlCl3 shows the best catalytic effect. It seems that the catalytic effect of TiCl3·1/3AlCl3 on the decomposition temperature of MgH2-LiAlH4 composite is similar to that of TiF3, in which the decomposition temperature is reduced by about 60 °C and about 90 °C for the first and second stage, respectively, compared with undoped MgH2-LiAlH4. From the results, one finds also that apart from TiF3 and TiCl3·1/3AlCl3, all the other metal halide additives yielded no significant change in the second dehydrogenation stage of the MgH2-LiAlH4 system.
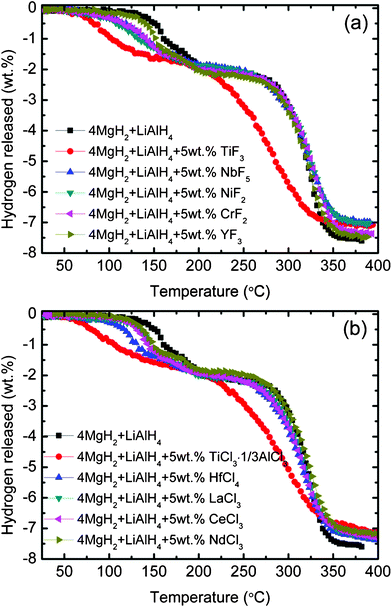 |
| Fig. 3
TPD curves of (a) the metal fluoride-added MgH2-LiAlH4 and (b) the metal chloride-added MgH2-LiAlH4 composites. | |
Fig. 4(a) and (b) compares the isothermal dehydrogenation kinetics of MgH2-LiAlH4 composite with and without metal halides at 320 °C. The dehydrogenation of MgH2 was also examined for comparison under the same conditions. At 320 °C, the pure MgH2 releases about 3.4 wt.% hydrogen after 60 min. Mixed with LiAlH4, the dehydrogenation kinetics of MgH2 was improved. The composite releases about 4.6 wt.% hydrogen after 40 min of dehydrogenation. With the addition of 5 wt.% metal halide, the results show that all the metal halides improved the dehydrogenation kinetics of MgH2-LiAlH4 compared with undoped MgH2-LiAlH4. The titanium based metal halide-added MgH2-LiAlH4 showed the best improvement, so that saturation of the dehydrogenation process for the TiF3-added MgH2-LiAlH4 sample can be achieved within 10 min, and within 20 min for the TiCl3·1/3AlCl3-added MgH2-LiAlH4 sample.
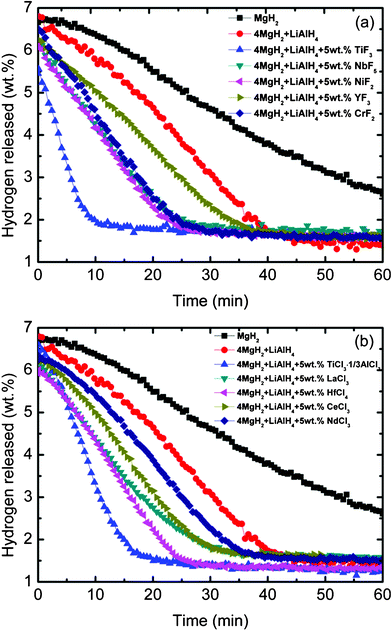 |
| Fig. 4 Isothermal dehydrogenation kinetics at 320 °C of (a) the metal fluoride-added MgH2-LiAlH4 and (b) the metal chloride-added MgH2-LiAlH4 composites. | |
Fig. 5 presents the DSC traces of the MgH2-LiAlH4 composite and the as-milled MgH2, which is also shown for comparison purposes. For the MgH2-LiAlH4 composite, the first exothermic peak at 140 °C is due to the presence of surface hydroxyl impurities in the LiAlH4 powder, as reported in our previous papers,18–20 and the first endothermic peak at 170 °C corresponds to the melting of LiAlH4.21 The second exothermic peak at 180 °C corresponds to the decomposition of liquid LiAlH4 (first step decomposition, eqn (1)), and the second endothermic peak at 225 °C is assigned to the decomposition of Li3AlH6 (second step decomposition, eqn (2)). The last endothermic peak at about 365 °C is due to the decomposition of MgH2, which occurs at a temperature 55 °C lower than that of the pure as-milled MgH2 (peak at about 420 °C). This decrease in the hydrogen release temperature is correlated with the results observed in the PCT measurement (Fig. 1).
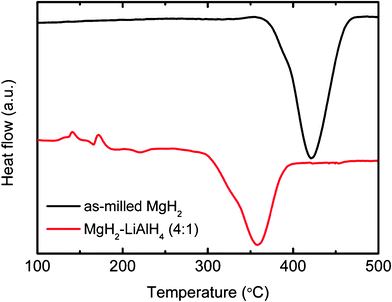 |
| Fig. 5
DSC traces of the MgH2-LiAlH4 composite and the as-milled MgH2. | |
Fig. 6 and 7 show the DSC curves for all metal halide-added MgH2-LiAlH4 samples. Apart from the YF3-added MgH2-LiAlH4 sample, the number of thermal events in all the metal halide-added MgH2-LiAlH4 samples is quite different from what occurs in the undoped MgH2-LiAlH4. These metal halide-doped MgH2-LiAlH4 samples showed one exothermic peak and two endothermic peaks. The exothermic peak and the first endothermic peak correspond to the decomposition of LiAlH4 and Li3AlH4, respectively. These results show that LiAlH4 decomposes without melting under the catalytic effects of TiF3, NiF2, CrF2, NbF5, TiCl3·1/3AlCl3, HfCl4, LaCl3, CeCl3, and NdCl3, agreeing well with the results on the 0.5 h ball-milled 4 mol% TiF3-doped LiAlH4 reported by Liu et al.22 and our previously reported NbF5-doped LiAlH4.18 For all metal halide-added MgH2-LiAlH4 samples, the second endothermic event, corresponding to the decomposition of MgH2, is similar to what occurs in the undoped LiAlH4-MgH2 sample. However, the broad peak in the TiF3 and TiCl3·1/3AlCl3-added MgH2-LiAlH4 composites indicates faster dehydrogenation kinetics.
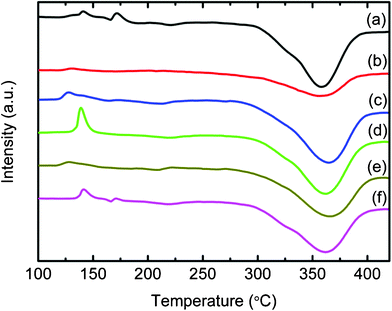 |
| Fig. 6
DSC traces of (a) the undoped MgH2-LiAlH4 and the MgH2-LiAlH4 with added (b) TiF3, (c) NbF5, (d) NiF2, (e) CrF2, and (f) YF3. | |
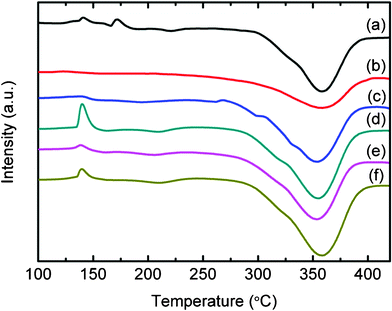 |
| Fig. 7
DSC traces of (a) the undoped MgH2-LiAlH4 and the MgH2-LiAlH4 with added (b) TiCl3·1/3AlCl3, (c) HfCl4, (d) LaCl3, (e) CeCl3, and (f) NdCl3. | |
We obtained the activation energy required for the MgH2-relevant decomposition using the Kissinger equation,23 which is usually utilized in thermal analysis as follows:
| ln[β/Tp2] = −EA/RTp + A | (9) |
where
β is the heating rate,
Tp is the peak temperature in the DSC curve,
R is the gas constant, and
A is a linear constant. Thus, the activation energy,
EA, can be obtained from the slope in a plot of ln[
β /
Tp2]
versus 1000/
Tp.
Fig. 8 shows
DSC traces for the as-milled MgH
2 and MgH
2-LiAlH
4 composite at different heating rates (
β = 10, 15, and 20 °C min
−1, respectively). From a Kissinger plot of the DSC data (inset of
Fig. 8) the apparent activation energy for the MgH
2-relevant decomposition in the MgH
2-LiAlH
4 composite is found to be 126 kJ/mol, which is much lower than the activation energy of the decomposition of as-milled MgH
2 (162 kJ/mol). This
reduction indicates that the apparent activation energy for
decomposition of hydrogen from MgH
2 was reduced by adding LiAlH
4.
Table 1 shows the apparent activation energy measured by the Kissinger method for selected metal
halide-added MgH
2-LiAlH
4 and undoped MgH
2-LiAlH
4 composites, as well as for as-milled MgH
2. The table show that, titanium-based metal
halides, TiF
3 and TiCl
3·1/3AlCl
3, exhibit the best additives in reducing the activation energy for H-desorption in the MgH
2-LiAlH
4 composites. The apparent
activation energies calculated were found to be 83 and 98 kJ/mol for the
hydride decomposition of TiF
3 and TiCl
3·1/3AlCl
3-added MgH
2-LiAlH
4 composites, respectively.
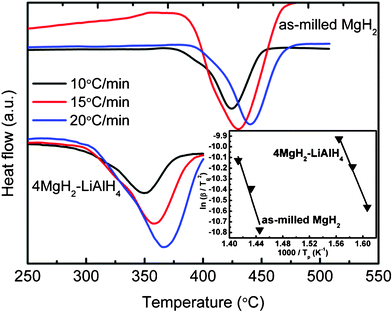 |
| Fig. 8
DSC traces of the as-milled MgH2 and the MgH2-LiAlH4 at different heating rates. The inset plot is the Kissinger's analysis for as-milled MgH2 and MgH2-LiAlH4 composite. | |
Table 1 The apparent activation energy and the enthalpy changes for as-milled MgH2, MgH2-LiAlH4 composite, and selected metal halide-added MgH2-LiAlH4
|
Non-catalysed |
4MgH2-LiAlH4 catalysed with metal halide |
As-milled MgH2 |
4MgH2-LiAlH4 |
TiF3 |
NbF5 |
NiF2 |
TiCl3·1/3AlCl3 |
HfCl4 |
LaCl3 |
Activation energy (kJ/mol) |
162 |
126 |
83 |
110 |
120 |
98 |
106 |
123 |
Enthalpy (kJmol−1 H2) |
76 |
61 |
59 |
62 |
63 |
60 |
62 |
64 |
To determine the enthalpy (ΔHdec) of MgH2 decomposition, the DSC curves were analysed by STARe software. From the integrated peak areas, the hydrogen desorption enthalpy was obtained. For the as-milled MgH2, the hydrogen desorption enthalpy can be calculated as 75.7 kJ mol−1 H2. This value is almost the same as the theoretical value (76 kJ mol−1 H2). By the same methods, the reaction enthalpies of the MgH2-LiAlH4 composite and the metal halide-added MgH2-LiAlH4 samples were determined. The enthalpy changes for selected samples are listed in Table 1. For the MgH2-LiAlH4 composite, the enthalpy change calculated from the DSC curves is 61 kJ mol−1 H2, which is lower than the overall decomposition enthalpy of pure MgH2 (75.7 kJ mol−1 H2). This result indicates that the presence of LiAlH4 destabilizes MgH2. The enthalpy change in the MgH2-LiAlH4 composite system is similar to that reported by Zhang et al.11 (61 kJ mol−1 H2). After the addition of metal halide, the enthalpy of hydrogen desorption from MgH2-LiAlH4 was similar to that of undoped MgH2-LiAlH4. Although the enthalpy reaction of these metal halide-doped MgH2-LiAlH4 composites remains unchanged, the destabilized complex hydride composites, MgH2-LiAlH4-5 wt.% metal halides, have better hydrogen storage behaviour with improved hydrogen desorption and faster desorption kinetics.
In order to investigate the reversibility of MgH2-LiAlH4 composite and metal halide-added MgH2-LiAlH4, the rehydrogenation of the dehydrogenated samples was performed under ∼3 MPa of H2 at 320 °C. The MgH2 system was also examined for comparison. However, the rehydrogenation measurements revealed that the MgH2-LiAlH4 (4
:
1) composite did not show any improvement in kinetics compared with the MgH2, as shown in Fig. 9. After 5 min, about 5.0 wt.% hydrogen was absorbed for the MgH2 while the MgH2-LiAlH4 composite just absorbed about 3.6 wt.% hydrogen after the same time. With addition of the titanium-based metal halides, the MgH2-LiAlH4 samples also did not show any improvement. Both the TiF3 and TiCl3·1/3AlCl3-added MgH2-LiAlH4 sample absorbed just about 3.3 wt.% hydrogen after 5 min rehydrogenation.
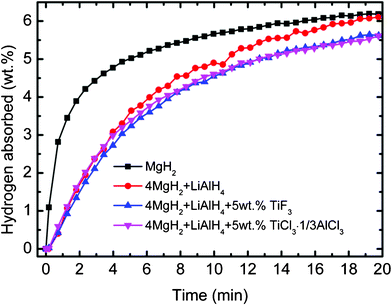 |
| Fig. 9 Isothermal rehydrogenation kinetics of the MgH2, the MgH2-LiAlH4 composite and the titanium-based metal halide-added MgH2-LiAlH4 at 320 °C and under 3 Mpa. | |
In order to determine the rehydrogenation products, XRD measurements were carried out on the rehydrogenated undoped MgH2-LiAlH4 sample, as shown in Fig. 10. From the pattern, it can be seen that the peaks correspond to MgH2, Al, and LiH, along with a small peak for Al3Mg2 that appears after rehydrogenation. The disappearance of the Al12Mg17 and Li0.92Mg4.08 peaks and the appearance of an Al3Mg2 peak in the rehydrogenated sample confirm that reactions (5) and (6) have occurred during the rehydrogenation process.
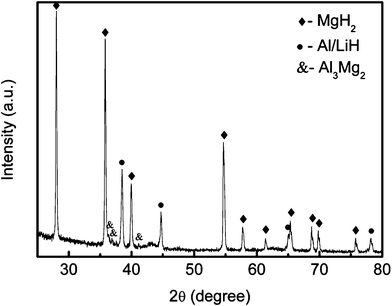 |
| Fig. 10
XRD patterns of the undoped MgH2-LiAlH4 composite after rehydrogenation at 320 °C and under 3 MPa. | |
To understand the possible mechanism behind the metal halide effects on the enhancement of MgH2-LiAlH4 composite, X-ray diffraction analysis was carried out on the TiCl3·1/3AlCl3-and TiF3-added MgH2-LiAlH4 composite. Fig. 11(a) and (b) shows the XRD patterns of the TiCl3·1/3AlCl3 and TiF3-added MgH2-LiAlH4 composites after 18 min ball milling and after dehydrogenation at 400 °C. For both samples, after 18 min ball milling, MgH2 phases are detected along with small peaks of LiAlH4, Li3AlH6, and Al (with Li3AlH6 and Al peaks overlapping in the XRD pattern). As compared with the peaks of LiAlH4 in the undoped MgH2-LiAlH4 sample (Fig. 2(a)), the diffraction peaks of LiAlH4 in the TiCl3·1/3AlCl3 and TiF3-added MgH2-LiAlH4 samples become weaker. The appearance of Li3AlH6 and Al indicates that LiAlH4 has already partly decomposed into Li3AlH6 and Al (eqn (1)) after 18 min ball milling in the presence of TiCl3·1/3AlCl3 or TiF3. After dehydrogenation at 400 °C, as compared with the undoped MgH2-LiAlH4 sample (Fig. 2(c)), the new phases Al3Ti, LiCl, and LiF were formed. The formation of Al3Ti and LiCl (Fig. 11(a)), and Al3Ti and LiF (Fig. 11(b)) may be due to the reaction of LiAlH4 with TiCl3·1/3AlCl3 or TiF3 during the ball milling or the dehydrogenation process. According to Resan et al.24 and Balema et al.25, doping LiAlH4 with Al3Ti improved the dehydrogenation behaviour. Meanwhile, a study by Yin et al.26 revealed that it was the F that actively played the catalytic role in enhancement of NaAlH4-TiF3 system. We assume that the catalytic effect of TiF3 on the dehydrogenation process of LiAlH4 could be similar to its effect on NaAlH4, as investigated by Liu et al.22 Furthermore, according to,27–29 the catalytic effect of a Ti-containing phase and the active function of the F anion have also been proved to be significant in improving the hydrogen sorption properties of MgH2. Therefore, Al3Ti and LiF are believed to act as the actual catalysts in the TiCl3·1/3AlCl3 and TiF3-added MgH2-LiAlH4 composites, which may promote the interaction of LiAlH4 and MgH2, and accelerate the hydrogen desorption process of the MgH2-LiAlH4 composite system.
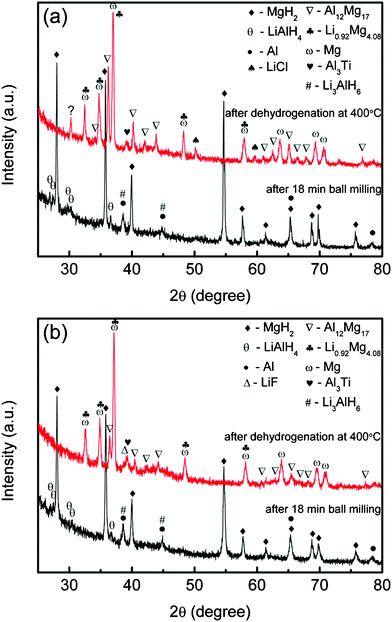 |
| Fig. 11
XRD patterns of the MgH2-LiAlH4 with addition of (a) 5 wt% TiCl3·1/3AlCl3 and (b) 5 wt.% TiF3, after 18 min ball milling and after dehydrogenation at 400 °C. | |
4. Conclusion
In the present study, the hydrogen storage properties of the MgH2-LiAlH4 (4
:
1) composite system with and without additives were investigated. It was found that the destabilization of MgH2 by the LiAlH4 resulted from the formation of Al12Mg17 and Li0.92Mg4.08 during the dehydrogenation process, furthermore improves the dehydrogenation properties of MgH2. The dehydrogenation process in the MgH2-LiAlH4 composite can be divided into two stages: the first stage is the two-step decomposition of LiAlH4. In the second stage, the yielded LiH and Al phases decompose the MgH2 to form Li0.92Mg4.08 and Al12Mg17 phases accompanied with the self-decomposition of the excessive MgH2. Among the additives examined, the titanium-based metal halides, TiF3 and TiCl3·1/3AlCl3, exhibit the best improvement in reducing the dehydrogenation temperature and enhancing the dehydrogenation rate. From the Kissinger plot, the activation energy for H-desorption is reduced from 126 kJ/mol for MgH2-LiAlH4 composite to 83 kJ/mol and 98 kJ/mol after addition of TiF3 and TiCl3·1/3AlCl3, respectively. DSC measurements indicate that the enthalpy change in the MgH2-LiAlH4 composite system was unaffected by the addition of metal halides. It is believed that the formation of Ti-containing and F-containing species during the ball milling or the dehydrogenation process may be actually responsible for the catalytic effects and thus further improve the dehydrogenation of the TiF3 and TiCl3·1/3AlCl3-added MgH2-LiAlH4 composite system.
Acknowledgements
The authors thank the University of Wollongong for financial support for this research. M. Ismail acknowledges the Ministry of Higher Education Malaysia for a PhD scholarship. Many thanks also go to Dr T. Silver for critical reading of the manuscript.
References
- B. Sakintuna, F. Lamari-Darkrim and M. Hirscher, Int. J. Hydrogen Energy, 2007, 32, 1121–1140 CrossRef CAS.
- A. Zaluska, L. Zaluski and J. O. Ström-Olsen, Appl. Phys. A: Mater. Sci. Process., 2001, 72, 157–165 CrossRef CAS.
- M. Zhu, H. Wang, L. Z. Ouyang and M. Q. Zeng, Int. J. Hydrogen Energy, 2006, 31, 251–257 CrossRef CAS.
- S. R. Johnson, P. A. Anderson, P. P. Edwards, I. Gameson, J. W. Prendergast, M. Al-Mamouri, D. Book, I. R. Harris, J. D. Speight and A. Walton, Chem. Commun., 2005, 2823–2825 RSC.
- H. Imamura, K. Masanari, M. Kusuhara, H. Katsumoto, T. Sumi and Y. Sakata, J. Alloys Compd., 2005, 386, 211–216 CrossRef CAS.
- A. Zaluska, L. Zaluski and J. O. Ström-Olsen, J. Alloys Compd., 1999, 288, 217–225 CrossRef CAS.
- J. Huot, G. Liang, S. Boily, A. Van Neste and R. Schulz, J. Alloys Compd., 1999, 293–295, 495–500 CrossRef CAS.
- A. Ranjbar, M. Ismail, Z. P. Guo, X. B. Yu and H. K. Liu, Int. J. Hydrogen Energy, 2010, 35, 7821–7826 CrossRef CAS.
- Z. S. Wronski, G. J. C. Carpenter, T. Czujko and R. A. Varin, Int. J. Hydrogen Energy, 2011, 36, 1159–1166 CrossRef CAS.
- J. Mao, Z. Guo, X. Yu, H. Liu, Z. Wu and J. Ni, Int. J. Hydrogen Energy, 2010, 35, 4569–4575 CrossRef CAS.
- Y. Zhang, Q.-F. Tian, S.-S. Liu and L.-X. Sun, J. Power Sources, 2008, 185, 1514–1518 CrossRef CAS.
- S.-S. Liu, L.-X. Sun, J. Zhang, Y. Zhang, F. Xu, Y.-H. Xing, F. Li, J. Zhao, Y. Du, W.-Y. Hu and H.-Q. Deng, Int. J. Hydrogen Energy, 2010, 35, 8122–8129 CrossRef CAS.
- R. Chen, X. Wang, L. Xu, L. Chen, S. Li and C. Chen, Mater. Chem. Phys., 2010, 124, 83–87 CrossRef CAS.
- A. W. Vittetoe, M. U. Niemann, S. S. Srinivasan, K. McGrath, A. Kumar, D. Y. Goswami, E. K. Stefanakos and S. Thomas, Int. J. Hydrogen Energy, 2009, 34, 2333–2339 CrossRef CAS.
- R. A. Varin, T. Czujko, C. Chiu, R. Pulz and Z. S. Wronski, J. Alloys Compd., 2009, 483, 252–255 CrossRef CAS.
- M. Ismail, Y. Zhao, X. B. Yu, J. F. Mao and S. X. Dou, Int. J. Hydrogen Energy, 2011, 36, 9045–9050 CrossRef CAS.
- J. J. Vajo, F. Mertens, C. C. Ahn, R. C. Bowman and B. Fultz, J. Phys. Chem. B, 2004, 108, 13977–13983 CrossRef CAS.
- M. Ismail, Y. Zhao, X. B. Yu and S. X. Dou, Int. J. Hydrogen Energy, 2010, 35, 2361–2367 CrossRef CAS.
- M. Ismail, Y. Zhao, X. B. Yu, A. Ranjbar and S. X. Dou, Int. J. Hydrogen Energy, 2011, 36, 3593–3599 CrossRef CAS.
- M. Ismail, Y. Zhao, X. B. Yu, I. P. Nevirkovets and S. X. Dou, Int. J. Hydrogen Energy, 2011, 36, 8327–8334 CrossRef CAS.
- M. McCarty, J. N. Maycock and V. R. P. Verneker, J. Phys. Chem., 1968, 72, 4009–4014 CrossRef CAS.
- S.-S. Liu, L.-X. Sun, Y. Zhang, F. Xu, J. Zhang, H.-L. Chu, M.-Q. Fan, T. Zhang, X.-Y. Song and J. P. Grolier, Int. J. Hydrogen Energy, 2009, 34, 8079–8085 CrossRef CAS.
- H. E. Kissinger, Anal. Chem., 1957, 29, 1702–1706 CrossRef CAS.
- M. Resan, M. D. Hampton, J. K. Lomness and D. K. Slattery, Int. J. Hydrogen Energy, 2005, 30, 1417–1421 CrossRef CAS.
- V. P. Balema, J. W. Wiench, K. W. Dennis, M. Pruski and V. K. Pecharsky, J. Alloys Compd., 2001, 329, 108–114 CrossRef CAS.
- L.-C. Yin, P. Wang, X.-D. Kang, C.-H. Sun and H.-M. Cheng, Phys. Chem. Chem. Phys., 2007, 9, 1499–1502 RSC.
- N. Hanada, T. Ichikawa, S. Isobe, T. Nakagawa, K. Tokoyoda, T. Honma, H. Fujii and Y. Kojima, J. Phys. Chem. C, 2009, 113, 13450–13455 CAS.
- L. P. Ma, X. D. Kang, H. B. Dai, Y. Liang, Z. Z. Fang, P. J. Wang, P. Wang and H. M. Cheng, Acta Mater., 2009, 57, 2250–2258 CrossRef CAS.
- L. Xie, Y. Liu, Y. T. Wang, J. Zheng and X. G. Li, Acta Mater., 2007, 55, 4585–4591 CrossRef CAS.
|
This journal is © The Royal Society of Chemistry 2011 |